The primary or immotile cilium is a nearly ubiquitous microtubule-based structure. It was once considered a vestigial remnant; however, recent advances have revealed that the cilium functions as a critical sensory and signaling center allowing cells to respond efficiently to external environmental cues. Seminal discoveries demonstrating that defects in cilia of mammals cause abnormal left–right body axis specification and cystic kidney disease have ignited strong research and clinical interest into the roles of the cilium. Defects in ciliary proteins are now known to be the cause of a group of disorders that are collectively called the ciliopathies . Patients with ciliopathies can present with a wide spectrum of disease phenotypes. These include cysts in the kidney, liver and pancreas, hydrocephalus, obesity, blindness, anosmia, cognitive deficits, ataxia, left-right body axis abnormalities, polydactyly and other bone malformations, deafness, and sterility. The molecular and cellular pathogenesis responsible for these phenotypes is beginning to emerge. This is occurring through research using model organisms such as Chlamydomonas and C. elegans , through identification of mutations in human patients with ciliary associated phenotypes, and through classical in vitro cell and biochemical studies. Currently, mutations in more than 45 genes have been identified in human ciliopathy patients; however, in most cases the genetic defect responsible for the phenotype is unknown. The ciliopathy phenotypes are thought to be a consequence of abnormal regulation of the diverse collection of receptors, channels, and other signaling machinery that are specifically enriched in the cilium. Importantly, these include the polycystin proteins, mutations in which cause polycystic kidney disease, one of the most common genetic diseases in humans.
Keywords
cilia; cyst; intraflagellar transport; signaling; microtubule; ciliopathy
Introduction
The first descriptions of the cilium are generally attributed to Leeuwenhoek (as translated in ). Cilia were thought to be analogous to the flagellum used by single cell organisms for motility. It soon became obvious that motile cilia also have a function in multiple human tissues; motile cilia found in the respiratory track are involved in clearance of mucus and debris, cerebral spinal fluid movement is assisted by motile cilia on ependymal cells lining the brain ventricles, and flagella function to propel sperm through the reproductive tract for fertilization. In fact, the first human disease associated with cilia dysfunction was Primary Ciliary Dyskinesia (“primary” here referring to the fact that impaired movement of cilia was the cause of the disease (PCD) ). These patients suffer from chronic rhinitis, bronchiectasis, infertility, and abnormal left–right body axis specification caused by defects in cilia motility.
Most cell types in the mammalian body do not have motile cilia; they possess a single immotile cilium (referred to as the primary cilium ). The functional importance of the primary cilium remained enigmatic, and the primary cilium was often considered vestigial. It wasn’t until the mid-1990s and early 2000s that a number of essential observations were made linking the primary cilium to disease phenotypes, largely through studies in model organisms. One of the initial breakthroughs came from a large-scale insertional mutagenesis project conducted on mice in the laboratory of Dr. Rick Woychik at the Oak Ridge National Laboratories. This screen resulted in the identification of the Oak Ridge Polycystic Kidney ( orpk ) mouse, a genetic model for autosomal recessive polycystic kidney disease (ARPKD, OMIM# 263200). orpk mutants have multi-organ defects, including cystic lesions in the kidney, liver, and pancreas, hydrocephalus, anosmia, retinal degeneration, skin and hair abnormalities, brain malformation, and skeletal defects. Many of the phenotypes observed in the orpk mutants have also been reported in human disorders caused by mutations in proteins associated with the cilium ( Table 11.1 ). These disorders are collectively referred to as “ ciliopathies ,” and the orpk mutant mouse has become an important mammalian model system for studying ciliary dysfunction and disease. The transgene insertion in orpk mutant mice caused a hypomorphic mutation in a gene called “ Tg737 ” that encodes the protein Polaris. Polaris localizes to the cilium, and in the orpk mutants both motile and primary cilia were stunted and malformed, although the function of the protein remained unknown.
