The kidney uses a large amount of energy, most of which are dedicated to solute reabsorption, especially Na + , from glomerular filtrate. Reabsorption of Na + drives the cellular and paracellular transport of water and other solutes. The mechanisms for energy production and preferred substrates are different among tubular segments. Renal metabolism for energy production is regulated by transport activity, and conversely, transport is affected by the cellular energy status. The intracellular adenine nucleotide level is an important regulatory factor for metabolism and transport; however, the interactions of these two processes are diverse and complex. For the last decade, AMPK was identified as the critical molecule for the regulator of cell metabolisms in various cells. Although the current information on its importance in the kidney is limited, emerging evidence has demonstrated its significance in the kidney. From pathophysiological viewpoints, the susceptibility of the kidney to ischemia and agents affecting energy production are critical clinical issues. Not only the role of acute ischemia, but also that of chronic ischemia in the kidney is now beginning to be a novel research subject. In this chapter the mechanisms of solute transport, energy consumption and production in the kidney are precisely reviewed.
Keywords
solute transport; energy production; Na + reabsorption; QO 2 ; primary active transport; Na + , K + -ATPase; preferred substrates; AMPK
Introduction
The kidney must reabsorb more than 99% of approximately 180 liters of water and 25,000 mmoles of Na + daily. To do this, the kidney consumes a large amount of energy. Although the kidney is only 0.5% of the total body weight, it utilizes approximately 7% of the oxygen consumed by the body. In fact, the kidney is the second only to the heart in terms of the rate of energy consumption. In this chapter, first we describe the energy consuming and production processes in the kidney. Second, the mechanisms of mutual relationships between energy consumption and production will be described. Significant advances have been made with regard to this issue, and AMPK (AMP-activated kinase) is considered to be the central for the regulation of energy consuming processes. Finally, we also refer to the pathophysiological states in which renal energy production is inhibited.
Energy Consumption
Na Transport and Energy Consumption in the Kidney
Na + Transport and O 2 Consumption
The energy utilized in the kidney is primarily required for the active reabsorption of Na + from the glomerular filtrate. This seems rational considering that the amount of Na + reabsorbed by the kidney is much higher than that of HCO 3 − (4,000 mmole/day), Ca 2+ (210 mmole/day), other electrolytes, and organic substances. The active Na + transport also energizes the reabsorption of water and other solutes by the osmotic gradient generated by Na + transport, and by the electrochemical gradient of Na + across the plasma membrane.
The data shown in Figures 6.1 and 6.2 indicate another important point. Extrapolation of the regression line to the Y ordinate indicates the basal oxygen consumption ( Figure 6.1 ). This value is identical to the energy used for cellular functions other than Na + transport, and was estimated to be between 3 to 18%, while other studies indicated a higher value for this basal O 2 consumption in the kidney.


With regard to energy production, the kidney generates approximately 95% of the ATP produced via aerobic mechanism, while in some nephron segments anaerobic metabolism also occurs efficiently. This is reasonable because of the highly efficient ATP production by mitochondrial oxidative phosphorylation compared to anaerobic glycolysis. Thirty-six moles of ATP are generated by the mitochondrial oxidative phosphorylation of one mole of glucose, whereas only two moles of ATP are produced via glycolysis in the absence of O 2 . Thus, historically, the relationship between Na transport and O 2 consumption (QO 2 ) in renal tissues has been extensively investigated.
Several investigators have examined Na + /O 2 stoichiomety in the kidney. Thurau demonstrated a linear relationship between Na + transport and QO 2 with a ratio of 28 Na + /O 2 in the whole dog kidney ( Figure 6.1 ). This stoichiometry is equal to a ratio of 4.6 for Na + /ATP, which indicates more efficient transport in the kidney compared to that in the frog skin and toad bladder. The discrepancy has been studied by many investigators and is attributed to the following.
First, there are alternative pathways for Na + transport in the nephron. In the leaky epithelia, such as proximal tubule (PT), paracellular Na + transport occurs, while only transepithelial transport is possible in the tight epithelia in cortical collecting duct (CCD) cells. Second, coupling of Na + transport to that of other solutes occurs, such as bicarbonate transport in PT, and Na + -K + -2Cl − transport via NKCC2 in the thick ascending limb of Henle (TAL). These two mechanisms produce more efficient Na + transport in certain nephron segments compared to that observed in frog skin and toad bladder, where only cellular transport of Na + driven by Na + ,K + -ATPase occurs. The different Na + /O 2 /ATP stoichiometry among individual nephron segments will be described below.
Heterogeneity in Na + Transport Efficiency among Nephron Segments
Proximal Tubule
In the PT, the Na + /QO 2 was reported to be 24 to 30, corresponding to a Na + /ATP ratio of 4 to 5. In the early portion of the PT (S1), preferential absorption of bicarbonate occurs, resulting in a rise in the luminal Cl − concentration, defined as axial anionic asymmetry. In the basolateral membrane of proximal tubular cells, a Na + -bicarbonate co-transporter (NBC) extrudes Na + with HCO 3 − with a stoichiometry of 1: 2~3. The electrochemical gradient of HCO 3 − across the plasma membrane in the PT cells, which is generated by the coordinated function of carbonic anhydrase and H + -ATPase or Na + -H + exchanger, drives Na + efflux via NBC. Because PT is more permeable to Cl − than to HCO 3 − , a driving force develops for isotonic fluid transport. It was suggested that 30% to 50% of Na + transport in the PT is passive, and not directly related to ATP consumption. In addition, in the early part of the PT, glucose, amino acids, and phosphate are actively reabsorbed by the Na + -co-transport mechanism, thereby rapidly reducing their concentration in the lumen. This luminal hypotonicity also contributes to the solvent drag which involves Na + . Thus, in the PT, more than 3 Na + could be transported via the hydrolysis of one mole of ATP.
Thick Ascending Limb of Henle
In the TAL, the most efficient sodium transport occurs, with the Na + -K + -2Cl − co-transporter (NKCC2) playing an important role. The transport by NKCC2 is electrically neutral, and the K + reabsorbed by NKCC2 in the TAL is leaked back into the tubular lumen via the ROMK channel in the apical membrane. This K + leakage results in a positive electrical potential difference in the lumen, which drives paracellular transport of Na + from the lumen to the plasma ( Figure 6.3 ). Although no direct measurement has been made of the Na + /O 2 /ATP stoichiometry in the TAL, the results obtained in the rectal gland and tracheal epithelium indicated that the Na + /ATP ratio could theoretically be up to 6 in the TAL.

CCD
In the CCD, the efficiency of Na + transport is the lowest among the nephron segments. In this segment, the junction between the epithelia is very tight, and paracellular transport is minimal. In addition, Na + entry from the luminal side into CCD cells is mediated only by ENaC, which is not associated with any coupled transport other than that of Na + . Thus, the Na + :ATP ratio in the CCD is estimated to be 3.
