have been retained. The official nomenclature for structures of the kidney has been established by the Renal Commission of the International Union of Physiological Sciences (12). This terminology is used in this chapter (Table 1.1).
is a normal anatomic variant without functional consequences (22,23,24). In some of the kidneys, three subtle zones are created by two shallow superficial grooves that radiate from the hilum to the lateral border. The three regions correspond roughly to the upper pole, middle zone, and lower pole and usually reflect regions drained by the segmental veins.
ducts of Bellini, creating the potential for intrarenal reflux during urinary tract obstruction and infection (30,31).
TABLE 1.1 Nomenclature of the kidney | ||||||||||||||||||||||||||||||||||||||||||||||||||||||||||||||||||||||||||||||||||||||||||||||||||||||||||||||||||||||||||||||||||||||||||||||||||||||||||||||||||||||||||||||||||||||||||||||||||||||||||||||||||||||||||||||||||||||||||||||||||||||||||||||||||||||||||||||||||||||||||||||||||||||||||||||||||||||||||||||||||||||||||||||||||||||||||||||||
---|---|---|---|---|---|---|---|---|---|---|---|---|---|---|---|---|---|---|---|---|---|---|---|---|---|---|---|---|---|---|---|---|---|---|---|---|---|---|---|---|---|---|---|---|---|---|---|---|---|---|---|---|---|---|---|---|---|---|---|---|---|---|---|---|---|---|---|---|---|---|---|---|---|---|---|---|---|---|---|---|---|---|---|---|---|---|---|---|---|---|---|---|---|---|---|---|---|---|---|---|---|---|---|---|---|---|---|---|---|---|---|---|---|---|---|---|---|---|---|---|---|---|---|---|---|---|---|---|---|---|---|---|---|---|---|---|---|---|---|---|---|---|---|---|---|---|---|---|---|---|---|---|---|---|---|---|---|---|---|---|---|---|---|---|---|---|---|---|---|---|---|---|---|---|---|---|---|---|---|---|---|---|---|---|---|---|---|---|---|---|---|---|---|---|---|---|---|---|---|---|---|---|---|---|---|---|---|---|---|---|---|---|---|---|---|---|---|---|---|---|---|---|---|---|---|---|---|---|---|---|---|---|---|---|---|---|---|---|---|---|---|---|---|---|---|---|---|---|---|---|---|---|---|---|---|---|---|---|---|---|---|---|---|---|---|---|---|---|---|---|---|---|---|---|---|---|---|---|---|---|---|---|---|---|---|---|---|---|---|---|---|---|---|---|---|---|---|---|---|---|---|---|---|---|---|---|---|---|---|---|---|---|---|---|---|---|---|---|---|---|---|---|---|---|---|---|---|---|---|---|---|---|---|---|---|---|---|---|---|---|---|---|---|---|---|---|---|---|---|---|---|---|
|
collecting ducts (CDs). The papilla protrudes into a minor calyx. Its tip, the area cribrosa (with cribriform appearance grossly), has from 20 to 70 openings of the papillary CDs (Bellini ducts); the large variation reflects simple versus compound pyramid arrangement (24,26,28).
![]() FIGURE 1.4 Composite adult and newborn human kidneys. Notice the smooth subcapsular surface of the adult kidney and the prominent lobations of the newborn kidney. |
either anterior or posterior branches or arising separately from the aorta as an aberrant, accessory, or polar artery (Figs. 1.13 and 1.14), which occurs in 25% of the kidneys (32). Another variation is supply of the upper or lower pole by the posterior segmental artery or a branch from the posterior segmental artery.
afferent arteriole (Figs. 1.15 and 1.16). The efferent arterioles, on exiting the glomeruli, form a portal system of capillaries that supplies the adjacent cortical tubules, or provides the main arteriolar flow to the renal medulla in the case of juxtamedullary glomeruli (Figs. 1.2B and 1.17; see Cortical Microvascularization section below). A few interlobular arteries reach the renal capsule and anastomose with branches of the suprarenal and gonadal arteries.
![]() FIGURE 1.10 A simple human renal pyramid with a convex papilla (P) nestled within a minor calyx. S, sinus; OM, outer medulla; C, cortex; Ca, calyx. |
![]() FIGURE 1.16 Normal human kidney. The interlobular artery (A) gives rise to two arterioles; each supplies one glomerulus. (PAS, ×200.) |
from juxtamedullary glomeruli. Their efferent arterioles course directly into the medulla, forming the descending vasa recta (Fig. 1.17). The second blood supply originates from an interlobar artery as it courses along a minor calyx. Several spiral artery branches enter the papilla at the calyceal fornices, sending arterioles to the papillary tip. These arterioles anastomose freely with arterioles from the opposite side, forming a plexus around the distal ducts of Bellini.