Gene Name | Gene Location | Protein Name | Aliases | Overlapping Loci | Associated Ciliopathies | Protein Characteristics | Subcellular Localization |
---|---|---|---|---|---|---|---|
BBS1 | 11q13.2 | BBS1 | BBS2L2 | BBS | BBSome component | Basal body; cilium | |
BBS2 | 16q12.2 | BBS2 | – | BBS | BBSome component | Basal body; cilium | |
ARL6 | 3q11.2 | Arl6 | – | BBS | GTPase | Basal body; cilium | |
BBS4 | 15q24.1 | BBS4 | – | BBS | BBSome component | Basal body; cilium | |
BBS5 | 2q31.1 | BBS5 | – | BBS | BBSome component | Basal body; cilium | |
MKKS | 20p12.2 | BBS6 | – | BBS | Chaperonin-like | Basal body | |
BBS7 | 4q27 | BBS7 | BBS2L1, FLJ10715 | BBS | BBSome component | Basal body; cilium | |
TTC8 | 14q31.3 | BBS8 | – | BBS | BBSome component | Basal body; cilium | |
PTHB1 | 7p14.3 | BBS9 | – | BBS | BBSome component | Basal body; cilium | |
BBS10 | 12q21.2 | BBS10 | – | BBS | Chaperonin-like | Basal body | |
TRIM32 | 9q33.1 | BBS11 | HT2A | BBS | E3 ubiquitin ligase motif; RING zinc finger | Cytosol; nucleus | |
BBS12 | 4q27 | BBS12 | FLJ35630 | BBS | Chaperonin-like | Basal body | |
WDPCP | 2p15 | BBS15 | Fritz | BBS | WD40 domains | Cytosol | |
INPP5E | 9q34.3 | INPP5E | CORS1, MORMS | JBTS | Inositol polyphosphate-5-phosphatase | Cilium | |
AHI1 | 6q23.3 | AHI1 | Jouberin | JBTS | SH3 domain; W40 repeats; coiled-coil domain | Cilium | |
ARL13B | 3q11.1 | ARL13B | ARL2L1 | JBTS | GTPase | Basal body; cilium | |
ACLS | 15q26.1 | Klf7 | KIF7, Costal2 | JBTS | Kinesin-family protein | Cilium | |
TCTN1 | 12q24.11 | Tectonic | TECT-1, Tectonic 1 | JBTS, MKS? | Transmembrane domain | Transition zone | |
MKS1 | 17q22 | MKS1 | MKS | BBS13 | MKS, BBS | B9 domain | Transition zone |
TMEM216 | 11q12.2 | MKS2 | JBTSB, CORS2 | JBTS2 | MKS, JBTS | Transmembrane domain | Transition zone |
TMEM67 | 8q22.1 | MKS3 | Meckelin | JBTS6, NPHP11 | MKS, JBTS, NPHP | 7-pass transmembrane domain protein | Transition zone |
CEP290 | 12q21.32 | Cep290 | – | NPHP6, BBS14, JBTS5, SLSN6 | MKS, BBS, NPHP, JBTS, SLSN | Coiled-coil domains/bipartite NLS | Basal body/centrosome |
RPGR1P1L | 16q12.2 | MKS5 | NPHP8 | NPHP8, JBTS7 | MKS, NPHP, JBTS | C2 domains, coiled-coil domains, bipartite NLS | Transition zone |
CC2D2A | 4p15.32 | MKS6 | – | JBTS9 | MKS, JBTS | C2 domains, coiled-coil domains | Transition zone |
TCTN2 | 12q24.31 | Tectonic-2 | MKS | Transmembrane domain | Transition zone | ||
B9D1 | 17p11.2 | B9D1 | MKS | B9 domain | Transition zone | ||
B9D2 | 19q13.2 | B9D2 | MKS | B9 domain | Transition zone | ||
NPHP1 | 2q13 | NPHP1 | JBTS4, SLSN1 | NPHP, JBTS, SLSN | SH3 domain/coiled-coil domain | Transition zone, adherens junctions | |
INVS | 9q31.1 | Inversin | NPHP2 | NPHP | IQ calmodulin-binding domain/ankyrin repeats | Inversin domain, cilium | |
NPHP3 | 3q22.1 | NPHP3 | Nephrocystin-3, NPH3 | MKS7, SLSN3 | NPHP, MKS, SLSN | IQ calmodulin-binding domain | Cilium |
NPHP4 | 1p36.31 | NPHP4 | Nephroretinin | SLSN4 | NPHP, SLSN | SH3 domain | Transition zone, adherens junctions |
IQCB1 | 3q13.33 | NPHP5 | PIQ | SLSN5 | NPHP, SLSN | IQ calmodulin-binding domain | Inversin domain, cilium |
GLIS2 | 15p13.3 | Glis2 | – | NPHP | Kruppel-like zinc finger | Cilium | |
NEK8 | 17q11.2 | Nek8 | NPHP9, JCK | NPHP | NIMA kinase | Inversin domain, cilium | |
SDCCAG8 | 1q43 | SDCCAG8 | NPHP10, CCCAP | SLSN7 | NPHP, SLSN | Coiled-coil domains, colon cancer auto-antigen | Basal body/centrosome |