Energy Cost of Primary Active Transport
The cellular transport of electrolytes and organic substances is classified into three types, namely, primary active, secondary active, and tertiary active transport. Primary active transport refers to that which directly utilizes ATP hydrolysis energy to accomplish transepithelial transport. The primary active transporter in the plasma membrane of mammalian cells is further subdivided into three subtypes, i.e., P-type-ATPase, V-type-ATPase, and ABC transporter. The secondary active transporters utilize the Na + gradient across the plasma membrane generated by the Na + ,K + -ATPase. Na + -coupled co-transporters (e.g., Na + -glucose co-transporter, Na + -amino acid co-transporter) transport substrates with Na + in the same direction, while Na + -exchangers (e.g., NHE: the Na + -H + exchanger) transport substrate and Na + in opposite directions. In addition, there exist the tertiary active transporters. One example of this is the anion exchangers (AE: Cl − /HCO 3 − exchanger). The proton gradient generated by the coordinated function of Na + ,K + -ATPase (primary active transporter) and NHE (secondary active transporter) is used as the driving force for the exchange of Cl − and HCO 3 − by AE (tertiary active transporter).
The energy cost for secondary and tertiary active transport processes is attributed to the active transport via Na + ,K + -ATPase, which alone is accompanied by hydrolysis of ATP. Primary active transporters other than Na + ,K + -ATPase are also related to the energy consumption processes in the kidney. In general, the expression level of ATPases along the nephron segments correlates well with the transport activity of each solute.
P-type-ATPases
P-type-ATPases ( Figure 6.4 ), including Na + ,K + -ATPase, H + ,K + -ATPase, and Ca 2+ -ATPase, share several common features: (1) they possess a seven amino acid motif with aspartate to which ATP binds; (2) they are transiently phosphorylated during the cation transport cycle (the term P-type derives from this transient phosphorylation); and (3) they catalyze cation transport between E 1 and E 2 conformations (P-type-ATPase was previously called E 1 -E 2 -ATPase). The transport activity of P-type-ATPases is commonly inhibited by vanadate.

Na + ,K + -ATPase
Na + ,K + -ATPase extrudes 3 Na + and takes up 2 K + across the plasma membranes through the hydrolysis of one ATP molecule in the presence of Mg 2+ . Na + ,K + -ATPase generates an inwardly-directed Na + gradient and inside a negative electrical gradient. Na + ,K + -ATPase accounts for approximately half of the total Na + reabsorption in the kidney.
Na + ,K + -ATPase is a heterodimeric integral membrane protein, with a minimal composition of α- and β-subunits. The α-subunit possesses ten membrane-spanning domains with a molecular mass of approximately 100 kDa. The β-subunit is a glycosylated type II membrane protein with a molecular weight of approximately 55 kDa. In mammalian genomes, four α-subunits and at least three β-subunits of Na + ,K + -ATPase have been identified. In addition, a γ-subunit, a member of the FXYD family of type II transmembrane proteins, constitutes an Na + ,K + -ATPase. In the kidney, two γ-subunit isoforms are expressed. The combination of each isoform comprises a number of Na + ,K + -ATPase isozymes that are expressed in a tissue- and cell-specific manner to evolve distinct properties to respond to cellular requirements. In the kidney, α1β1 is predominantly expressed.
The α-subunit is the catalytic subunit of the Na + ,K + -ATPase, and α1, α2, and α3 isoforms differ in their affinities for ATP, Na + and K + . β-subunits are suggested to facilitate the correct membrane integration and packing of the α-subunit, and β-subunits also participate in the determination of the intrinsic transport properties of Na + ,K + -ATPase. The γ-subunit was shown to be a specific regulator of renal α1β1 isozymes. A putative dominant-negative mutation in the gene encoding the γ-subunit (FXYD2) which leads to defective routing of the protein in a family with dominant renal hypomagnesaemia indicates the physiological importance of the γ-subunit. The overall structure of the α-subunit of Na + ,K + -ATPase determined by electron crystallography using two-dimensional crystals is similar to the X-ray structure of Ca 2+ -ATPase.
The pump activity of Na + ,K + -ATPase has been investigated using direct measurement of hydrolysis activity, and axial heterogeneity in the nephron segments was demonstrated ( Figure 6.4 ). Na + ,K + -ATPase hydrolysis activity was high in the TAL, distal convoluted tubule (DCT), and proximal convoluted tubule (PCT), and low in the pars recta (PST) and collecting tubule (CT). Ouabain binding studies show the highest density of Na + ,K + -ATPase (20–30 fmol/mm length of tubule) in the DCT and the MTAL, intermediate density (10 fmol/ mm) in the PCT and connecting tubule (CNT), and lowest density (2–7 fmol/mm) in the PST, CTAL, and CT. The pump activity was proportional to the number of catalytic units. α1β1 has a maximum turnover rate of 7,700/min. The measurement of Na + ,K + -ATPase hydrolytic activity at V max , and initial rates of ouabain-sensitive Rb uptake indicated that in intact cells the pump works at approximately 20–30% of its V max . Western blotting analysis, RT-PCR using microdissected nephron segments, in situ hybridization, and immunohistochemical analysis demonstrated similar intranephron heterogenetic localization of Na + ,K + -ATPase consistent with those observed in the studies on pump activity.
Regulation of Na + ,K + -ATPase by dexamethasone, deoxycorticosterone, intracellular Na concentration, cAMP, potassium depletion, aldosterone and vasopressin was demonstrated. Many of these modulations influence the cell surface expression of Na + ,K + -ATPase, while MEK1/2 inhibitors changed the intrinsic activity rather than cell surface expression.
Ca 2 + -ATPase
Renal calcium transport is comprised of two processes: a paracellular, passive process that predominates in most nephron segments; and a transcellular, energy-dependent step in the DCT. Transcellular calcium transport involves: (1) entry into the DCT cell via a Ca 2+ channel (ECaC) across the luminal membrane; (2) intracellular Ca movement facilitated by the presence of the vitamin D-dependent calcium-binding protein (calbindin D); and (3) extrusion by the Ca 2+ -ATPase located at the basolateral membrane. The extrusion of Ca 2+ , the final step in the transcellular transport of Ca 2+ , is mediated by the plasma membrane Ca 2+ -ATPase (PMCA), which is a P-type-ATPase.
PMCA is a monomeric protein consisting of approximately 1,220 amino acids with a molecular mass of 140 kDa. The sequence contains the calmodulin-binding domain, and two domains resembling calmodulin, one of which may play a role in the binding of Ca 2+ . There are at least four isoforms of PMCA, and isoforms 1 and 4 are more widely distributed than 2 and 3. The activity of renal PMCA showed two saturable components: a high-affinity component with a Km of 33 μM ATP, and a low-affinity component with a Km of 0.63 mM ATP. PMCA is regulated by calmodulin, estrogen and dihydrotestosterone, protein kinase C or A, extracellular ATP, and pathophysiologic states such as hypercalciuria. RT-PCR, immunohistochemical analysis, and Western blot analysis demonstrated high expression of PMCA in the DCT. PMCA was also detected in Madin–Darby canine kidney (MDCK) cells. Doucet and co-workers examined sodium azide-insensitive plasma membrane Ca 2+ -ATPase activity. The Ca 2+ -ATPase was maximally activated by Ca 2+ concentrations with an apparent Km of 0.3–0.4 μM. Ca 2+ -ATPase activity was found in all segments of the nephron: activity was highest in the DCT and CCT, intermediate in the PCT and MTAL, and lowest in the PST, CTAL and MCT.