![]() FIGURE 1.19 Human kidneys with aorta (A) and vena cava (V). The left main renal vein (arrow) is much longer than the right main renal vein. |
a loose vascularized connective tissue layer, the lamina propria, with an underlying thin muscularis propria.
nephrons are short and quickly transition into a peritubular capillary plexus that forms a uniformly distributed anastomosing vascular lattice amid the cortical tubules of the labyrinth. In the medullary rays the capillary plexus assumes a more longitudinal orientation, following the course of the straight tubules.
monkeys, the vascular bundles are of the simple type, and the tubulovascular relationships of the outer stripe are maintained in the inner stripe. The descending thin limbs (DTLs) and ascending thick limbs of the long-looped juxtamedullary nephrons are situated close to the vascular bundle, whereas the DTLs and ascending thick limbs of the short-looped nephron and the CDs are more peripheral (Fig. 1.33). In other species, such as rat, mouse, and desert rodents, the vascular bundles are of the so-called complex type. In this type, DTLs of shortlooped nephrons leave the interbundle region and curve toward the vascular bundles, intermingling with ascending vasa recta. The thick limbs of both short- and long-looped nephrons and the thin limbs of long-looped nephrons remain in the interbundle region with CDs. These histotopographic differences in tubulovascular relationships generally correlate with urinary concentration ability. However, exceptions do occur such as in the hamster with its simple vascular bundles and high concentration capacity.
TABLE 1.2 Tubular segments within each zone of the medulla | ||||||||||||||||||||||||
---|---|---|---|---|---|---|---|---|---|---|---|---|---|---|---|---|---|---|---|---|---|---|---|---|
|
which is sparse in true capillaries. They also travel outward from the bundle as they enter the outer stripe. The ascending venules finally empty into interlobular or arcuate veins. The venae rectae and the arteriolae rectae are arranged in close proximity throughout their course as they travel down to the papillary tip and back up. The intermingled venous and arterial limbs compose the countercurrent exchange system that maintains the medullary osmotic gradient.
The tubular portion of the nephron has complex spatial and topographic relationships with its microvasculature and demonstrates sequential variation in its cellular constitution tightly linked to function.
the other two, but there is no distinct boundary between these three regions.
this is related to the needs for urinary concentration and water conservation. However, urinary concentration correlates more with the complexity of medullary development rather than simply the number of long-looped nephrons (62,63,64,65,66). In the human kidney, approximately 15% of nephrons are long looped. In the rodent kidney, this is almost doubled to 28%, whereas in cats and dogs, all nephrons are long looped (72).
There are afferent, more peripherally arrayed glomerular capillary domains and efferent, more centrally located domains, with the former constituting most of the capillaries (73,74,75,76,77). There are multiple anastomoses between capillaries within a lobule, and between capillaries in separate lobules, creating a capillary network (Fig. 1.41).
of the BC. Parietal cells abruptly transition into columnar proximal tubular cells at the urinary pole (see Figs. 1.38 and 1.39).
viewing an H&E-stained section under fluorescence (Fig. 1.44). This is useful in kidney biopsies for correlation with immunofluorescence findings (81).