XPNPEP3 | 22q13.2 | NPHP-1L | APP3 | NPHP | Peptidase | Mitochondria | |
ATD | 15q13 | ATD1 | ATD | ATD | Unknown | Cilium? | |
IFT80 | 3q25.33 | IFT80 | WDR56 | ATD | IFT-B complex, WD40 domains | Cilium | |
DYNC2H1 | 11q22.3 | Dync2H1 | DHC2, DHC1B | ATD | Cytoplasmic dyenin component | Cilium, cytosol | |
TTC21B | 2q24.3 | IFT139 | THM1 | JBTS11, NPHP12 | ATD, NPHP, JBTS | IFT-A complex, TPR repeats | Cilium |
IFT122 | 3q21.3–q22.1 | IFT122 | WDR10 | CED | IFT-A complex, WD40 domains | Cilium | |
WDR35 | 2p24.1 | IFT121 | TULP4, Naofen | CED | IFT-A complex, WD40 domains | Cilium | |
IFT43 | 14q24.3 | IFT43 | – | CED | IFT-A complex | Cilium | |
OFD1 | Xp22.2 | Ofd1 | – | JBTS10 | OFD, JBTS | Coiled-coil domains | Basal body/centrosome |
ALSM1 | 2p13.1 | ALSM | ALSS | ALMS | Trafficking protein? | Cytosol | |
ATXN10 | 22q13.31 | Ataxin 10 | E46L, SCA10 | MKS? | ATTCT repeats | Perinuclear |
a Genes are categorized by ciliopathy based on Online Mendelian Inheritance in Man (OMIM, http://www-ncbi-nlm-nih-gov.easyaccess1.lib.cuhk.edu.hk/omim ) entries at time of writing.
Another major advance came from the proteomic analysis of flagella isolated from the green alga Chlamydomonas reinhardt . This study identified several components of the intraflagellar transport (IFT) particle that included intraflagellar protein 88 ( Ift88 ), the homolog of Polaris in Chlamydomonas . IFT is an evolutionarily conserved transport system that mediates bidirectional movement of proteins between the base and tip of the cilia/flagella, and is essential for the construction and maintenance of cilia/flagella ( Figure 11.1 ; Table 11.2 ; see section “Intraflagellar Transport (IFT) and IFT Motors”). In agreement with a role in IFT, further studies in Caenorhabditis elegans ( C. elegans ) on OSM-5, the IFT88/Polaris homolog, revealed that mutations disrupting this protein also cause severe defects in cilia assembly. Thus, the function IFT88 is highly conserved across diverse species.

IFTA-Complex | IFTB-Complex | IFT Anterograde Motors | IFT Retrograde Motors |
---|---|---|---|
IFT43 * | IFT20 | KIF3A | Cytoplasmic dynenin2 ^ |
IFT121 * | IFT22 | KIF3B | |
IFT122 * | IFT25 | KAP3 | |
IFT139 ^ | IFT27 | ||
IFT140 | IFT46 | ||
IFT144 | IFT52 | ||
IFT54 | |||
IFT57 | |||
IFT70 | |||
IFT74 | |||
IFT80 ^ | |||
IFT81 | |||
IFT88 | |||
IFT172 |
One of the most significant breakthroughs connecting cilia dysfunction to human diseases came from studies in C. elegans by Barr et al. They conducted a genetic screen to identify genes involved in male mating behavior and uncovered mutations in lov-1 and pkd-2 , the homologs of human polycystin-1 (PC-1) and polycystin-2 (PC-2), respectively. Importantly, mutations in these two genes cause human autosomal dominant polycystic kidney disease (ADPKD OMIM# 601313, # 173910); a disorder that affects nearly 1 in 1000 individuals and is a leading cause of end-stage renal disease. In C. elegans , both lov-1 and pkd-2 proteins localize to the cilium of male sensory neurons. Subsequently, the mammalian proteins were also localized in the cilium of renal epithelium leading to a paradigm shift in the understanding of the possible mechanisms involved in human PKD. Multiple cystic kidney disease genes in mice, rats, and humans have now been identified and in nearly every case, the affected proteins encoded by these genes are associated with the cilium (reviewed in ).