In addition to PMCA, there exists another distinct Ca 2+ -ATPase, the sarco/endoplasmic reticulum Ca 2+ -ATPase (SERCA), which also belongs to the P-type-ATPase. The SERCA family includes three gene products, SERCA1 (ATP2A1), SERCA2 (ATP2A2), and SERCA 3 (ATP2A3), which function in the removal of free cytosolic Ca 2+ into the sarco/endoplasmic reticulum. Although thapsigarigin is known to be a specific inhibitor of the endoplasmic reticulum Ca 2+ -pump, no study has been reported on the relative energy consumption rate of SERCA in the kidney.
H + ,K + -ATPase
H + ,K + -ATPase was originally characterized in a study of gastric mucosa. The gastric H + ,K + -ATPase is located in the apical membrane of stomach parietal cells, and mediates electroneutral exchange of K + and H + . Gastric H + ,K + -ATPase activity is independent of extracellular sodium, and is inhibited by vanadate.
Molecular cloning identified two types of H + ,K + -ATPase: gastric and colonic type H + ,K + -ATPase. H + ,K + -ATPase is comprised of α- and β-subunits. The catalytic α-subunit of gastric H + ,K + -ATPase shows structural similarity to that of Na + ,K + -ATPase, and the greatest homology occurs in the phosphorylation site region, and domains presumably involved in nucleotide binding and energy transduction. The α-subunit of colonic H + ,K + -ATPase exhibits 63% amino acid identity to that of the gastric H + ,K + -ATPase. The β-subunit of H + ,K + -ATPase shows 41% amino acid sequence identity to the β2-subunit of Na + ,K + -ATPase in the rat.
In the kidney, the existence of both gastric H + ,K + -ATPase and colonic H + ,K + -ATPase was demonstrated. Gastric H + ,K + -ATPase is expressed constitutively along the length of the collecting duct, is responsible for H + secretion and K + reabsorption under normal conditions, and may be stimulated with acid–base perturbations and/or K + depletion. The level of expression of colonic H + ,K + -ATPase is much lower in the kidney than in the distal colon.
Using in vitro microperfusion, Wingo and colleagues provided evidence of the existence of omeprazole-sensitive acidification and a K + -absorptive mechanism in OMCD in rabbits. By enzymatic analysis, Doucet and Garg quantified the K + -stimulated, Na-insensitive ATPase activity in the nephron segments. K + -stimulated ATPase activity was identified in the CNT, CCT, and MCT, although the activities were very low compared to those of other P-type-ATPases in the kidney. The renal K + -ATPase had a high affinity for K + (Km of approximately 0.2~0.4 mM) and was inhibited by vanadate, omeprazole, and SCH 28080, specific inhibitors of gastric H + ,K + -ATPase, but was insensitive to ouabain. A correlation between the magnitude of enzymatic activity and the percentage of intercalated cells in a given segment suggested that K + -ATPase activity originates in intercalated cells.
Immunohistochemical analysis revealed H + ,K + -ATPase in intercalated cells in the CCD and OMCD. In all segments studied, except for the CCD, the percentage of H + ,K + -ATPase-immunoreactive cells corresponded to the percentage of intercalated cells. The RT-PCR technique demonstrated the gastric H + ,K + -ATPase α-subunit in the CCD and IMCD, and a specific hybridization signal for the gastric H + ,K + -ATPase α-subunit cDNA was demonstrated. The colonic H + , K + -ATPase α-subunit is specifically expressed in the CCD and OMCD in K + -depleted rats. An increase in the H + ,K + -ATPase activity, and enhanced expression of gastric H + ,K + -ATPase α-subunit and colonic H + ,K + -ATPase in K + depletion, suggests the physiological adaptation of renal H + ,K + -ATPase.
V-type ATPases
V-type (vacuolar) ATPases represent the second family of ATP-dependent ion pumps. Vacuolar H + -ATPase is primarily responsible for the acidification of intracellular compartments such as endosomes, lysosomes, Golgi apparatus, and clathrin-coated vesicles. H + -ATPase is also expressed in the plasma membrane, and functions in acid–base transport in epithelia. In the kidney, vacuolar H + -ATPase mediates H + secretion, mainly in the PT and CCD.
H + -ATPase is a multi-subunit complex composed of two functional domains. The V(1) domain is a 570 kDa peripheral complex composed of eight subunits of molecular mass 73–14 kDa (subunits A–H) that is responsible for ATP hydrolysis. The V(o) domain is a 260 kDa integral complex composed of five subunits of molecular mass 100–17 kDa (subunits a, d, c, c’, and c”) that is responsible for proton translocation.
H + -ATPase is insensitive to vanadate or ouabain, but inhibited by bafilomycin A, N,N’-dicyclohexylcarbodiimide (DCCD: Ki= 50 μM) and N-ethylmaleimide (NEM: Ki= 20 μM). Physiological experiments indicated the existence of H + -ATPase in the PT. DCCD caused a fall in CO 2 absorption by 15% under eucapnia, and by 30% during acute hypercapnia in the PT. In other experiments, the S 3 segment was shown to possess plasma membrane H + -ATPase activity.
The relative contribution of H + -ATPase to ATP consumption by the kidney was examined by Noel et al. In dog proximal tubules incubated under control conditions, 81% of the respiration was directly related to oligomycin-sensitive ATP synthesis, and 29% of this amount was inhibited by bafilomycin A. In rabbit and hamster PT, the bafilomycin-sensitive ATP requirement involves only 5 and 10%, respectively, of the total ATP turnover. Thus, the metabolic cost of H + -ATPase in PT varies significantly among species.
Ait-Mohamed et al. examined the localization of NEM-sensitive ATPase in all the segments of the rat nephron; its activity was highest in the PCT; intermediate in the PST, TAL and CCT; and lowest in the OMCD. Immunocytochemical analysis demonstrated localization of rat H + -ATPase in the PCT, the initial part of the thin descending limb, TAL, DCT, and CT consistent with the aforementioned H + -ATPase activity.
Garg and co-workers examined the effect of acid-base balance and aldosterone on NEM-sensitive ATPase activity, and demonstrated the modulation of NEM-sensitive ATPase activity by metabolic acidosis and administration of aldosterone, and these effects were observed mainly in the CT.
