Transmembrane transport of HCO − 3 and CO 2 is central to body pH and volume homeostasis. The Na + -independent, electroneutral CI − /HCO − 3 exchangers AE1/Band 3 and the related AE2 and AE3 polypeptides of the SLC4 gene family play central roles in these processes. The SLC4/AE anion exchangers regulate intracellular pH and, through their polarized localization in renal and other epithelial cells, regulate compartmental and systemic pH. SLC4/AE anion exchangers also regulate cell volume and modulate the cellular equilibrium potential for Cl − , thus contributing to determination of driving forces for multiple ion transport pathways throughout the body. Distinct mutations of human AE1 cause distal renal tubular acidosis, spherocytic hemolytic anemia, and cation-leak stomatocytosis. Lack of AE2 in the mouse causes early death with osteopetrosis and wide impairment of epithelial secretion. Lack of AE3 in the mouse causes late-onset retinal degeneration and increases susceptibility to seizures and heart failure. These phenotypes illustrate the consequences of disrupting local and/or systemic bicarbonate/chloride homeostasis.
Key Words
chloride bicarbonate exchangers, anion exchangers, band 3, AE1, AE2, AE3, erythrocyte, intercalated cell, distal renal tubular acidosis, hereditary spherocytosis.
Introduction
Optimal function of mammalian cells requires tight control of the acid–base status of intracellular and extracellular compartments. The pH of arterial blood is approximately 7.35–7.45, whereas intracellular pH is normally 7.1–7.3. The CO 2 /bicarbonate <SPAN role=presentation tabIndex=0 id=MathJax-Element-1-Frame class=MathJax style="POSITION: relative" data-mathml='(HCO3−)’>(HCO−3)(HCO−3)
(HCO3−)
(HCO−3)
( HCO 3 − )
buffer pair is the body’s major pH buffer system for maintenance of pH homeostasis. CO 2 and <SPAN role=presentation tabIndex=0 id=MathJax-Element-2-Frame class=MathJax style="POSITION: relative" data-mathml='HCO3−’>HCO−3HCO−3
HCO3−
HCO−3
HCO 3 −
also serve as substrates and products in numerous metabolic pathways. Up to fifteen carbonic anhydrase gene products accelerate the slow aqueous equilibration of CO 2 and <SPAN role=presentation tabIndex=0 id=MathJax-Element-3-Frame class=MathJax style="POSITION: relative" data-mathml='HCO3−’>HCO−3HCO−3
HCO3−
HCO−3
HCO 3 −
inside and outside cells by up to five orders of magnitude. Rapid plasmalemmal <SPAN role=presentation tabIndex=0 id=MathJax-Element-4-Frame class=MathJax style="POSITION: relative" data-mathml='HCO3−’>HCO−3HCO−3
HCO3−
HCO−3
HCO 3 −
transport across cell plasma membranes by Na + -dependent and Na + -independent <SPAN role=presentation tabIndex=0 id=MathJax-Element-5-Frame class=MathJax style="POSITION: relative" data-mathml='HCO3−’>HCO−3HCO−3
HCO3−
HCO−3
HCO 3 −
transporters is critical for intracellular and extracellular carbonic anhydrases to maintain this equilibrium in the face of changing metabolic demands. At least two gene superfamilies encode Na + -independent <SPAN role=presentation tabIndex=0 id=MathJax-Element-6-Frame class=MathJax style="POSITION: relative" data-mathml='Cl−/HCO3−’>Cl−/HCO−3Cl−/HCO−3
Cl−/HCO3−
Cl−/HCO−3
Cl − / HCO 3 −
exchange polypeptides: the SLC4 “bicarbonate transporter superfamily” and the SLC26 “sulfate permease” multi-anion transporter superfamily. Under normal physiological conditions, the <SPAN role=presentation tabIndex=0 id=MathJax-Element-7-Frame class=MathJax style="POSITION: relative" data-mathml='Cl−/HCO3−’>Cl−/HCO−3Cl−/HCO−3
Cl−/HCO3−
Cl−/HCO−3
Cl − / HCO 3 −
exchangers among the polypeptides encoded by both gene superfamilies are bicarbonate extruders (acid loaders) and Cl − loaders. Thus, both sets of <SPAN role=presentation tabIndex=0 id=MathJax-Element-8-Frame class=MathJax style="POSITION: relative" data-mathml='Cl−/HCO3−’>Cl−/HCO−3Cl−/HCO−3
Cl−/HCO3−
Cl−/HCO−3
Cl − / HCO 3 −
exchangers contribute to regulation of intracellular pH (pH i ), cell volume, tonicity, and intracellular [Cl − ].
This chapter will focus on the Na + -independent <SPAN role=presentation tabIndex=0 id=MathJax-Element-9-Frame class=MathJax style="POSITION: relative" data-mathml='Cl−/HCO3−’>Cl−/HCO−3Cl−/HCO−3
Cl−/HCO3−
Cl−/HCO−3
Cl − / HCO 3 −
exchangers of the SLC4 gene family: AE1/SLC4A1, AE2/SLC4A2, and AE3/SLC4A3. Other members of the SLC4 gene family will also be briefly introduced. The SLC4 and the SLC26 gene families. have been reviewed previously.
The AE Anion Exchangers Among the SLC4 and SLC26 Superfamilies.
The SLC4 bicarbonate transporter superfamily ( Fig. 54.1 ) includes autosomal genes encoding at least three electroneutral Na + -independent anion exchangers (SLC4A1–3/AE1–3), three Na + -bicarbonate cotransporters (the electrogenic SLC4A4/NBCe1 and SLC4A5/NBCe2 and the electroneutral SLC4A7/NBCn1), and Na + -dependent anion exchangers (SLC4A8/NBCDE and SLC4A10/NCBE). The transport mechanisms of three SLC4 proteins remain controversial. SLC4A10, originally described as a Na + -dependent <SPAN role=presentation tabIndex=0 id=MathJax-Element-10-Frame class=MathJax style="POSITION: relative" data-mathml='Cl−/HCO3−’>Cl−/HCO−3Cl−/HCO−3
Cl−/HCO3−
Cl−/HCO−3
Cl − / HCO 3 −
exchanger has also been described as an electroneutral Na + -bicarbonate cotransporter NBCn2. SLC4A9 was originally described as a Na + -independent <SPAN role=presentation tabIndex=0 id=MathJax-Element-11-Frame class=MathJax style="POSITION: relative" data-mathml='Cl−/HCO3−’>Cl−/HCO−3Cl−/HCO−3
Cl−/HCO3−
Cl−/HCO−3
Cl − / HCO 3 −
exchanger, despite the strong phylogenetic prediction of Na + -dependent transport. The most divergent mammalian member of the family, SLC4A11/BTR1/NaBC1 was first reported as an electrogenic Na + -borate cotransporter important for cell proliferation, but the result has remained unconfirmed despite SLC4A11’s phylogenetic proximity to the SLC4 homologs AtBor1 of A. thaliana and YNL275w/Bor1 of S. cerevisiae , both responsible for maintenance of nontoxic cellular borate levels in the face of elevated environmental borate. SLC4 genes are represented in all metazoans, including the fruitfly D. melanogaster , the mosquito A. aedes , the roundworm C. elegans , the zebrafish D. rerio and the pufferfish T. nigroviridis , as well as in unicellular eukaryotes. An SLC4-related gene was also recently reported in a marine prokaryotic genome.