These initial observations in model systems ushered in a renewed interest into the functions and clinical importance of the primary cilium. Even though research into the role of primary cilia has now extended to almost every tissue in the body, the involvement of the cilium in the kidney has garnered particular focus. In part this may reflect the morbidity and mortality associated with PKD, as well as the high incidence of PKD.
Cilia Ultrastructure and Components
The primary cilium on renal epithelial cells is a small, membranous, hair-like extension protruding from the surface of the cell into the lumen of the nephron tubule. This simple description, however, disguises the high level of complexity that is necessary for the cilium to function as an intricate signaling and sensory organelle ( Figure 11.1 ). Below the membranous covering of the cilium lies a precisely ordered series of axoneme microtubules. At the base of the cilium, the axoneme microtubules are anchored to the cell and cell membrane by a series of specialized structural components including transition fibers, the transition zone, ciliary necklace, and a modified centriole termed the basal body . These structures are further defined below.
Centrosomes and Basal Bodies
Centrosomes are comprised of a pair of centrioles, an older mother centriole and a daughter centriole, surrounded by a proteinaceous matrix. Mother–daughter centrioles are aligned orthogonally, with the mother centriole aligned towards the cell surface. The mother centriole becomes the basal body, which acts as a microtubule-organizing center (MTOC) for axonemal nucleation at the base of the extending cilium. Basal bodies are comprised of a nine-ring helix of triplet microtubules that contain γ-tubulin, in addition to α- and β-tubulins ( Figure 11.1d ). Axoneme microtubule polymerization is initiated by nucleation of γ-tubulin-containing microtubules of the basal body.
In addition to their microtubule cores, basal bodies are also comprised of numerous associated proteinaceous appendages that facilitate ciliogenesis. Although the basal body appears symmetrical, the distribution of centriolar appendages gives the basal body asymmetry with respect to its proximal–distal axis. The basal foot (also called sub-distal appendages) and the transition fibers (also called distal appendages) extend from the more distal end of the basal body. The formation of transition fibers on the basal body is a distinct process from that of ciliogenesis, yet it has been demonstrated that transition fibers are necessary for cilia to form. Transition fibers appear necessary for tethering the basal body to the membrane (see section “Transition Fibers” and ), thus establishing the basis for cilia anchorage.
The proteinaceous matrix surrounding the basal body is termed the pericentriolar material and contains centriolar components. Although the pericentriolar material does not form the structure of the centriole/basal body, these proteins are necessary for centriolar organization and control of mitosis. Puncta of proteins surrounding centrioles (centriolar satellites) will often be dynamically associated with the centrosome depending on cell cycle stage.
Ciliary Axoneme
The core of both motile and primary cilium is an organized microtubule-based axoneme extending from the basal body. Axonemal microtubules have (+) ends, localized at the ciliary tip, and (–) ends anchored to the basal body. These microtubules serve as tracks for molecular motor protein transport of cargo along the cilium ( Table 11.2 and see section “Intraflagellar Transport (IFT) and IFT Motors”).
Axonemal microtubules are arranged in a ring of nine doublets ( Figure 11.1b ). Cryo-electron tomography imaging of cilia axoneme microtubles by Sui and Downing illustrate that each doublet is comprised of a larger A-tubule, and a smaller incomplete B-tubule. Both A and B tubules of the axoneme microtubles are comprised of α- and β-tubulin protofilaments similar to the cytoplasmic microtubules. The axonemal microtubule doublet may either extend the entire length of the cilium or terminate into a mircotubule singlet that continues into the distal cilium ( Figure 11.1a ). Most motile forms of cilia also contain additional structures used in generating motility. These include a central pair of microtubules, in addition to their nine-ring doublet, radial spokes, inner and outer dynein arms, and nexin links. The incorporation of a central pair of microtubles in motile cilia is referred to as a 9+2 arrangement . However, not all 9+2 cilia are motile, as revealed by olfactory sensory neuron cilia. Immotile primary cilia, such as those found in renal epithelia, lack the central microtubule pair and many of the motility components. Lack of a central microtubule pair is referred to as a 9+0 axonemal microtubule arrangement ( Figure 11.1 ).
The Transition Zone
The cilium is not a simple extension of the cell membrane or cytosol, but rather it has a distinct complement of proteins necessary for its construction, signaling, and sensory functions. The specialization is established by structures located at the base of the cilium that function to separate the ciliary compartment from the rest of the cell.