The significance of H + -ATPase in final urinary acidification along the collecting system has been confirmed by hereditary defects in H + -ATPase. Mutations in the gene encoding the B1-subunit of H + -ATPase cause distal renal tubular acidosis with sensorineural deafness, and defects in the 116 kDa subunit ATP6N1B cause recessive distal renal tubular acidosis with preserved hearing.
ABC Superfamily
The third subgroup of primary active transporters is the ABC (ATP-binding cassette) transporter family. The prototype ABC transporter is the P-glycoprotein (P-gp) encoded by the MDR gene. P-gp was originally isolated as a drug extrusion pump in cancer cells which confers multiresistance to antineoplastic drugs. Later, P-gp was also shown to be expressed in normal tissues such as the kidney, intestine, and brain capillary cells, where it acts as a functional barrier to xenobiotics by extruding them from the tissues. Then, a subfamily of ABC transporters, the MRP (multidrug-resistance-associated protein) family, was identified, and the number of its isoforms is expanding rapidly.
The common molecular structure of ABC transporters is as follows; they possess two transmembrane (TM) domains, each with six TM segments and two nucleotide-binding domains, both of which can hydrolyze ATP. The stoichiometry of two ATPs hydrolyzed per molecule of drug transported was proposed.
Although the molecular properties of the members of ABC transporters, such as tissue distribution and substrate selectivity, have been extensively characterized, their significance in energy consumption in the kidney remains to be investigated, and to date no information is available.
Comparison of Ion Transporting ATPase Activities and QO 2 along the Nephron
In the upper panel of Figure 6.5 a comparison of the ion transporting ATPase activities is shown. In the lower part, relative distribution of QO 2 along the nephron segments is depicted.

Metabolic Basis in the Kidney
Energy Production Pathway in the Kidney
ATP synthesis in the kidney is mainly performed by mitochondrial oxidative phosphorylation, and a variety of energy fuels, such as glucose, fatty acids, and ketone bodies, are metabolized. Anaerobic glycolysis also occurs in certain nephron segments. Because of its heterogeneity in structural and functional properties, metabolic pathways and preferred substrates are distinct among the nephron. In this section, metabolic basis in the kidney and individual nephron segments are described.
Mitochondrial Oxidative Phosphorylation
Mitochondrial oxidative phosphorylation ( Figures 6.6, 6.7 ) is comprised of the following three steps: (1) production of reduced equivalents, i.e., NADH and FADH 2 , mostly by the TCA cycle in the matrix of mitochondria; (2) electron transfer via the mitochondrial respiratory chain in the inner membrane of mitochondria, associated with proton extrusion across the inner membrane of mitochondria; and (3) ATP production by F 0 F 1 -ATPase using the proton gradient generated ( Figures 6.6 and 6.7 ). The mitochondrial respiratory chain catalyzes electron transfer via four large multimeric integral membrane protein complexes (complex I to IV), ATP synthase (alternatively called complex V), and two relatively small hydrophobic proteins, i.e., ubiquinone (Q: Coenzyme Q) and cytochrome c.


Tricarboxylic Acid Cycle
The tricarboxylic acid (TCA) ( Figure 6.8 ) cycle is present in all mammalian cells, except those lacking mitochondria such as mature red blood cells. The TCA cycle oxidizes acetyl CoA derived from carbohydrates, fatty acids, amino acids, and ketone bodies, and produces NADH and FADH 2 . In addition, the TCA cycle provides intermediates that are utilized for the formation of glucose, lipids, and amino acids. Thus, the TCA cycle is central for metabolism, and is regulated to meet a variety of cellular metabolic demands.

Le Hir et al. assayed three TCA cycle enzymes, i.e., oxoglutarate dehydrogenase, citrate synthase and isocitrate dehydrogenase, in dissected rat nephron segments. The activities of the enzymes were higher in distal segments (TAL and DCT) than in the PT. The distal versus proximal ratios of activities were about 1.5, 2.5, and 2 for oxoglutarate dehydrogenase, citrate synthase, and isocitrate dehydrogenase, respectively. Oxoglutarate dehydrogenase showed the lowest activity along the entire nephron segments, and appeared to catalyze the rate-limiting step of the TCA cycle. Marver et al. determined citrate synthase levels in isolated rabbit nephron segments. The order of relative citrate synthase activities in normal rabbit nephron segments (per kg of dry tissue) was as follows: DCT> PCT> CTAL> CCD> PST. The activity in CCD was regulated by aldosterone.
β-Oxidation of Fatty Acids
Fatty acid is a major energy fuel in the kidney. β-Oxidation of short-, medium- and long- chain fatty acids ( Figure 6.8 ) occurs in mitochondria, and that of very long-chain fatty acids in peroxisomes. The 3-hydroxyacyl-CoA dehydrogenase activity, which mainly represents the mitochondrial β-oxidation pathway, is similarly distributed in all cortical proximal and distal segments, and is much lower in glomeruli and collecting ducts. The peroxisomal fatty acyl-CoA oxidase is restricted to the PT, with a capacity comparable to that in liver cells.
Ketone Body Metabolism
The kidney, as well as the muscle and brain, utilizes ketone bodies as metabolic fuel, while the liver cells do not. Acetoacetate and β-hydroxybutyrate are converted to acetyl CoA in the mitochondrial matrix. In this process, three enzymes, i.e., D-3-hydroxybutyrate dehydrogenase, 3-ketoacyl CoA transferase (3-oxoacid CoA- transferase), and acetoacetyl CoA thiorase, are involved.
Guder and co-workers measured 3-oxoacid CoA- transferase, and D-3-hydroxybutyrate dehydrogenase in mouse nephron. The activities of these enzymes were high in TAL and DCT, but decreased to nearly 20% in the CCD. In the PCT and PST, the 3-oxoacid CoA-transferase activity was almost equal, while the 3-hydroxybutyrate dehydrogenase activity was five-fold higher in PST than in PCT. In glomeruli and thin descending limbs of Henle’s loop, the enzymatic activities were markedly low. These results indicate that 3-hydroxybutyrate and acetoacetate can be metabolized in all of the mouse nephron segments with different capacities. The enzymatic activity for ketone body oxidation mirrors the distribution of mitochondria along the nephron segment.
Glycolysis
The role of glycolysis ( Figure 6.8 ) as a metabolic pathway is different among cells and the state of oxygen supply. In the kidney, glycolysis occurs primarily from glucose, since the storage of glycogen is minimal. The hexokinase activity in single microdissected rabbit nephron segments was the lowest in the PCT, and increased along the nephron segments. It was the highest activity in the CNT. The activities of phosphofructokinase and pyruvate kinase in the rat nephron were ten-fold higher in the distal nephron than in the proximal portion. Thus, the glycolytic activity in the kidney is mostly distributed along the distal part of the nephron. Lactate production from glucose, with and without antimycin A, was investigated in the rat nephron. PT produced no lactate, and the distal segments all produced lactate. Antimycin A, an inhibitor of mitochondrial oxidation, increased lactate production significantly in all of the distal segments. The increase was the largest in the MTAL (1400%), cortical (798%), and outer medullary collecting ducts (357%). Increments were smaller in CTAL (98%) and DCT (98%), and were the lowest in the IMCD (28%). Thus, anaerobic glycolysis is important, particularly in the distal segments of the nephron.