Cl−/HCO3−
Cl−/HCO−3
Cl − / HCO 3 −
exchangers; Na + -dependent bicarbonate transporters (blue), including electrogenic and electroneutral <SPAN role=presentation tabIndex=0 id=MathJax-Element-13-Frame class=MathJax style="POSITION: relative" data-mathml='Na+/HCO3−’>Na+/HCO−3Na+/HCO−3
Na+/HCO3−
Na+/HCO−3
Na + / HCO 3 −
cotransporters and Na + -dependent <SPAN role=presentation tabIndex=0 id=MathJax-Element-14-Frame class=MathJax style="POSITION: relative" data-mathml='Cl−/HCO3−’>Cl−/HCO−3Cl−/HCO−3
Cl−/HCO3−
Cl−/HCO−3
Cl − / HCO 3 −
exchangers; and borate transporters (green). BTR/SLC4A11 is an electrogenic Na + -borate cotransporter. The borate transporter clade includes proteins from S. cerevisiae (yeast) and A. thaliana (plant) of yet undefined transport mechanism.
The most extensively studied member of the SLC4 gene family is SLC4A1/AE1 (also known as “band 3”), a <SPAN role=presentation tabIndex=0 id=MathJax-Element-15-Frame class=MathJax style="POSITION: relative" data-mathml='Cl−/HCO3−’>Cl−/HCO−3Cl−/HCO−3
Cl−/HCO3−
Cl−/HCO−3
Cl − / HCO 3 −
exchanger abundant in erythrocytes of vertebrates and elasmobranchs, but apparently absent from erythrocytes of the jawless fishes. AE1 is also expressed in the Type A intercalated cells of kidney collecting duct in mammals and birds, and at much lower levels in heart and distal colon. Mutations in the SLC4A1/AE1 gene ( Table 54.3 ) are associated with dominant hereditary spherocytic anemia, dominant Southeast Asian ovalocytosis and stomatocytic cation leak syndromes, and dominant and recessive forms of distal renal tubular acidosis. AE2 is expressed widely among epithelial and other cell types, with highest expression in subsets of epithelial cells including gastric parietal cells, choroid plexus, proximal colonic enterocytes, and thick ascending limb cells. AE3 is expressed at highest levels in brain, heart, and retina, and at lower levels in smooth muscle and epithelial cells. Mutations in the genes encoding SLC4A2/AE2 and SLC4A3/AE3 have not been directly linked to Mendelian human disease, but mutations in the SLC4A4/NBCe1 electrogenic Na + -bicarbonate cotransporter gene cause the recessive syndrome of proximal renal tubular acidosis, ocular abnormalities, and elevated pancreatic amylase, sometimes accompanied by migraine. Mutations in the SLC4A11 (nominal Na + -borate cotransporter) gene are associated with congenital hereditary endothelial dystrophy of the cornea type 2 associated with vision loss, and with Harboyan syndrome (with added hearing loss). Mouse Slc4 knockout disease models for Na + -dependent <SPAN role=presentation tabIndex=0 id=MathJax-Element-16-Frame class=MathJax style="POSITION: relative" data-mathml='HCO3−’>HCO−3HCO−3
HCO3−
HCO−3
HCO 3 −
transporters for which corresponding human genetic diseases remain unidentified are slc4a5 -/- and slc4a10 -/- mice exhibiting altered choroid plexus function with decreased cerebral ventricular volume; slc4a7 -/- mice with retinal and cochlear degeneration and mild hypertension resistant to exacerbation by angiotensin II; slc4a8 -/- mice with coincident absence of Slc26a4/pendrin lack electroneural NaCl reabsorption by cortical collecting duct Type B intercalated cells; and slc4a11 -/- mice that exhibit, in addition to corneal and auditory abnormalities shared with the corresponding human diseases, polyuria likely related to renal medullary thin limb dysfunction in the countercurrent multiplier mechanism.
<SPAN role=presentation tabIndex=0 id=MathJax-Element-17-Frame class=MathJax style="POSITION: relative" data-mathml='Cl−/HCO3−’>Cl−/HCO−3Cl−/HCO−3
Cl−/HCO3−
Cl−/HCO−3
Cl − / HCO 3 −
exchange and other anion exchange functions are also mediated by SLC26 polypeptides. The SLC26 gene family was identified first among Neurospora sulfate transporters, and subsequently in vertebrates and throughout the evolutionary tree, including prokaryotes. Polypeptide products of the 11 SLC26 genes transport a wide range of monovalent and divalent anions including sulfate <SPAN role=presentation tabIndex=0 id=MathJax-Element-18-Frame class=MathJax style="POSITION: relative" data-mathml='(SO42−)’>(SO2−4)(SO2−4)
(SO42−)
(SO2−4)
( SO 4 2 − )
, chloride (Cl − ), bicarbonate <SPAN role=presentation tabIndex=0 id=MathJax-Element-19-Frame class=MathJax style="POSITION: relative" data-mathml='(HCO3−)’>(HCO−3)(HCO−3)
(HCO3−)
(HCO−3)
( HCO 3 − )
, hydroxyl anion (OH − ), oxalate, and formate. Although unrelated in sequence to the SLC4 gene family, at least three of the SLC26 anion exchangers can mediate <SPAN role=presentation tabIndex=0 id=MathJax-Element-20-Frame class=MathJax style="POSITION: relative" data-mathml='Cl−/HCO3−’>Cl−/HCO−3Cl−/HCO−3
Cl−/HCO3−
Cl−/HCO−3
Cl − / HCO 3 −
exchange: the congenital chloride-losing diarrhea gene SLC26A3/DRA, the Pendred syndrome gene SLC26A4/Pendrin, and SLC26A6/Pat-1/CFEX. SLC26A7, SLC26A9, and SLC26A11 also mediate <SPAN role=presentation tabIndex=0 id=MathJax-Element-21-Frame class=MathJax style="POSITION: relative" data-mathml='Cl−/HCO3−’>Cl−/HCO−3Cl−/HCO−3
Cl−/HCO3−
Cl−/HCO−3
Cl − / HCO 3 −
exchange, but SLC26A7 and SLC26A9 also appear to function as anion channels. SLC26A2 mediates sulfate/Cl − exchange in chondrocytes, hepatocytes and enterocytes. SLC26A6 and SLC26A1 mediate enterocyte and proximal tubule oxalate/Cl − exchange.
AE Domain Structure and Alternative Transcripts
The AE polypeptide products of the SLC4A1–3 and SLC4A9 genes share a tripartite domain structure as predicted by hydropathy analysis and confirmed by chemical and antibody reactivity studies ( Fig. 54.2 ). A long N-terminal cytoplasmic domain extends for 400–700 amino acids (aa) before the polypeptide chain enters the lipid bilayer. Overall % aa identity of this region among the AE polypeptides is only ~35%, but short stretches within the region are highly conserved ( Fig. 54.3 ). The N-terminal domain of AE1 binds to cytoskeletal proteins, but binding partners of the N-terminal domains of AE2 and AE3 remain less well investigated. The transmembrane domains of ~500 aa in length ( Fig. 54.2 ) are modeled to traverse the bilayer approximately 12–14 times, with at least one large extracellular loop carrying 1,2, or 3 N-glycans. The AE transmembrane domains share a higher overall aa identity of~65%, with yet greater sequence identity among the putative transmembrane spans ( Fig. 54.3 ). When expressed in Xenopus oocytes and in HEK 293 human embryonic kidney cells, the transmembrane domain of AE1 mediates anion transport in the absence of all but the membrane-proximal~30 aa of the N-terminal cytoplasmic domain. The transmembrane domain is followed by a short C-terminal cytoplasmic tail of ~35–40 aa, and harbors a putative binding site for carbonic anhydrase II (but see ).

Cl−/HCO3−
Cl−/HCO−3
Cl − / HCO 3 −
exchange function, despite their inclusion in the Na + -dependent clade ( Fig. 54.1 ). Blue indicates predicted transmembrane domains. Total polypeptide lengths (aa) at right. Length (aa) of variant N-terminal domains is indicated within leftmost boxes, and of variant C-terminal domains within rightmost boxes (for the AE3–14nt variants). Aa# of junctions indicated in middle boxes. rb, rabbit.