At the proximal end of the cilium nearest the basal body, the cilia membrane is closely associated with the axoneme microtubules. This region is known as the transition zone . Ultrastructurally, the transition zone ( Figure 11.1c ) is comprised of a radial array of Y-shaped links, with the bottom of the “Y” attaching to each axonemal microtubule doublet and top of the “Y” likely forming attachments with the nearby cilia membrane. The molecular composition of the Y-links are unknown, however, centrosomal protein 290 (CEP290) and the protein p210 were shown to localize to the distal end of the basal body in the area of the transition zone Y-links in Chlamydomonas. The transition zone appears to be an important domain, as many proteins associated with Meckel-Gruber Syndrome (MKS, OMIM# 249000) and Nephronophthisis (NPHP, OMIM# 256100) function as part of genetically and biochemically interacting complexes that also localize in this region. In C. elegans , mutations disrupting the interactions between the transition zone proteins involved in NPHP and MKS cause the loss of Y-links, detachment of the ciliary membrane from the axoneme, abnormalities in regulating protein entry into the cilium, and defects in cilia positioning, orientation, and assembly. It is not currently known whether the MKS and NPHP proteins are a direct component of the Y-links or whether the loss of the Y-links in nphp and mks mutants is a secondary consequence.
The Ciliary Necklace
At the position where the Y-links attach to the inside of the ciliary membrane, the outside of the membrane is decorated with electron-dense proteinaceous nodules. These decorations are collectively referred to as the “ ciliary necklace ” ( Figure 11.1d ; denoted “CN”). When observed by electron microscopy, the ciliary necklace appears to restrict around the axoneme, and somewhat resemble tight junctions in epithelial cells. As such, the ciliary necklace, along with the Y-links, may function as a barrier machinery that is selective for ciliary transmembrane and membrane associated proteins. The composition of the ciliary necklace remains to be defined, and the mechanisms by which this region may function as ciliary barrier is a major focus of ongoing studies.
Transition Fibers
Through electron microscopy studies, it is apparent that there are electron-dense fiber-like structures extending from the distal end of the basal body that tether the basal body to the base of the ciliary membrane. These structures are known as transition fibers ( Figure 11.1 ). Nine transition zone fibers extend at an angle from the distal tip of the basal body and connect with membrane creating an elaborate pinwheel-like conformation. Space filling models reconstructed from electron micrographs suggest that the space between transition fibers could accommodate particles up to 60 nm, suggesting that this region could act as part of a ciliary gating or barrier mechanism preventing soluble cytosolic protein from entering the cilia. It has therefore been hypothesized that there must be other, active mechanisms for ciliary entry that are localized to this region. Data obtained from studies in C. elegans suggest that the transition fibers are docking sites for the IFT particles (see section “Cilia Transport and Trafficking”), and that the transition fibers function in loading and unloading of ciliary cargo onto the IFT particles for transport. Relatively few transition fiber proteins have been identified. One candidate is Cep164, which localizes in the region of the transition fibers and is necessary for ciliogenesis.
Cilia Membrane
The cilium has a distinct composition with regards to both protein and lipid content. Identifying the composition of the primary cilia in mammalian cells has been technically challenging, as the surface area of the cilia membrane comprises a small fraction of the total cell membrane. Initial analyses indicate that the cilia membrane contains a high concentration of sterols relative to phosphinostitides when compared to plasma membrane. The high levels of sterols in cilia have led some to speculate that cilia are enriched in lipid rafts, which also have high sterol content. In fact, several ciliary and cystic kidney disease proteins, such as cystin, associate with lipid raft components, and inhibiting this association can impair their transport to the cilium.
Information regarding the protein composition of the cilium has been obtained through proteomic and genetic studies in model systems, as well as comparing the genomes of ciliated and non-ciliated organisms. Although proteomic analysis has been performed on motile cilia and photoreceptor cilia isolated from mammalian cells, currently the most complete information regarding the protein composition of the cilium/flagellum come from Chlamydomonas . This is in part due to the ease with which deflagellation can be induced in this organism, allowing for purification and proteomic analyses.