Gluconeogenesis
The capacity of the kidney to conduct gluconeogenesis ( Figure 6.8 ) was demonstrated more than 50 years ago. Actually, the glucose synthesis rate of the kidney is higher than that of the liver when they are compared using the same tissue amount. The release of glucose by the kidney has been reported to account for ~20% of all glucose released into the circulation in postabsorptive healthy humans. Sustained hypoglycemia enhances the renal extraction of circulating precursors for gluconeogenesis, and stimulates renal glucose production, which may represent an important additional component of the body’s defense mechanism against hypoglycemia in humans.
Among the nephron segments, the PT is the only site where net glucose synthesis occurs. The gluconeogenic enzyme PEPCK was exclusively found in the PT, and the PST exhibited 50% of the enzyme activity in the PCT. All other renal structures exhibited only negligible PEPCK activity. Thus, the activities of glycolysis and gluconeogenesis are a mirror image. Meyer et al. demonstrated that lactate is the most important precursor in renal gluconeogenesis, which exceeded the sum of renal gluconeogenesis from glycerol, glutamine, and alanine. Gluconeogenesis from these substrates accounts for ~90% of renal glucose release.
Kondou et al. demonstrated that renal gluconeogenesis is stimulated by short-term ischemia. They suggested that such stimulating gluconeogenesis supplies an energy fuel for further regeneration in nephron segments other than the PT.
There are several studies indicating a reciprocal relationship between Na + transport and gluconeogenesis. The inhibition of Na + ,K + -ATPase by ouabain stimulated gluconeogenesis. In contrast, the stimulation of Na + ,K + -ATPase by nystatin and monesin inhibited gluconeogenesis. Silva et al. suggested that under certain circumstances, gluconeogenesis competes with Na + reabsorption in an intact kidney.
Metabolic Parameters along Nephron Segments
As described, aerobic and anaerobic metabolisms are different among the nephron segments ( Figure 6.8 ). This difference is in good accord with the distribution of mitochondria along the nephron. Pfaller and colleagues investigated the density of mitochondrial volume (mitochondrial volume per unit volume of cytoplasm) by stereoscopic analysis, and demonstrated PCT (33%), PST (22%), thin limb (6–8%), MTAL (44%), DCT (33%), CCD (20%), and MCD (10%) ( Figure 6.8 ).
Intracellular ATP contents in various nephron segments were measured by several investigators. Uchida and Endou demonstrated that the cellular ATP content is largely dependent on the exogenous substrates available ( Figure 6.9 ).When appropriate exogenous substrates are present, the cellular ATP content is high in the PT, MTAL DT, and CCD, whereas it is low in glomerulus, CTAL, and MCD.

Preference of Metabolic Substrates in Nephron Segments ( Figure 6.10a,b )
Substrate Preference Along the Nephron Segments
Ever since Gyoergy et al. identified differences in metabolisms between the renal cortex and medulla in 1928, numerous studies identified different metabolic profiles along the nephron. In the previous section, the activities of metabolic enzymes in the nephron were described. However, preferred substrates for each segment do not always correlate well with the distribution of specific enzymes. The substrate preference in a specific nephron segment depends on several factors other than the enzymatic activities, such as the cellular uptake systems for substrates, oxygen supply, hormonal stimuli, and the demand for metabolic end products.

Knowledge of the preferred substrates for each segment has accumulated, especially from studies using microdissected nephrons. The determination of 14 CO 2 production from distinct labeled substrates, in conjunction with the effects of the substrate on cellular ATP level, QO 2 , redox state (NADH fluorescence), and active ion transports provide evidence that a variety of metabolic fuels, such as lactate, glucose, pyruvate, fatty acids, ketone bodies, amino acids, and TCA cycle intermediates energize cellular metabolism.
In this section, we briefly summarize the data on the substrate preference in each nephron segment.
Proximal Tubule (PT)
Numerous studies examined the metabolic profiles of the PT, and consistently indicated that the PT metabolizes a variety of substrates, such as fatty acids, ketone bodies, lactate, pyruvate, glutamine, glutamate, and TCA cycle intermediates. One notable exception is glucose: the PT, especially proximal convoluted tubule (PCT), poorly metabolizes glucose.
Glucose : PT poorly metabolizes glucose, and there is a difference in the utilization of glucose between PCT and the proximal straight tubule (PST). 14 CO 2 production from glucose in the PT was low, and glucose was poorly converted to lactate under both aerobic and anaerobic conditions. Uchida and Endou measured cellular ATP contents in mouse nephron segments in the presence of several exogenous substrates. When only glucose was supplied to the PT, the cellular ATP content significantly decreased in the S1 and S2 segments, while it was sustained in the S3 segment (PST). A study using rabbit PCT or PST suspensions demonstrated a similar metabolic profile for glucose: the addition of glucose to the rabbit PCT did not increase QO 2 , whereas that to the rabbit PST increased it by 57%. The differences in glucose utilization do not correlate with the distribution of glycolytic enzymes between the PCT and PST, suggesting that a metabolic regulation mechanism other than that by glycolytic enzymes may determine the ability of each segment to utilize glucose. It should be noted that the gluconeogenic activity is high in the PT.
Fatty acids : Short-chain fatty acids are important fuels in the PT. Balaban and Mandel examined the effects of various short-chain fatty acids, carboxylic acids, and amino acids on NADH fluorescence and QO 2 in the rabbit PT. The short-chain fatty acids (butyrate, valerate, and heptanoate) were the most effective in increasing NADH fluorescence and QO 2 , followed by the carboxylic acids and amino acids. Butyrate supported mitochondrial respiration to a greater degree than lactate, glucose, and alanine when the Na + ,K + -ATPase activity was maximally stimulated by nystatin. Butyrate was also shown to enhance the volume regulation of the isolated nonperfused PST under hypoosmotic conditions by activating Na + Cl − transport. Ruegg et al. demonstrated that PT exhibits maximal QO 2 and ATP content when incubated in culture medium with 2 mM heptanoate. In contrast, palmitate oxidation occurs minimally in the PCT. Thus, short-chain fatty acids are one of the best substrates, especially when active sodium transport is stimulated.
TCA cycle intermediates : The PCT demonstrated marked 14 CO 2 production from labeled succinate, 2-oxoglutarate, glutamate, glutamine, and malate (approximately 10 to 45 pmoles/mm/hr), and moderate 14 CO 2 production from citrate (approximately 3 pmoles/ml/hr). Gullans et al. demonstrated that succinate stimulates gluconeogenesis, and the hyperpolarization of the plasma membrane potential, and promotes intracellular K + without altering Na + ,K + -ATPase activity. Since TCA cycle intermediates are highly hydrophilic substances, cellular metabolisms of TCA cycle intermediates require specific transporters in the plasma membrane. The sodium-dicarboxylate co-transporters, NaDCs encoded by the SLC13 family, are expressed mainly in both the luminal and basolateral membrane of the PT.