Cl−/HCO3−
Cl−/HCO−3
Cl − / HCO 3 −
exchanger polypeptides AE1/SLC4A1 (eAE1, NP_000333), AE2/SLC4A2 (AE2a, NP_003031), and AE3/SLC4A3 (bAE3, NP_005061). Alignment was performed with DNAStar software, with manual revision. Conserved residues are highlighted in gray. Putative transmembrane spans are overlined, guided by models of and.
The majority of AE polypeptide variants described to date vary in their N-terminal amino acid sequences ( Fig. 54.2 ). This variation derives from alternative promoter usage resulting in distinct 5′ mRNA exons expressed in tissue-specific and cell type-specific patterns. The AE1 and AE3 genes encode two such 5′-variant transcripts encoding proteins with distinct N-terminal aa sequences. The AE2 genes of rat and human express four 5′-variant transcripts, and the mouse gene expresses five such transcripts. Two additional transcripts of the AE3 gene encode alternate 3′-transcripts encoding predicted soluble proteins devoid of intrinsic anion transport function. The AE4 gene encodes at least four ( Fig. 54.2 ) and possibly many more variant transcripts. Unusual among the AE genes in its greater sequence similarity with Na + -dependent than with Na + -independent SLC4 polypeptides ( Fig. 54.1 ), AE4 may nonetheless function as a Na + -independent anion exchanger (but see ).
The AE anion exchangers mediate electroneutral, bidirectional exchange of monovalent anions, capable of hetero-exchange and homo-exchange ( Fig. 54.4A ). They can also mediate cotransport of divalent anions (sulfate and oxalate are the best studied) with a proton in homoexchange or in heteroexchange for monovalent chloride ( Fig. 54.4B ). Exchange is believed to be via a “ping-pong” mechanism, also known as a “sequential” or an “alternating sites” mechanism ( Fig. 54.4C ), on the basis of biochemical, kinetic, and spectroscopic changes measured during studies of anion substitution and ligand inhibition. Extensive functional investigation of AE1 in intact red cells has been accompanied by study of recombinant AE polypeptides in the major expression systems of Xenopus oocytes and HEK 293 cells, as well as in CHO, K562, MDCK, and Sf9 insect cells, S. cerevisia , and E. coli .

HCO3−
HCO−3
HCO 3 −
, then inhibited by extracellular DIDS. D. Reversible <SPAN role=presentation tabIndex=0 id=MathJax-Element-25-Frame class=MathJax style="POSITION: relative" data-mathml='Cl−/HCO3−’>Cl−/HCO−3Cl−/HCO−3
Cl−/HCO3−
Cl−/HCO−3
Cl − / HCO 3 −
exchange by BCECF-AM-loaded osteoclasts from wildtype ( +/+ ), heterozygous ( +/- ) and homozygous ( -/- ) Ae2(a,b) knockout mice (from with permission). E. The ping-pong (sequential) exchange mechanism is believed applicable to the transport modes portrayed in A and B (C modified from with permission).
Localization and Function of Anion Exchangers in Tissues
AE1 is essential for postnatal survival in humans zebrafish, mice and cattle. AE1 is expressed at highest levels in erythrocytes as the eAE1 polypeptide, constituting 25% of total membrane protein and 50% of intrinsic membrane protein. This high density in the red cell membrane is needed for rapid <SPAN role=presentation tabIndex=0 id=MathJax-Element-26-Frame class=MathJax style="POSITION: relative" data-mathml='HCO3−’>HCO−3HCO−3
HCO3−
HCO−3
HCO 3 −
transport during the sub-second traversal of pulmonary capillaries (and most other non-sinusoidal capillary beds). Sparingly soluble CO 2 generated by cellular metabolism diffuses across capillary endothelial cells into red cells. There it is converted by erythroid cytoplasmic carbonic anhydrases into highly soluble <SPAN role=presentation tabIndex=0 id=MathJax-Element-27-Frame class=MathJax style="POSITION: relative" data-mathml='HCO3−’>HCO−3HCO−3
HCO3−
HCO−3
HCO 3 −
and promptly exported by eAE1 in exchange for extracellular Cl − . The H + liberated by the OH − -consuming carbonic anhydrase reaction is buffered by hemoglobin to enhance oxygen release to hypoxic tissues.
In the pulmonary capillaries this process operates in reverse, with entry of extracellular <SPAN role=presentation tabIndex=0 id=MathJax-Element-28-Frame class=MathJax style="POSITION: relative" data-mathml='HCO3−’>HCO−3HCO−3
HCO3−
HCO−3
HCO 3 −
into the red cell in exchange for cytoplasmic Cl − . The newly transported intracellular <SPAN role=presentation tabIndex=0 id=MathJax-Element-29-Frame class=MathJax style="POSITION: relative" data-mathml='HCO3−’>HCO−3HCO−3
HCO3−
HCO−3
HCO 3 −
is used by red cell carbonic anhydrase to generate CO 2 , which diffuses down its concentration gradient into the alveolar lumen for exhalation. At the same time, hemoglobin’s surrender of H + to the OH − newly generated by carbonic anhydrase enhances hemoglobin binding of O 2 diffusing into the red cell from the alveolus. This reversible <SPAN role=presentation tabIndex=0 id=MathJax-Element-30-Frame class=MathJax style="POSITION: relative" data-mathml='Cl−/HCO3−’>Cl−/HCO−3Cl−/HCO−3
Cl−/HCO3−
Cl−/HCO−3
Cl − / HCO 3 −
exchange cycle of the red cell, shuttling between hypoxic, hypercarbic peripheral capillaries and hyperoxic, hypocarbic pulmonary capillaries, increases by five- to eight-fold the total CO 2 carrying capacity of the blood (~90% <SPAN role=presentation tabIndex=0 id=MathJax-Element-31-Frame class=MathJax style="POSITION: relative" data-mathml='HCO3−’>HCO−3HCO−3
HCO3−
HCO−3
HCO 3 −
, 5% free CO 2 , and 5% carbamino-hemoglobin and other carbamino-CO 2 ).
The role of oxygen tension in controlling the rate of mammalian eAE1-mediated anion exchange remains controversial, with reports of both hypoxic stimulation and hypoxic inhibition. eAE1 also mediates H + -cotransport of sulfate and oxalate in exchange for Cl − . Elevated erythroid oxalate transport has been correlated with hyperoxaluria and oxalate nephrolithiasis, but AE1 polymorphisms have not correlated with oxalate lithiasis. eAE1 has been proposed to contribute to erythroid transport of nitric oxide (NO) or its metabolites as part of red-cell facilitated vasodilation, by a nitrite transport-independent mechanism possibly involving AE1 S-nitrosothiolation. However, the stilbene disulfonate-insensitivity of erythroid NO transport, and its undiminished transport by hagfish and lamprey red cells that lack AE1, suggest a dominant AE1-independent NO transport mechanism.
The kidney isoform of AE1 is a truncated form of erythroid AE1, lacking the initial 65 (human) N-terminal amino acids due to transcriptional initiation of kAE1 mRNA from an alternative promoter in exon 3 of the AE gene. kAE1 is expressed in the basolateral membrane of Type A intercalated cells of the mammalian renal collecting duct, where it is essential for normal distal urinary acidification. AE1 is also expressed at lower levels in glomerular podocytes, heart (whether as a tissue-specific transcript variant remains controversial), and in distal colon of rat but not human. Recently, AE1 was localized in the sensory cilia of olfactory neurons.