Proteomic data revealed that the cilium contains a large number of transmembrane and membrane associated proteins that in many cases are not found in other regions of the cell. These proteins must be specifically targeted to the cilium (see section “Cilia Transport and Trafficking”). The localization of proteins in the cilia membrane is necessary for normal cilia mediated sensory functions and signaling activities (see section “Cilia Signaling in the Nephron”). This has important implications in common renal disorders, since transmembrane proteins, such as PC-1 and PC-2 localize in the cilium (see Chapter 81 for a detailed description). Other cystic kidney disease transmembrane proteins found in the cilium include fibrocystin/polyductin, and the Meckel–Gruber syndrome proteins 2 and 3 (MKS2 and MKS3 respectively) that localize to the transition zone region (see section “Transition Fibers”).
Cilia Transport and Trafficking
For the cilium to function as a sensory and signaling center, it requires the specific targeting and transport of proteins into and out of the cilium. Although the mechanism of ciliary targeting and protein transport remain poorly understood, great advances have come from seminal studies in model organisms and human ciliopathy patients. We now know many of the core components of the cilia trafficking machinery. Machinery involved in cilia trafficking include the microtubule motor driven intraflagellar transport (IFT) proteins originally defined in Chlamydomonas , and the BBSome, a complex of proteins disrupted in Bardet–Biedl syndrome (BBS, OMIM# 209900), that function in cilia membrane protein trafficking and vesicular transport. It has become evident that ciliary trafficking machinery often utilizes canonical endocytic pathways involving the Rab-family of proteins (reviewed in ). Understanding how ciliary trafficking falls into conventional trafficking modules is the focus of many ongoing studies.
Intraflagellar Transport (IFT) and IFT Motors
The axoneme provides the cilium structure, and also serves an important role in building the organelle and trafficking proteins through the cilium. Axonemal microtubules are used for trafficking components in both anterograde (toward the cilia tip) and retrograde (toward the basal body) directions in a process known as intraflagellar transport (IFT). Coordinated, bidirectional IFT movement is localized between the outer doublet microtubules and the ciliary membrane.
IFT is required to build and maintain the primary cilium through the movement of two interacting macromolecule complexes, IFT-A and IFT-B (summarized in Table 11.2 and reviewed in ). IFT-A and IFT-B interact to form a large IFT-A/B particle chain that moves together in both anterograde and retrograde IFT. It has been documented that mutations in IFT complex proteins can cause human ciliopathies. Both Jeune Asphixiating Thoracic Dystrophy (ATD, OMIM# 208500) and Sensenbrenner syndrome (CED, OMIM# 218330) are caused by mutations in IFT genes ( Tables 11.1 and 11.2 , and see sections “Jeune Asphyxiating Thoracic Dystrophy (ATD)” and “Sensenbrenner Syndrome (Cranionectodermal Dysplasia, CED).” In general, the mutations in IFT proteins identified in human patients are thus far hypomorphic, suggesting that complete loss of IFT function is not viable.
In addition to the proteins that make up IFT complexes A and B proper, there is emerging evidence for IFT associated proteins that are important for ciliary protein trafficking and ciliary function. For example, IFT20 is associated with trafficking of proteins from the Golgi to the cilia base. Also, IFT27 (Rabl4) and IFTA-2 (Rabl5) are both Rab-like proteins that associate with the IFT-A/B complex. The function of these proteins is poorly understood; however, in the absence of Rabl5 in C. elegans , cilia regulated signaling pathways are affected even though Rabl5 is not required for ciliogenesis. Thus, the current hypothesis is that Rabls5 and other IFT associated proteins may function to connect specific cargo with the IFT complex or in more subtle regulation of cilia signaling and sensory activities.
Specific motor proteins mediate IFT bidirectional movement in the cilium ( Table 11.2 ). Kinesin-2 associates with the IFT-A/B complex to move the IFT particle in the anterograde direction toward the cilia distal segment. A second kinesin, Kif17, specifically associates with the IFT-B complex, and appears to function largely in movement of the IFT-A/B particle along the distal segment of the cilium. The retrograde motor, cytoplasmic dynein-2, mediates movement of the IFT-A/B particle with its associated cargo in the retrograde direction toward the basal body. It should be noted that most of the data concerning motor-microtubule specificity is largely based on studies using homologs of cilia motor proteins in C. elegans and Chlamydomonas .