Ketone bodies : Guder et al. reported that ketone bodies, i.e., acetoacetate and β-hydroxybutyrate, are presumably the most preferred substrate as kidney fuel. In a study using kidney slices, acetoacetate was estimated to support up to 80% of renal energy demands. β-hydroxybutyrate supported ATP levels to the same extent as lactate and glutamine in isolated mouse S3, while S1 and S2 had a low capacity to metabolize β-hydroxybutyrate. These results correlate with the high enzyme activities for ketone bodies such as 3-hydroxybutylate dehydrogenase in this segment.
Lactate : Lactate is also a preferred substrate for the PT. Goldstein et al. investigated the extraction of substrates from the blood by the rat kidney in normal, acidotic, and diabetic ketoacidotic conditions, and demonstrated that lactate accounts for 78% of the total amount of substrates extracted in normal control rats. Exogenous lactate maintained the cellular ATP content in the mouse PT. However, Kline et al. indicated that a negligible amount of 14 CO 2 was released from the labeled lactate as well as glucose in the rat PCT.
Thus, the proximal tubule metabolizes a wide range of substrates. This seems to be, at least in part, due to the existence of cellular transport systems for various substrates in the PT. PT cells possess Na-dependent transport systems for most of the nutrients across the luminal membrane. Nutrient transporters also exist in the basolateral membrane of the PT. Recent molecular studies have revealed the exclusive distribution of these transporters in the PT.
In the rat PT, preferred substrates are ketone bodies, short-chain fatty acids, lactate, and TCA cycle intermediates. However, there are species differences: the rabbit PT has a limited capacity to oxidize ketone bodies.
The PT normally conducts transport work at 50 to 60% of its maximal respiratory capacity, and has significant amounts of endogenous fuels, probably neutral lipids, which support about half of the energy in the absence of exogenous substrates. Metabolism of endogenous fuel is suppressed when there is an adequate supply of exogenous substrates. The preferred substrates differ under different physiological conditions.
Thin Descending Limb of the Loop of Henle (TDL)
The data available regarding the metabolic profile of the TDL is insufficient. The mitochondrial density of this segment is low, and its oxidative metabolism is limited. Jung and Endou measured the cellular ATP content in the rat short loop of TDL (SDL) and the rat long loop of TDL (LDL) in the presence of alanine, glucose, glutamine, β-HBA, lactate, and pyruvate. They demonstrated that the substrate preference in SDL is pyruvate=glucose > glutamine=lactate=β-HBA > alanine, and that in the LDL is pyruvate=glucose=glutamine > alanine=β-HBA=lactate. They also demonstrated that ATP is depleted when the TDL is incubated in the absence of exogenous substrate, indicating a limited store of endogenous fuels in this segment.
Cortical Thick Ascending Limb of the Loop of Henle (CTAL)
In the TAL, the rate of Na + transport and QO 2 are high, and the mitochondria are enriched, suggesting there is active oxidative metabolism in this segment. Klein et al. measured 14 CO 2 production from 14 C-labeled substrates in the rat MTAL and CTAL. MTAL and CTAL oxidized glucose, 2-oxoglutarate, lactate, glutamate, and glutamine, but not malate, succinate, and citrate. Palmitate oxidation occurred in MTAL and CTAL. Lactate production from glucose in the rat nephron indicated that the distal segments produce a significant amount of lactate from glucose, and under anaerobic conditions (with antimycin A), lactate production increased significantly in all of the distal segments. The increase was the largest in the MTAL (1400%), CCD (798%), and OMCD (357%), whereas increments were smaller in CTAL (98%) and DCT (98%), and were the lowest in the IMCD (28%). Thus, CTAL possesses modest anaerobic glycolysis capacity. The ATP content in the mouse CTAL is maintained in the presence of glucose, β-OHB or lactate, but not with glutamine.
Wittner et al. investigated the substrate preference by measuring the short circuit current (Isc) in the isolated rabbit CTAL perfused in vitro . They further examined which sides, i.e., luminal or basolateral, substrates were taken up from. Isc rapidly decreased to 50% after 3 minutes and to 27% after 10 minutes without any exogenous substrates, indicating that Na + transport is strictly related to the presence of substrates in CTAL segments. When substrates were added from the luminal side, only butyrate sustained Isc, while all other substrates tested (pyruvate, acetate, β-OHB, D-glucose, and L-lactate) showed a marked decrease in Isc. When the substrates were added from the basolateral side, D-glucose, D-mannose, butyrate, β-OHB, acetoacetate, L-lactate, acetate, and pyruvate sustained the Isc, but citrate, α-ketoglutarate, succinate, glutamate, glutamine, propionate, caprylate, and oleate did not. This study clearly indicates that the cellular uptake system is an important determinant in the substrate metabolisms in the CTAL. In the CTAL, most substrates, except for butyrate, are taken up by basolateral nutrient transporters. In fact, cytochalasin B and phloretin, inhibitors of the facilitated glucose transporter (GLUTs), inhibited the sustained Isc by glucose.
Regarding fatty acids, the CTAL possesses mitochondrial β-oxidation activity (3-hydroxyacyl-CoA dehydrogenase), and 14 CO 2 is produced from palmitate, while oleate (C-18) does not sustain Isc in the CTAL. Taken together, glucose, lactate, pyruvate, ketone bodies, and fatty acids are the preferred exogenous substrates for the CTAL.
Medullary Thick Ascending Limb of the Loop of Henle (MTAL)
Chamberlin and Mandel measured QO 2 in MTAL suspensions. In the absence of exogenous substrates, the control QO 2 decreased only by 15%. Torikai et al. demonstrate a similar result in the rat MTAL. These results indicate that endogenous substrates support most of the energy required for the MTAL under normal conditions. However, nystatin-stimulated QO 2 was inhibited 36% in the absence of exogenous substrates, indicating that the oxidation of endogenous substrates cannot meet the ATP demand when Na + transport is fully stimulated. They also investigated the role of endogenous substrates in the MTAL. The inhibitors of fatty acid, carbohydrate or amino acid metabolisms further inhibited QO 2 , revealing that endogenous fatty acids, glycogen, and amino acids (or proteins) contribute to energy production in the MTAL. The addition of fatty acids or acetoacetate increased QO 2 in 10 mM glucose, indicating that the MTAL oxidizes exogenous fatty acids and ketones in addition to glucose. In the MTAL, organic acids failed to enhance QO 2 , possibly due to the absence of transport systems for organic acids. The rat MTAL generated 14 CO 2 from glucose, lactate, palmitate, glutamate, glutamine, and α-ketoglutarate. 14 CO 2 from succinate, citrate, and malate was minimal. Glucose, β-hydroxybutyrate, and lactate maintained the intracellular ATP content, whereas the effect of glutamine on the ATP content was partial. The tight coupling of Na transport and QO 2 was demonstrated in the MTAL. Furosemide inhibited oxygen consumption by 43%, and ouabain inhibited it by 42% ; 50% of the oxygen consumption of the MTAL cells is related to the transport of Na + and Cl − .