The AE2/SLC4A2 <SPAN role=presentation tabIndex=0 id=MathJax-Element-32-Frame class=MathJax style="POSITION: relative" data-mathml='Cl−/HCO3−’>Cl−/HCO−3Cl−/HCO−3
Cl−/HCO3−
Cl−/HCO−3
Cl − / HCO 3 −
exchanger is the most widely expressed of the Na + -independent SLC4 anion exchangers, and in the mouse is essential for post-weaning survival. In the basolateral membrane of gastric parietal cells, AE2 is required for normal gastric acid secretion. AE2 is required for normal osteoclast activity, and for normal male fertility. AE2 is also expressed ( Fig. 54.14 ) in conjunctival epithelium, in odontoblasts and ameloblasts in choroid plexus epithelial cells, intestinal enterocytes, respiratory epithelium, and in biliary ductular epithelium. AE2 is expressed along the length of the nephron ( Fig. 54.13 ), at highest levels in basolateral membranes of medullary thick ascending limb and inner medullary collecting duct. AE2 has also been noted in basolateral membrane of macula densa in mouse, rat, and rabbit, where it may possibly contribute to glomerulotubular feedback. The near-ubiquitous distribution of AE2 suggests that AE2 also serves a homeostatic, housekeeping function in the regulation of intracellular pH (pH i ), intracellular chloride concentration and cell volume regulation in renal epithelial cells as well as in other tissues. In some circumstances, as in IMCD cells, AE2 may contribute to transepithelial anion secretion.
AE3 expression is prominent in heart, brain, retina, adrenal gland, as well as at lower levels in smooth muscle and in epithelial cells of kidney and gut. AE3 can modulate seizure threshold in mice, but its role in renal and cardiac function remains little understood, slowed in part by questionable histochemical specificity of some antibodies as judged by absence of immunostaining in knockout tissue (unpublished results). AE4/SLC4A9 appears apical in gastric surface mucous and duodenal villus cells, but shows species-dependent cell polarity and cell type-specificity in immunostained intercalated cells. The antibodies may prove unreliable or species-specific, since the AE4 promoter exhibits Type B-intercalated cell specificity. The physiological function of AE4 remains unclear.
AE1 N-Terminal Cytoplasmic Domain Structure and Binding Proteins
Much structural information available for AE anion exchangers derives from study of the abundant erythroid AE1 (eAE1) polypeptide. The N-terminal cytoplasmic domain is attached to and may anchor cytoskeletal protein complexes at the inner face of the erythroid plasma membrane, as shown in Fig. 54.5 . eAE1 is linked to the spectrin-actin cytoskeleton through its binding of the linker protein ankyrin. Ankryin copy number is ~20% that of eAE1 monomers. eAE1 in the red cell membrane appears to be homodimeric or homotetrameric as bound to ankyrin. eAE1 also binds tightly to protein 4.2 and glycophorin A, and with lower apparent affinity to protein 4.1, an actin-binding component of the cytoskeletal junctional complex ( Fig. 54.5 ). Less direct interactions have been detected with NH 3 channel RhAG and with water/CO 2 channel AQP1, but see. AE1 participates in an erythroid membrane macromolecular complex established early during erythropoiesis.

The X-ray structure of the recombinant dimeric N-terminal cytoplasmic domain of eAE1 overexpressed in E. coli and crystallized at pH 4.8 has been solved at 2.6 A resolution ( Fig. 54.6 ). The eAE1 monomers are intertwined through “domain swapping” of individual dimerization domains via leucine zipper interactions. The monomers show near two-fold symmetry around an axis at the dimer interface, and the dimers are packed in the crystal as tetramers. The N-terminal 54 amino acids (aa) and the membrane-proximate C-terminal-most 19 aa of eAE1 were disordered in the crystal, and so not visualized. The 20–25 aa immediately preceding entry of the polypeptide chain into the lipid bilayer were omitted from the crystallized recombinant polypeptide, guided by earlier protease accessibility studies. The absence of this junctional structure renders uncertain the orientation of the AE1 N-terminal cytoplasmic domain with respect to its adjacent transmembrane domain. This eAE1 crystal structure has been used to model the corresponding region of SLC4A2/AE2, and will provide the template for structural analysis of the recently crystallized recombinant N-terminal cytoplasmic domain of the homologous electrogenic sodium bicarbonate cotransporter SLC4A4/NBCe1A.

The soluble eAE1 N-terminal cytoplasmic domain undergoes several structural changes upon pH shift from 6.0 to 10.0, including elongation of the Stokes radius and the axial ratio, increased intrinsic fluorescence, decreased thermal stability, and increased protein segmental motion. The crystal structure of the eAE1 N-terminal cytoplasmic domain is more compact than predicted by the domain’s solution properties, possibly reflecting the acidic crystallization pH. The crystal structure can be modeled to undergo an alkaline pH-induced elongation in the region of the dimerization domain ( Fig. 54.6 ), leading to separation of the peripheral protein binding domain from the dimerization domain, supported by intradimer luminescence resonance energy transfer (LRET) measurements between Cys201 residues in adjacent monomers. Single particle fluorescence resonance energy transfer (FRET) measurements document two populations of conformers at neutral pH, with inter-Cys201 distances similar to those measured by LRET at extreme pH values. However, this distance is larger when estimated “in situ” in endogenous AE1 present in KI-stripped inside-out red cell vesicles. Moreover, the pH-dependence of that distance differs from that of pure recombinant N-terminal cytoplasmic domain prepared from inside-out vesicles, further suggesting distinct conformations in the two experimental systems, and the potential for considerable plasticity of conformation dependent on local environment and binding partners.
Many functions have been assigned to the N-terminal cytoplasmic domain of eAE1 ( Table 54.1 ). Glycolytic enzymes translocated from cytosol to membrane binding sites where they may form macromolecular complexes to regulate ATP production in concert with metabolic demand. Aldolase binding to AE1 requires two sites within aa 1–23, including aa 6–10 and aa 19–23. Glyceraldehyde-3-phosphate dehydrogenase binds to either of two sites located within aa 1–11 or within 12–23. Phosphofructokinase binds to aa 12–23, but AE1 does not contribute the membrane binding sites for lactate dehydrogenase and pyruvate kinase. Thus, organization of the entire glycolytic complex at the red cell membrane requires not only AE1 but additional membrane proteins. Oxygen tension and hemoglobin binding to the eAE1 N-terminal region also contribute to membrane association of glycolytic enzymes. Glycolytic enzymes are largely membrane-localized in inactive form in oxygenated erythrocytes through binding to N-terminal residues of eAE1. Deoxygenation leads to enzyme release and translocation to the cytosol, thus derepressing erythroid glycolytic flux. Glycolytic enzymes of Ae1 -/- mouse red cells exhibit oxygenation-independent, constitutively cytosolic localization. The eAE1 peptide aa 1–10 bound with low affinity to tetrameric hemoglobin, and decreased hemoglobin cooperativity to the same degree as did the entire N-terminal cytoplasmic domain, or as 2,3-diphosphoglycerate. AE1 N-terminal cytosolic domain mutants with enhanced hemoglobin binding accelerated polymerization of sickle hemoglobin in solution, suggesting a possible point of therapeutic intervention in sickle disease. Conversely, hemoglobin also induced conformational change in AE1.