![]() FIGURE 1.43 Normal human glomerulus. The thin delicate detail of the capillary loops and scant mesangium is nicely delineated by the Jones methenamine silver stain. (×650.) |
![]() FIGURE 1.44 Normal human cortex. This H&E-stained section is viewed under fluorescent microscopy. The general architectural features of the glomerulus and tubules are easily recognized. (H&E, ×250.) |
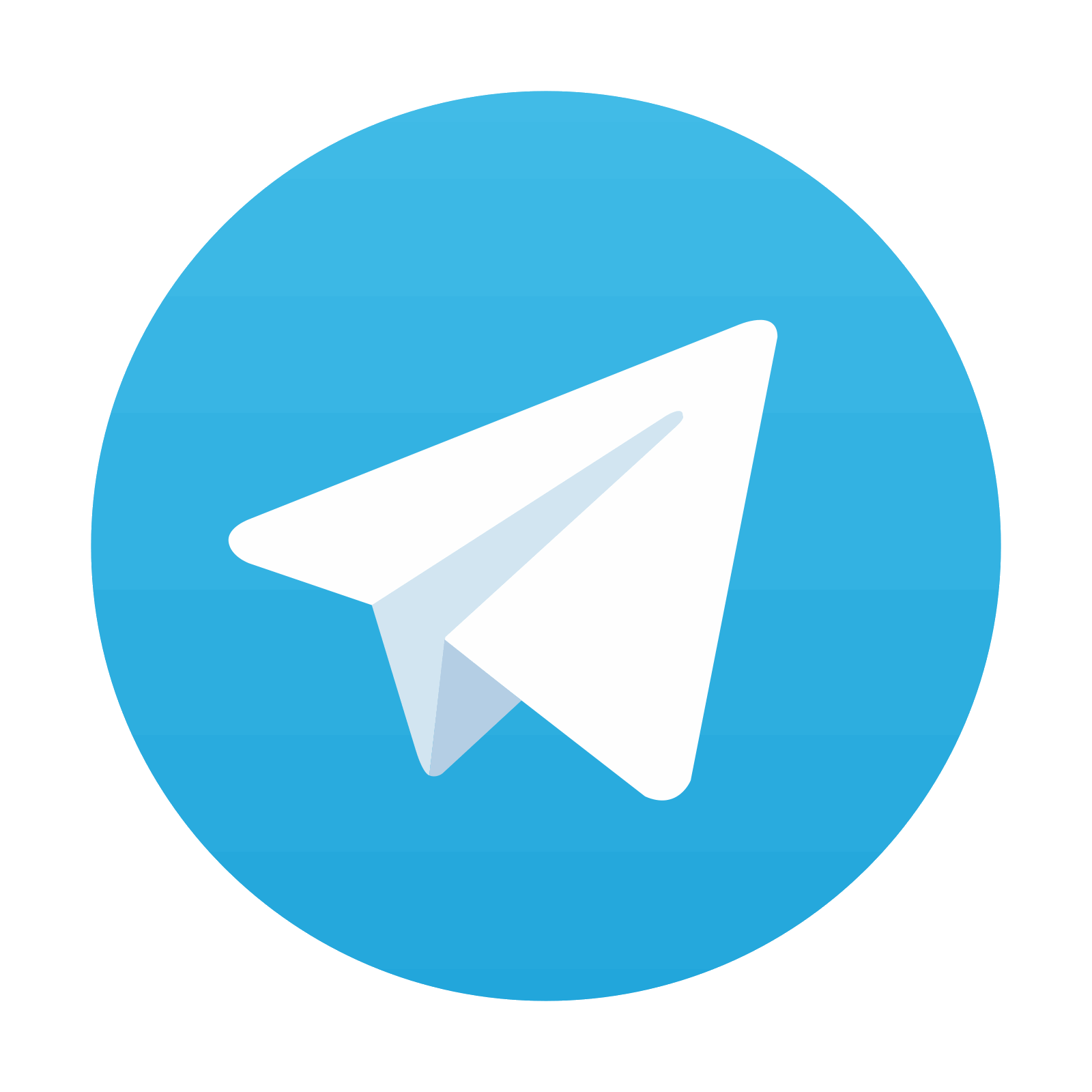
Stay updated, free articles. Join our Telegram channel
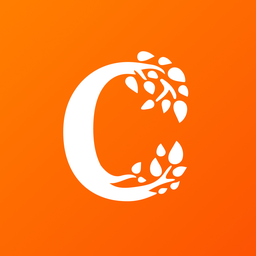
Full access? Get Clinical Tree
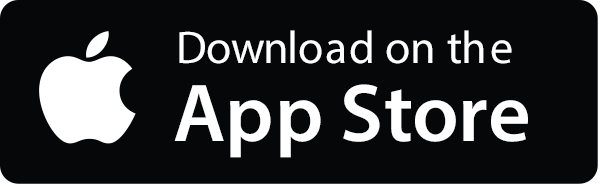
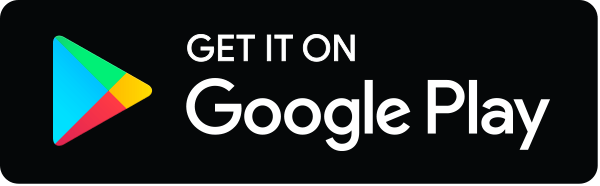
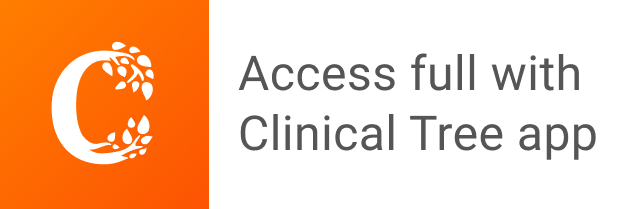