In the MTAL, anaerobic glycolysis is also an important energy source. Ten mimutes of anoxia led to only a 15% decrease in potassium content in the rabbit MTAL, and an anaerobic metabolism maintained 73% of cellular ATP during 10 minutes of anoxia. The exposure of anoxic tubules to iodoacetate produced a 57% decrease in ATP level, and a 33% decrease in potassium content. Lactate production was remarkably enhanced in MTAL (1400%) under anaerobic conditions with antimycin A.
Distal Convoluted Tubule (DCT)
Although studies have examined the metabolic and transport properties in DCT, such as the mitochondrial density, Na + ,K + -ATPase activity, and enzymatic activities, data regarding its substrate preference is limited. The ATP content of the DCT was maximal in the presence of β-OHB or lactate, and somewhat lower in the presence of glucose. Glutamine did not increase the ATP content in the DCT. The lactate production rates from glucose under aerobic conditions were comparable to that observed in the CTAL, whereas under anoxic conditions, the glycolytic lactate production rate was increased two-fold as observed in CTAL. These results indicate that DCT utilizes both oxidative metabolisms and anaerobic glycolysis.
Cortical Collecting Duct (CCD)
Torikai demonstrated that substrate deprivation for 30 minutes does not change the cellular ATP content in the rat CCD and OMCD. However, a marked decrease in the PT and medullary TDL, and a slight decrease in CTAL and MTAL were observed, suggesting that CCD and OMCD possess sufficient amount of endogenous fuels. Hering-Smith et al. demonstrated the dependence of CCD on oxidative metabolisms in the Na + reabsorption and bicarbonate transport in the rabbit CCD. There was no significant glycolysis or any difference in substrate-dependence of solute transport in the CCD. Na + reabsorption was optimally supported by a mixture of basolateral metabolic substrates (glucose, acetate, and fatty acid), whereas bicarbonate secretion was fully supported by either glucose or acetate. This result indicates that principal cells and intercalated cells differ not only in their morphology and function, but also in their metabolism. Alanine was not effective in the CCD. Nonaka and Stokes examined the effects of substrates on Na + transport in the rabbit CCD, and concluded that the majority of the energetic support of Na + transport appears to come from an oxidative metabolism. Glucose supports transport better than the other substrates tested, and lactate, pyruvate and some organic acids also provide near maximal support.
The CCD synthesized modest amounts of lactate from glucose under aerobic conditions, which increased eight times under anaerobic conditions. The addition of glucose, β-hydroxybutyrate or lactate, but not glutamine, restored the cellular ATP content, and glucose was the best substrate in CCD for maintaining the ATP level. Natke showed that the rabbit nonperfused CCD regulates the cellular volume against extracellular hypertonic solution when exogenous butyrate is available, although rat CCD possesses a relatively low enzymatic activity for β-oxidation.
Outer Medullary Collecting Duct (OMCD)
The OMCD cells play an important role in the final acidification of urine, which is mediated by apical H + -ATPase. The OMCD has a relatively low QO 2 , which is inhibited only by 8% by ouabaine. Several studies indicated the importance of anaerobic glycolysis in this segment. The ATP content in the OMCD did not significantly change with the addition of antimycin A. Lactate production from glucose was significantly increased in the outer medullary collecting ducts by 357% under anaerobic conditions. Under aerobic conditions, the ATP content was supported equally well by glucose, glutamine, lactate or β-hydroxybutyrate. Thus, glucose appears to be a preferred substrate for this segment, particularly under hypoxic or anoxic conditions, but other alternative substrates, such as glutamine, lactate or β-hydroxybutyrate, can be metabolized under aerobic conditions. The uptake of glucose by the OMCD, as well as that by the TAL cells, is mainly through a facilitated basolateral diffusion. Studies of H + transport and ATP content also indicated the existence of a significant amount of endogenous fuel, most likely glycogen in the OMCD.
Inner Medullary Collecting Duct (IMCD)
Substrate metabolism in IMCD cells has not been sufficiently analyzed. QO 2 in IMCD is lower than that in other nephron segments. Glycolysis under aerobic and anaerobic conditions was examined by several investigators. Addition of glucose to IMCD cells stimulates both QO 2 and lactate production, indicating that glucose can be readily metabolized to both CO 2 and lactate under aerobic conditions. Lactate production in the IMCD under aerobic conditions was three- to five-fold greater than that in other nephron segments in the outer medulla, such as the TAL or OMCD.
To evaluate the relative contributions of aerobic and anaerobic metabolism in the IMCD, the effects of specific inhibitors of mitochondrial oxidative phosphorylation and glycolysis were examined. Stokes et al. examined metabolism in rat renal papillary collecting duct cells, i.e., IMCD cells. In the presence of rotenone, glycolysis increased by 56% and maintained the cellular ATP level at 65% of the control. Without any exogenous substrates, IMCD respiration was normal, and had a nearly normal ATP content, but lactate production was markedly decreased. At normal PO 2 and in the presence of D-glucose, the IMCD cells showed a substantial amount of aerobic glycolysis, although their mitochondrial respiration was not rate-limiting. In the absence of glucose, the cells acquired the majority of their energy from an endogenous substrate. Kone and co-workers showed that the addition of glucose to IMCD cells results in the accumulation of 12% more intracellular K + , even in the presence of lactate and glutamine. The data indicates that glucose is a preferred substrate in IMCD.
Coupling of Transport and Metabolism in the Kidney
As described in the section “Energy Consumption,” Na transport and QO 2 show a linear relationship in the kidney. The nature of the cellular mechanism linking active transport to energy production is a fundamental physiological question. Alterations in the rate of active transport cause changes in the mitochondrial state and/or concentrations of adenine nucleotides in epithelia. Conversely, the cellular respiration rate and/or ATP concentration affects active transport. This section focuses on the coupling mechanism of transport and energy production in the kidney.
The Effect of Active Transport on Metabolism
Whittam Model: Intracellular Signaling Between Transport and Energy Production
Whittam and co-workers primarily proposed a simple model indicating the coupling of active transport and mitochondrial respiration ( Figure 6.11 ). In this model, the rate of active cation transport is a pacemaker for cellular respiration in the renal cells: increased ATP hydrolysis and elevated cytosolic levels of ADP and Pi by Na + ,K + -ATPase activity would activate mitochondrial oxidative phosphorylation and oxygen consumption. Conversely, decreased Na + ,K + -ATPase activity would induce the opposite result ( Figure 6.11 ).