Location | eAE1 Residue | Importance/Function | Reference |
---|---|---|---|
N-term cytoplasmic | 1–11, 12–23 | GAPDH binding site | |
12–23 | Deoxy-hemoglobin/hemichrome binding site | ||
Y8, Y21 | p72syk phosphorylation sites, Y8 inhibits binding of glycolytic enzymes | ||
6–10, 19–23 | Aldolase binding site | ||
12–23 | Phosphofructokinase binding site | ||
T42 | Major casein kinase 1 phosphorylation site | ||
1–54 | Disordered in crystal | ||
55–65, 175–185 | Required for Ankyrin-1 binding site | ||
Y105, D316 | Postulated to contribute inter-monomeric H bond related to pH-sensitive elongation, Trp fluorescence, thermal stability | ||
C201, C317 | Able to form inter-monomeric disulfide link | ||
202–211, 357–9 | Disordered in crystal | ||
208–225 | Contributes to dimer: dimer interface of tetramer | ||
252–261 | Contributes to dimer: dimer interface of tetramer | ||
S303 | Minor casein kinase 1 phosphorylation site | ||
314–344 | Dimerization arm | ||
343–347 | Required for Protein 4.1 binding site | ||
K360 | Cytoplasmic domain trypsin cleavage site | ||
G381 | Deletion abolishes function but maintains surface expression | ||
357–399 | Flexible link between N-term cyto and transmembrane domains | ||
Y359 | Cytoplasmic domain chymotrypsin cleavage site. | ||
Secondary p53/56lyn phosphorylation site, requires prior syk phosphorylation of Y8/Y21; SHP-2 binding to phospho-Y359 allows dephosphorylation of Y8, Y21, Y904. | |||
Required for basolateral targeting in polarized MDCK cells | |||
C-term transmembrane | K430 | EC1 site of extracellular eosin-maleimide binding, reductive methylation; inhibits function | |
C479 | TM3 site reacts with PCMBS, unreactive with maleimides | ||
K539 | EC3 covalent binding site for DIDS/H 2 -DIDS, SITS and DNFB; inhibits function | ||
K542 | EC3 alternate covalent binding site for DIDS (not H 2 (DIDS) when K542 mutated | ||
K551, K562 | EC3 sites of inter-monomeric crosslinking with BSSS within dimer | ||
M559 | Oxidized by Chloramine T (N-chloro-p-toluenesulfoamide) | ||
K590 | Inner TM6 covalent binding site for phenyl isothiocyanate and DNFB; inhibits function | ||
Q630 | EC4 extracellular papain cleavage site; inhibits function | ||
N642 | EC4 N-glycosylation site; glycan not required for trafficking or function | ||
E681 | Inner TM8 binding site for extracellular Woodward’s Reagent K; inhibits function | ||
Reduction by borohydride leads to H+-independent sulfate self-exchange. | |||
H734 | Intracellular DEPC labeling site, as assigned based on mutagenesis and function in oocytes. | ||
M741 | Oxidized by Chloramine T, partially blocked by DNDS and by DEPC. | ||
K743 | Intracellular trypsin cleavage site (at low ionic strength) | ||
H834 | Intracellular DEPC labeling site; modification decreases sulfate affinity, but not substrate-induced conforational change | ||
C843 | Inner TM13 palmitoylation site | ||
K851 | Outer TM13 PLP binding site and H 2 DIDS intramonomeric crosslinking site; inhibitory | ||
C885 | C-term cyto tail NEM labeling site | ||
886–890 | Carbonic anhydrase 2 binding site; stimulatory | ||
886–911 | Alanine scan mutagenesis reduces V max for sulfate transport in S. cerevisiae. | ||
R901 | C-terminal cyto tail site of hydroxyphenylglyoxal binding, reduced in H 2 -DIDS-locked outward conformation of AE1. Modification or mutations reduces V max for sulfate transport. | ||
D902EY | Involved in binding GAPDH to kAE1 | ||
Y904 | Secondary p53/56lyn phosphorylation site, requires prior syk phosphorylation of Y8/Y21 | ||
Required for basolateral targeting in polarized MDCK cells. | |||
M909 | Oxidized by Chloramine T | ||
878–911 | Binds cytoplasmic N-terminal tail of glycophorin A in vitro and in yeast two hybrid. | ||
893–911 | Encodes or activates red cell ghosts glycophorin A protease. |
The major human AE1 binding site for erythroid ankyrin is a surface loop comprising aa 175–185. The AE1-ankyrin interaction, although critical for membrane stability, was insensitive to shear stress in resealed red cells. Mouse red cells lacking the corresponding critical Ae1 aa 188–199, despite loss of ability to bind ankyrin in vitro , were found to retain 60% of AE1 cytoskeletal binding in red cells that were less destabilized than predicted. The sustained cytoskeletal binding was later attributed in part to interaction with adducin and to the junctional complex. Increased cytoskeletal attachment of AE1 gradually reduces AE1 mobility in the plane of the plasma membrane during erythroid development. Binding of the N-terminal 30 kDa membrane-association domain of protein 4.1R was localized to the LRRRY motif at AE1 aa 343–347 by ligand blot, whereas solution binding methods indicated a predominant role for sites near the far N-terminus of the cytoplasmic domain. ~20% of erythroid protein 4.1 binds to AE1, with larger proportions bound to glycophorin C and to p55/dematin. Phosphatidylinositol-4,5-bisphosphate (PIP2) decreased protein 4.1 binding to AE1 while increasing binding to glycophorin C. Single particle tracking experiments in wildtype and mutant mouse red cells suggest that ~40% of eAe1 is bound to ankyrin, ~33% is bound to adducin, and 27% is unattached to cytoskeletal proteins ( Fig. 54.5 ).
The ribbon diagram of Fig. 54.6C shows as a dark β strand the eAE1 aa 55–65 that are absent from kidney AE1 (kAE1, Fig. 54.2 ) of renal collecting duct Type A intercalated cells. Absence from kAE1 of this β-strand and the preceeding erythroid-specific residues predicts a structure quite different from that of eAE1, consistent with the inability of kAE1 to bind erythroid ankyrin protein 4.1R, and aldolase. The lack of ankyrin binding by kAE1 corresponds to apparent absence of erythroid ankyrin in Type A intercalated cells. The influence of eAE1-specific aa 1–65 (absent from kAE1) on the conformation of the N-terminal cytoplasmic domain is further suggested by the ability of the common, clinically benign polymorphism K56E (band 3 Memphis, Table 54.3 ) to retard the SDS-polyacrylamide gel mobility of the N-terminal 60 kDa chymotryptic fragment of erythroid AE1. An additional influence on the structure of the transmembrane domain is suggested by the decreased erythroid transport rate for the (slowly transported putative) substrate, phosphoenolpyruvate.
The erythroid-specific protein 4.2 closely associated with eAE1 during fractionation, and interacted when coexpressed in Xenopus oocytes. Genetic deficiency of AE1 in human hereditary spherocytosis or in knockout animals can be associated with decreased protein 4.2. Conversely, the hereditary elliptocytosis or spherocytosis of protein 4.2 deficiency is associated with partial reduction in abundance of erythroid AE. Incorporation of protein 4.2 into band 3 proteoliposomes slightly reduced anion transport. In contrast, coexpression of protein 4.2 in Xenopus oocytes increased AE1-mediated anion transport, whereas some protein 4.2 spherocytosis mutants lacked this phenotype. Protein 4.2 may also link AE1 to the Rh complex via red cell CD47.
Undefined AE1 residues bind to aa 200–211 of protein 4.2, immediately adjacent protein 4.2’s ankryin-binding site at aa 187–200. AE1 cleavage at aa 45 and aa 205 by erythroid caspase 3 decreased binding of protein 4.2. Since activated red cell caspase 3 also promotes externalization of phosphatidylserine, a marker and agent of terminal red cell aging and a signal for erythrophagocytosis, the band 3-protein 4.2 interaction may signal erythrocyte removal from the circulation. In HEK-293 cells, AE1 hereditary spherocytosis mutant polypeptides E40K, G130R, and P327R exhibited diminished binding to co-expressed protein 4.2, with decreased protein 4.2. The AE1 transmembrane domain did not interact with protein 4.2.
The tyrosine kinases Syk and Lyn bind to human eAE1 and phosphorylate its N-terminal cytoplasmic domain at Y8, Y21, and Y359. The phosphorylation state of these Tyr residues regulates glycolytic enzyme binding, but likely not anion transport. eAE1 tyrosine phosphorylation is increased by malaria parasite invasion, the oxidative stress of sickle disease, thalassemia, G6PD deficiency, and normal cell aging, as well as by hypertonic cell shrinkage. Tyrosine phosphorylation also promotes AE1 dissociation from the cytoskeleton and promotes mobility in the membrane, perhaps adaptive responses to the above stresses.
A calponin homology domain of human kAE1 (aa 27–189, corresponding to aa 92–254 of eAE1) was shown to bind to the C-terminal catalytic domain of integrin-linked kinase in the endoplasmic reticulum, traffic together to the basolateral membrane leading to increased surface expression with proportionately increased transport activity, although without the expected increase in actin cytoskeletal association. The AE1 N-terminal cytoplasmic domain has also been shown to interact with the C-terminal tail of GLUT1. Although the N-terminal cytoplasmic domain of AE2 may bind erythroid ankyrin, others detected no ankyrin binding to N-terminal cytoplasmic domains of kAE1, AE2, or cAE3. AE2 binding to the distinct Golgi ankyrin Ank 195 may contribute to membrane anchoring of the Golgi cytoskeleton. AE3 N-terminal cytoplasmic domain binding proteins have not been reported.
AE1 C-Terminal Transmembrane Domain Structure and Binding Proteins
The C-terminal transmembrane domains of AE1, AE2, and AE3 suffice to mediate anion exchange in the absence of their corresponding N-terminal cytoplasmic domains. The native or detergent-solubilized transmembrane domains of AE1, AE2, and AE3 are dimers or higher-order oligomers. Figs 54.7A–F shows 18A resolution cryo-electron microscopy images of the dimeric, H 2 -DIDS-crosslinked transmembrane domain of solubilized eAE1 in tubular crystals, supporting earlier electron microscopic images of negatively stained two-dimensional crystals. Higher resolution ( Fig. 54.7A ) electron microscopic images of 2D crystals ( Fig. 54.7G ) revealed outlines of several transmembrane helices, allowing identification of an overall fold with similarities to the Clc Cl − /H + exchanger gene superfamily. This similarity has prompted consideration of an AE1 transmembrane domain model incorporating anti-parallel V-shaped motifs differing from that previously derived from topographically directed mutagenesis and labeling studies (as in Fig. 54.8 ).


Growth of three-dimensional crystals suitable for high resolution X-ray diffraction has proven difficult for the transmembrane domain of eAE1 and for the S. cerevisiae SLC4 putative borate transporter polypeptide YNL275w/Bor1p ( Fig. 54.1 ). This difficulty may reflect in part the conformational flexibility of AE1 required to accommodate a wide range of anion substrate dimensions, and the possibly increased susceptibility of AE1 Met residues to oxidation by peroxides accumulating in polyoxyethylene detergents such as C12E8. Polytopic transmembrane domains of prokaryotic membrane proteins overexpressed in E. coli have proven more amenable to crystallization and have provided insight into SLC26-related anion transporter structure. The recent description of a marine prokaryotic SLC4 anion transporter suggests that this or another route to crystallization and structure determination will soon bear fruit for a prototype SLC4 anion transporter transmembrane domain.
In the absence of a high resolution crystal structure, indirect studies of SLC4 transmembrane domain structure have relied upon chemical modification of native or detergent-solubilized erythroid eAE1, and site-directed mutagenesis studies of recombinant AE1 ( Table 54.1 ) and AE2 ( Table 54.2 ). Fig. 54.8 presents a topographical model of the secondary structure of eAE1, based on hydropathy analysis updated by scanning cysteine accessibility mutagenesis data (but without considering the 2D crystal EM data shown in Fig. 54.7 ). The model is also informed by proteolytic, glycolytic, and chemical labeling studies ( Table 54.1 ), identification of polymorphic blood group antigen sites recognized by extracellular antibodies ( Fig. 54.8 and Table 54.3 ), and scanning N-glycosylation insertional mutagenesis studies. These latter studies suggest that folding of the transmembrane domain in the endoplasmic reticulum (as measured by in vitro translation) differs from that in the plasma membrane, and likely undergoes further maturation during transit to the cell surface. The proposal of two flexible re-entrant loop regions suggested by accessibility of introduced Cys residues to impermeant reagents from either side of the cell membrane deviates considerably from earlier models based solely on hydropathy analysis. A model of AE1 transmembrane helix packing was proposed based on groups of two or more complementary subfragments of the AE1 transmembrane domain capable of partial reconstitution of wildtype chloride transport activity. Intra- and intersubunit distance constraints have been proposed based upon fluorescence resonance energy transfer (FRET) and luminescence resonance energy transfer experiments (LRET) between inhibitor binding sites or between inhibitor probes and targeted cysteine probes. FRET experiments have been extended to single dimeric AE1 subdomains on coverslip, allowing measurement of the kinetics of single molecule conformational changes. Intermolecular FRET has also been used to estimate the local pH i gradient change produced by AE1-mediated <SPAN role=presentation tabIndex=0 id=MathJax-Element-33-Frame class=MathJax style="POSITION: relative" data-mathml='Cl−/HCO3−’>Cl−/HCO−3Cl−/HCO−3
Cl−/HCO3−
Cl−/HCO−3
Cl − / HCO 3 −
exchange in the immediate intracellular vicinity of the AE1 polypeptide expressed in HEK-293 cells.
Location | AE2a Residue | Importance/Function | Reference |
---|---|---|---|
N-term cytoplasmic | 127–134, 145–149 | Deletion or Ala substitution alkaline-shifts pH o (50) | |
150–175 | Progressive deletion acid-shifts pH o (50) | ||