The validity of this model has been examined by: (1) direct measurement of ATP, ADP, Pi; and/or (2) monitoring of the mitochondrial redox state, in various states of transport. Early investigations into the intracellular nucleotide concentration failed to detect a change in the intracellular ATP levels. This was probably due to the rapid ATP turnover in the renal cortex; the half-life of ATP in the anoxic state was estimated to be as low as 3.3 seconds. Balaban and co-workers measured the cellular ATP/ADP concentrations and the QO 2 of a rabbit cortical tubule suspension under ideally designed conditions. They demonstrated that: (1) ouabain caused a 54% inhibition of QO 2 and a 30% increase in the ATP/ADP ratio; and (2) the addition of K + (5 mM) to K + -depleted tubules caused an initial 127% stimulation of QO 2 , followed by a new steady-state QO 2 50% above the control, and a 47% decrease in the cellular ATP/ADP ratio.
The monitoring of the redox state by optical measurements also demonstrated appropriate “mitochondrial state of transition.” Stimulation of Na + ,K + -ATPase activity stimulated QO 2 and decreased NADH fluorescence (in whole kidney and proximal tubules). Inhibition of Na + ,K + -ATPase with ouabain decreased QO 2 and increased NADH. Addition of rotenone to proximal tubules decreased QO 2 , and ATP content net fluid transport with increase of NADH fluorescence.
Regulation of Mitochondrial Respiration
The precise mechanism by which the rate of mitochondrial oxidative phosphorylation is regulated is of major interest in the field. There is much evidence suggesting the importance of cytosolic ADP concentration. Chance and Williams first proposed that the availability of ADP determines the mitochondrial respiration rate. The respiratory state of mitochondria was classified into five states according to the supply of ADP, Pi, substrates, and O 2 . The addition of ADP to the mitochondria with a sufficient amount of substrates and O 2 induces the maximal rate of respiration, called “state 3 (active)” respiration. When all of the ADP is phosphorylated to ATP, QO 2 and ATP production decrease (the phase called “state 4 resting” respiration). The ratio of QO 2 in state 3 and state 4 is termed the “ respiratory control index. ” This change in respiration was also demonstrated in proximal tubules permeabilized to ADP by digitonin, in which QO 2 was stimulated by four- to five-fold by the addition of ADP. Nevertheless, the predominant parameter, (e.g., only [ADP] itself, [ATP]/[ADP] ratio or [ATP]/[ADP][Pi] ratio), should be determined by further studies using mitochondria, and two conflicting hypotheses on mitochondrial phosphorylation have been proposed.
The first hypothesis indicated that the [ATP]/[ADP] ratio is the rate-limiting step in oxidative phosphorylation. This hypothesis is founded on kinetic considerations of adenine nucleotide translocase. Addition of atractyloside, an inhibitor of ATP/ADP translocase, caused inhibition of ADP influx into the mitochondrial matrix and oxidative respiration. However, the data from intact tissues with high oxidative phosphorylation capacities, i.e., heart, brain, and kidney, indicated that the cytosolic concentration of ADP and Pi do not change significantly with work.
The second hypothesis, called the “near-equilibrium theory,” stated that oxidative phosphorylation is dependent on the phosphorylation potential. This hypothesis suggested that oxidative phosphorylation is regulated thermodynamically by four factors: (1) [ATP]/[ADP][Pi] ratio; (2) intramitochondrial [NAD + ]/[NADH] ratio; (3) respiratory chain components, especially cytochrome c oxidase; and (4) oxygen concentration. This theory was partially correct, however, oxidative phosphorylation is not always close to equilibrium, at least in isolated mitochondria.
Furthermore, both of these hypotheses should be re-evaluated by the determination of the cytosolic concentration of ATP, ADP, and Pi by nuclear magnetic resonance (NMR) spectroscopy. NMR spectroscopy is a method by which the radiofrequency signal of specific molecules (P, N, C, and H) in a strong magnetic field can be recorded and quantified. There are particular features of this technology which can be applied to the measurement of biological events as follows: (1) the ability to define the chemical nature of phosphorus-containing molecules and follow their transition in time; (2) completely nondestructive and repeated determination is possible; (3) rapid determination over a few seconds; and (4) wide application from isolated mitochondria to intact kidney in vivo . Freeman and colleagues quantified inorganic phosphate (Pi) and high-energy phosphates in the isolated, functioning perfused rat kidney. Compared with enzymatic analysis, 100% of ATP, but only 25% of ADP and 27% of Pi were visible by NMR spectroscopy, indicating that a large proportion of both ADP and Pi are bound to proteins in the intact kidney. The data obtained by NMR spectroscopy, in conjunction with biochemical assays, estimated the free concentration of cytosolic ADP as approximately 30 μM and Pi as 0.6 mM. As a consequence, the [ATP]/[ADP][Pi] ratio (phosphorylation potential) and [ATP]/[ADP] ratio should be at least one order of magnitude higher than previously estimated values.
There are several other theories on the regulation of mitochondrial respiration. One claimed that the interplay of all aspects of oxidative phosphorylation affects respiration control. Another new hypothesis implies the regulation of respiration and ATP synthesis via allosteric modification of respiratory chain complexes, in particular of cytochrome c oxidase by metabolites, cofactors, ions, hormones, and the membrane potential. At the moment, there seems no simple answer to the question “what controls respiration?” The answer varies with: (1) the size of the system examined (mitochondria, cells or organs); (2) the conditions (rate of ATP use, level of hormonal stimulation); and (3) the particular organ examined.
The Effect of Metabolism on Active Transport
Intracellular ATP and Cation Transport
The intracellular ATP content ( Figure 6.12 ) was reported 2~8 mM, and the Km value of α-subunits for ATP was estimated to be 0.1 to 0.4 mM. Therefore, Na + ,K + -ATPase should be saturated for ATP under physiological conditions. However, intact renal cells normally function at almost half of their maximal respiratory capacity. Harris measured cellular QO 2 during stimulation and inhibition of the Na + ,K + -ATPase in mitochondria released from the rabbit renal tubules by digitonin shock. In the presence of NADH-linked substrates and fats, isolated renal cells respired at 50 to 60% of the maximum occurring in state 3 respiration, and addition of ouabain resulted in a decline in respiration to 25 to 30%. Stimulation of Na + ,K + -ATPase by nystatin resulted in increased respiration with increased oxygen consumption. Gullans and colleagues demonstrated that partial inhibition of oxidative metabolism with rotenone caused proportional reduction in QO 2 , ATP content, and absorption rates of fluid and phosphate. The effect of inhibition of oxidative metabolism on transport systems was also demonstrated using arsenate, which uncouples oxidative phosphorylation.
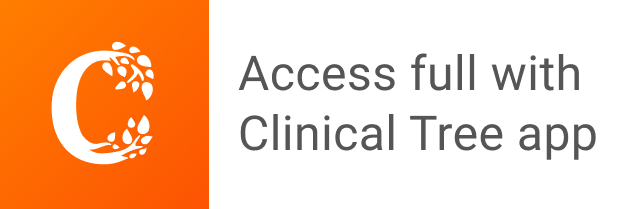