H313/H317 | Ala substitution acid-shifts pH o (50) | ||
E318/E322 | Ala Substitution acid-shifts pH o (50) , reduces pH i sensitivity | ||
F320 | Ala, Tyr substitution acid-shifts pH o (50) , reduces pH i sensitivity | ||
L323 | Ala substitution acid-shifts pH o (50) , reduces pH i sensitivity | ||
W336 | Ala substitution acid-shifts pH o (50) , reduces pH i sensitivity | ||
E338 | Ala substitution reduces pH i sensitivity | ||
R341 | Ala substitution acid-shifts pH o (50) , reduces pH i sensitivity | ||
W342 | Ala substitution reduces pH i sensitivity | ||
I343 | Ala substitution reduces pH i sensitivity | ||
F345 | Ala substitution reduces pH i sensitivity | ||
E346 | Ala or Asp substitution acid-shifts pH o (50) , reduces pH i sensitivity | ||
E347 | Ala or Asp substitution acid-shifts pH o (50) , reduces pH i sensitivity | ||
W356 | Ala substitution reduces pH i sensitivity | ||
H360 | Glu substitution acid-shifts pH o (50) , reduces pH i sensitivity | ||
403–408 | Ala substitution acid-shifts pH o (50) | ||
C-term transmembrane | R789 | Glu and Ser substitution reduces AE-mediated Cl – transport but with wild-type surface expression | |
E888 (EC3) | Ala substitution acid-shifts pH o (50) | ||
K889 (EC3) | Ala substitution acid-shifts pH o (50) | ||
R921 (IC3) | Thr substitution reduces pH i sensitivity | ||
F922 (IC3) | Tyr substitution reduces pH i sensitivity | ||
D970/K971 (EC4) | Ala/Ser substitution increases pH i sensitivity | ||
E981 (EC4) | Leu substitution acid-shifts pH o (50) | ||
K982 (EC4) | Tyr substitution acid-shifts pH o (50) | ||
E1007 (inner TM8) | His, Gly, Lys substitution abolishes Cl – transport; Q substitution makes sulfate transport H + -independent | ||
H1029 (TM9) | Ala substitution inhibits Cl – transport; wild-type surface expression | ||
A1051 | Ser substitution alkaline-shifts pH o (50) | ||
A1053 | Thr substitution alkaline-shifts pH o (50) | ||
R1056 | Ala substitution inhibits Cl − transport; wild-type surface expression | ||
S1068 | Gly substitution acid-shifts pH o (50) , reduces pH i sensitivity | ||
V1071 | Ser substitution acid-shifts pH o (50) , reduces pH i sensitivity | ||
A1072 | Gly substitution acid-shifts pH o (50) , reduces pH i sensitivity | ||
D1075 | Ala substitution acid-shifts pH o (50) | ||
P1077 | Ala substitution reduces pH i sensitivity | ||
I1079 | Asp/Glu/Lys/Phe/Thr/Tyr substitution reduces pH i sensitivity, Glu or Lys substitution acid-shifts pH o (50). | ||
Cys substitution increases pH i sensitivity and alkaline-shifts pH o (50) | |||
R1107 | Ser substitution reduces pH i sensitivity | ||
R1134 | Cys substitution inhibits Cl − transport; apparent lack of surface expression | ||
H1144 | Tyr substitution alkaline-shifts pH o (50) | ||
H1145 | Ala substitution alkaline-shifts pH o (50) , increases pH i sensitivity | ||
R1155 | Lys substitution increases pH i sensitivity | ||
R1202 | Leu substitution increases pH i sensitivity |
Band 3 | Mutation | Ref | |
---|---|---|---|
A. SLC4A1 (AE1) MTATIONS ASSOCIATED WITH RED CELL SHAPE CHANGE | |||
Southeast Asian ovalocytosis | Δ400–408 | ovalocytosis (MIM:109270,130600,166900) | |
High transport (HT) | P868L | acanthocytosis, increased sulfate transport. | |
B. SLC4A1 (AE1) MUTATIONS ASSOCIATED WITH HEREDITARY SPHEROCYTOSIS (MIM:109270) | |||
Genas | nt 89 (G→A) from cap site in 5′ untranslated region; 33% decrease in mRNA | ||
Neapolis | Splice donor mutation retains intron 2 in primary transcript to yield two variant mRNAs: variant 1: intron 2 retention; polypeptide terminates after 19 neocodons. | ||
variant 2: Δ1–11; exon 2 skipping leads to deletion of first 11 aa to encode near full-length polypeptide with initiator codon at Met 12. | |||
Severe HS in homozygotes 12% eAE1 protein; mild HS in heterozyotes, 82% of eAE1 protein. | |||
Montefiore | E40K | recessive hemolytic anemia with protein 4.2 deficiency | |
Foggia | H55T-fs | 1-nt deletion | |
Kagoshima | E56fs | 1-nt deletion (A) | |
Hodonin | W81X | mutant mRNA undetected | |
Capetown | E90K | compound heterozygote with Prague III, both mRNAs present | |
Napoli I | S100F-fs-term; nt 447insT; mutant mRNA undetected | ||
Fukuyama I | R112–113 fs, 2-nt del (AC) aa | ||
Nachod | Δ117–12 intron-5 splice acceptor mutation, maybe near ankyrin binding site | ||
Fukuoka | G130R | asymptomatic alone, recessive, exacerbated HS when in trans with G714R Okinawa | |
Mondego | P147S | in cis with E40K Montefiore; in trans with V488M Coimbra. | |
Enhances severity of heterozygous HS V488M Coimbra | |||
Lyon, Osnabruck | R150X | ||
Worcester | L170–172 fs 1-nt insertion; mutant mRNA undetected | ||
Fukuyama II | D183 fs | 1-nt insertion | |
Campinas | Q203 fs | followed by 13-aa neosequence, 1-nt insertion. | |
incomplete dRTA by furosemide test, resiting bicarbonaturia | |||
Bohain | V241 fs | 1-nt deletion | |
Princeton | A273–275 fs | 1-nt insertion; mutant mRNA undetected | |
Boston | A285D | ||
Tuscaloosa | P327R | ||
Noirterre | Q330X | ||
Bruggen | P419 fs | 1-nt deletion | |
Benesov | G455E | in TM2 | |
Bicetre II | A456 fs | 1-nt deletion | |
Pribram | S477 fs | 1-nt deletion in splice acceptor, intron-12 retention, terminates after 7 neocodons | |
small amount mutant mRNA detected, mutant protein not detected | |||
also incomplete RTA by CaCl 2 load test, bicarbonaturia following <SPAN role=presentation tabIndex=0 id=MathJax-Element-34-Frame class=MathJax style="POSITION: relative" data-mathml='HCO3−’>HCO−3HCO−3 HCO3− HCO−3 HCO 3 − load | |||
Coimbra | V488M | exacerbated by in trans–double-mutant E40K/P147S | |
Bicetre I | R490C | ||
Evry | W492 fs | 1-nt deletion | |
Milano | I500G followed by duplicated aa 478–499; mutant protein undetected | ||
Dresden | R518C | ||
(Mexico) | G580fs63X | frameshift, 4 nt duplication, frameshift | |
Smichov | I616 fs | 1-nt deletion, mutant mRNA undetected | |
Trutnov | Y628X | mutant mRNA undetected | |
Hobart | R646–647 fs 1-nt deletion, mutant mRNA undetected | ||
Osnabruck II | M663–664 fs | ||
Tambau | M663K | de novo mutation | |
Most | L707P | ||
Okinawa | G714R | HS more severe when in trans with band 3 Fukuoka | |
Prague II | R760Q | mutant polypeptide not detected | |
Hradec Kralove | R760W | ||
Chur | G771D | mutant mRNA present at normal level | |
Napoli II | I783N | mutant mRNA present, mutant protein undetected | |
Jablonec | R808C | ||
Nara | R808H | ||
Prague I | V822 fs | 10-nt duplication | |
Birmingham | H834P | ||
Philadelphia | T837M | ||
Tokyo | T837A | mutant mRNA present at normal level | |
Prague III | R870W | in compound heterozygote with Capetown E90K, both mRNAs present | |
Vesuvio | T894 fs | 1-nt deletion replaces C-terminal 18 aa with 133 aa neoseq. | |
silent polymorphism 904 TAC-to-TAT in trans. | |||
mutant mRNA present, mutant protein undetected | |||
C. SLC4A1 (AE1) MUTATIONS ASSOCIATED WITH RED CELL CATION LEAK SYNDROMES | |||
Southeast Asian ovalocytosis | Δ400–408 | ||
Blackburn | L687P | ||
Horam | D705Y | ||
Hemel | S731P | ||
Hurstpierpoint | H734R | ||
R730C | |||
New Haven | E758K | ||
Prague II | R760Q | ||
S762R | |||
Ceinge | G796R | ||
D. SLC4A1 (AE1) MUTATIONS ASSOCIATED WITH DISTAL RENAL TUBULAR ACIDOSIS (MIM:179800,602272,109270) | |||
Capetown | E90K | compound heterozygous recessive dRTA | |
Sotheast Asian Ovalocytosis | Δ400–408 | compound heterozygous recessive dRTA | see below |
Edmonton | C479W | compound heterozygous recessive dRTA | |
Coimbra | V488M | homozygote shows complete absence of erythroid AE1, with hydrops fetalis, hemolytic anemia, accompanying recessive | |
dRTA heterozygote exhibits dominant dRTA with HS | see below | ||
Kaohsiung | E522K | compound heterozygous recessive dRTA | |
dRTA | R589H | dominant dRTA | |
dRTA | R589C | dominant dRTA | |
dRTA | R589S | dominant dRTA | |
dRTA | R602H | dominant dRTA as compound heterozygote with SAO | |
dRTA | G609R | dominant dRTA | |
dRTA | S613F | dominant dRTA | |
Courcouronnes | S667P | compound heterozygous recessive dRTA | |
Bangkok I | G701D | homozygous recessive dRTA | |
Unimas | Q759H | recessive dRTA as compound heterozygote with SAO | |
dRTA | S773P | recessive dRTA as compound heterozygote with G701D | |
dRTA | ΔV850 | homozygous recessive dRTA, and as compound heterozygote with SAO | |
dRTA | A858D | homozygous recessive dRTA, and as compound heterozygote with SAO | |
dRTA | A888L/889X dominant dRTA | ||
Walton | R901X | dominant dRTA, frameshift from 13-bp duplication | |
Qingdao | D905Gfs15 | dominant dRTA | |
dRTA | M909T | dominant | |
E. SLC4A1 (AE1) POLYMORPHISMS ASSOCIATED WITH BLOOD GROUP ANTIGENS | |||
NFLD | E429D | plus P561A | |
ELO | R432W | ||
Fra | E480K | ||
Rb(a) | P548L | ||
Tr(a) | K551N | ||
WARR | T552I | ||
Vga | Y555H | plus silent L441L | |
Wd(a) | V557M | ||
BOW | P561S | ||
Wu | G565A | ||
Jna | P566S | ||
KREP | P566A | ||
Moa | R656H | ||
Bpa | N569K | ||
Swa | R646Q | ||
Sw! | R646W | ||
Hga | R656C | ||
Wright b+ | E658 | common allele | |
Wright a+ | E658K | rare allele (a+/b+⊕1:1000 in general population) | |
Dia, Memphis II | P854L | Diego a+antigen, rare allele | |
Dib | P854 | common variant | |
F. SLC4A1 (AE1) ASYMPTOMATIC POLYMORPHIC VARIANTS | |||
Bangkok II | M31T | in cis with Memphis I and the dRTA mutation G701D | |
Napoli II | D38A | ||
Memphis I | K56E | 80% decreased phosphoenolpyruvate transport | |
Intron-3 polymorphisms | c87t, c242t*, g259a*, a580g (numbered from 5′ end of intron-3) | ||
(kAE1 promoter region); *, linked with Memphis I polymorphism | |||
Variant | E72D | found in HS assoc with other mutation | |
Variant | R112S | ||
Variant | I442F | ||
Variant | M586L | ||
Variant | I688V | ||
Variant | S690G | ||
Variant | R832H | ||
variant | A858S | ||
Variant | V862I | ||
G. ANIMAL MODELS | |||
B. taurus | Bovine equivalent of human R646X. Erythroid AE1 absent, also reductions in spectrin, ankyrin, actin, 4.2 | ||
Systemic acidosis with urine of less than maximally acidic pH | |||
D. rerio | Zebrafish retsina ret(b245) 1.5- to 2-cM deletion encompassing AE1 gene | ||
ret(tr265) E456G (aligns with human E472) | |||
Slightly reduced mRNA in early embryo, nonfunctional protein | |||
ret(tr217) frameshift 13 nt insertion at nt 503 (creates new splice acceptor in intron 5), greatly reduced mRNA, severely truncated protein | |||
All genotypes show phenotype of dyserythropoietic anemia type II (binuclearity with failure of cytokinesis) | |||
M. musculus | Mouse AE1(−/−). Insertion/disruption of exons 9–11 | ||
M. musculus | Mouse AE1(−/−). Insertion/disruption of exon 3 | ||
M. musculus | Band 3 wan. mAE1 Q85X missense terminator. Severe recessive HS | ||
Heterozygotes nonanemic | |||
Wan/wan on C3H background, no post-weaning survival, better on mixed background |
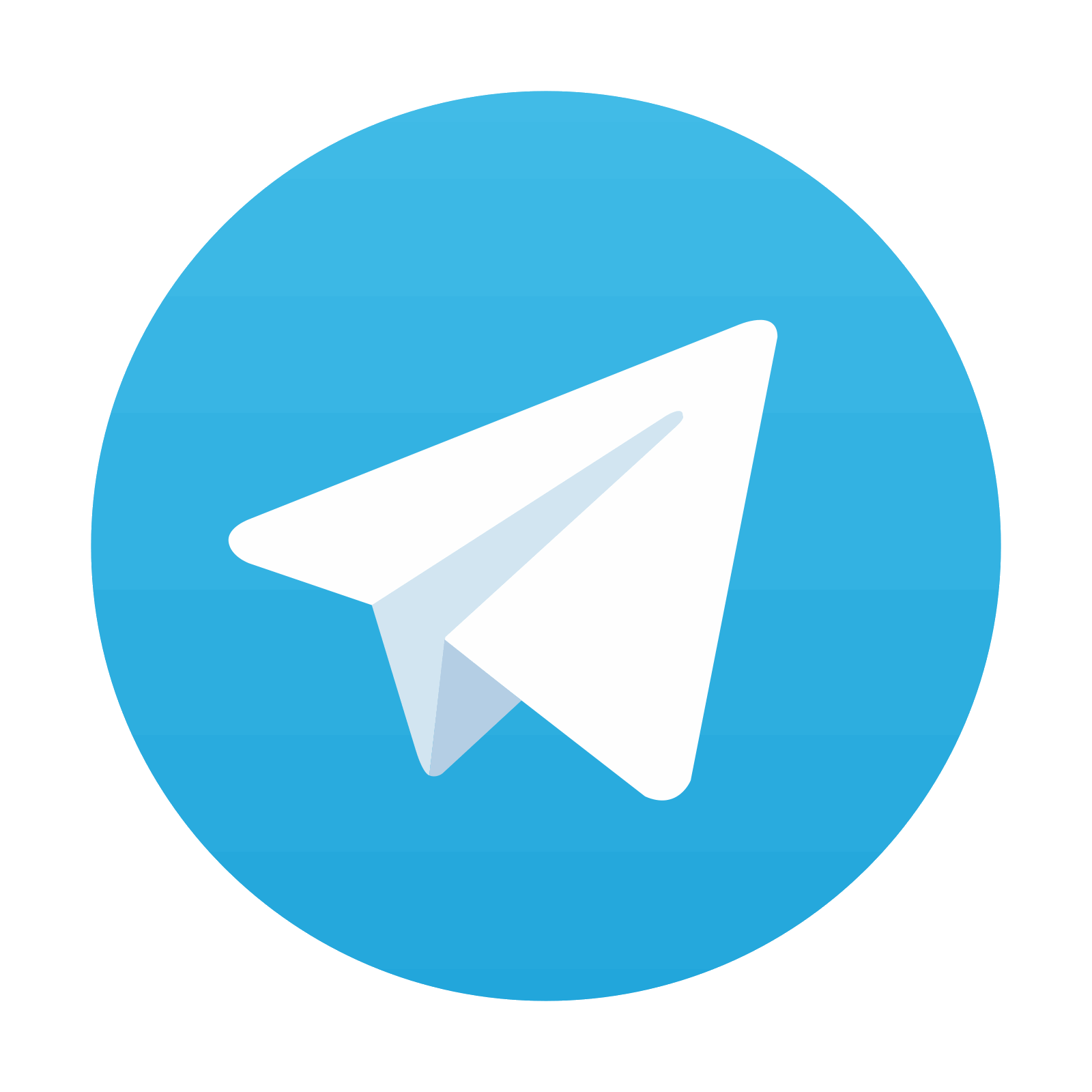
Stay updated, free articles. Join our Telegram channel
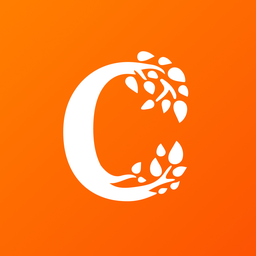
Full access? Get Clinical Tree
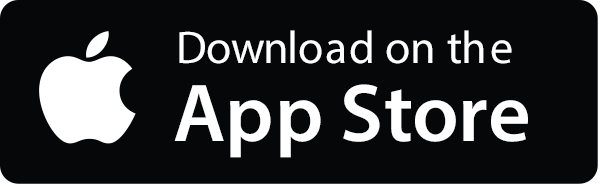
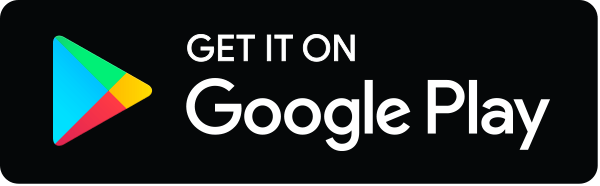
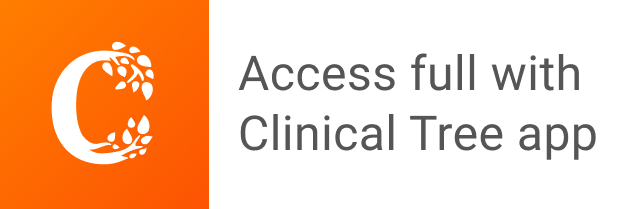