IFT builds and maintains its primary cilium by shuttling tubulin subunits to the growing (+) end of the axoneme microtubules at the ciliary tip by anterograde IFT, while cilia microtubles are cleared from the cilia by retrograde IFT. This can be demonstrated both in vivo and in vitro , where mutations in Kinesin-2 proteins (anterograde motor) lead to the absence of cilia, while mutations in cytoplasmic dynein-2 (retrograde motor) lead to bulbous, stumpy cilia caused by an accumulation of IFT particles and other ciliary proteins.
Mechanisms of Cilia Protein Entry
The correct function of the primary cilium is dependent on the localization or enrichment of receptors, channels, and effectors in this specialized compartment. As such, the mechanisms restricting or facilitating protein entry into the cilium remain an area of intense research.
A ciliary barrier or gate, which is likely found at the base of the cilium, could function to restrict entry of non-ciliary proteins and/or impair exit of proteins from the cilium. As indicated above, one of the current models is that the transition zone, transition fibers, and ciliary necklace constitute the barrier machinery ( Figure 11.1 ). Evidence supporting a diffusion barrier at the base of the cilium comes from studies using a glycosylphosphatidylinositol-anchored fluorescent protein that is targeted to the apical plasma membrane. This protein diffuses rapidly across the apical surface, but is restricted from the cilium and a region around the base of the cilium called the periciliary membrane domain . Similarly, other membrane proteins localized to the apical plasma membrane are not found in the cilium.
Although there is evidence for a cilia barrier, there are also data indicating that cilia access may in part be determined by protein size and governed by diffusion. GFP is a 27 kDal protein that rapidly equilibrates between the cilium and cytosol. However, larger proteins do not accumulate freely in the cilium, and are dependent on IFT. This has led to the hypothesis that the ciliary barrier may be analogous to nuclear pore complex. Passage through the nuclear pore involves the association of a protein containing a nuclear localization signal (NLS) binding to an importin-family protein for movement into the nucleus catalyzed by Ran GTPase activity. Intriguingly, importin-β2 has been localized to the basal body/transition zone in cultured cells, and knockdown of importin-β2 inhibits cilia localization of retinitis pigmentosa 2. Likewise, Ran is found in the cilium, and experiments using mutated Ran have shown that ciliary entry is mediated through a Ran-GTP gradient. The question remains as to whether there are specific cilia localization signals (CLS) similar to the putative NLS sequence. Indeed, NLS-like sequences have been reported in cilia proteins, such the kinesin KIF17, and disruption of this NLS motif inhibits Kif17 cilia localization. A sequence, V-x-P, necessary for ciliary localization has also been identified in the transmembrane proteins PC-2 and CNGB1b, and in several G-protein coupled receptors (SSTR3, 5HT6, DRD1, Rhodopsin, and MCHR1); however, the mechanism by which this motif targets these proteins to the cilium is variable, and is most likely protein-specific. Most other cilia transmembrane proteins do not have this signature, indicating there is no universal sequence directing proteins into the cilium.
An alternate hypothesis for ciliary enrichment was proposed by Hu et al. and involves inhibition of lateral diffusion. Ciliary proteins were highly mobile within the cilium, but there was limited exchange between the plasma membrane and the cilium. These data suggest a barrier-impeded diffusion. Hu et al. demonstrated that septin-2 is located around the base of the cilium, and may function as this barrier. Septins were originally identified as a family of guanosine triphosphatases with diffusion barrier function. Knockdown of septin-2 in embryonic fibroblasts and renal epithelium resulted in a marked increase in diffusion and exchange between cell and ciliary membranes, defects in ciliogenesis, and inhibition of cilia dependent signaling (e.g., Sonic Hedgehog (Shh) pathway, see section “Renal Cilia and Hedgehog Signaling”). Thus, one possible mechanism is that ciliary transmembrane proteins are continually transported into the cilium and accumulate there as a consequence of slow outward diffusion.
Recently, another model for ciliary membrane protein entry was proposed from the laboratory of Ira Mellman. Francis et al. analyzed gp135/podocalyxin, which is a transmembrane protein localized to the apical surface of renal epithelial cells that is also restricted from the cilium and the periciliary membrane region around the cilia base. gp135 is anchored to the cortical actin cytoskeleton through its PDZ domain. When this motif was disrupted, gp135 was now present ectopically in the cilium. Conversely, proteins that normally enter the cilia could be inhibited from doing so if the gp135 PDZ domain was incorporated into the protein. These data suggested that ciliary exclusion was dependent on attachment of membrane proteins to the cytoskeletal network, rather than the presence of a diffusion barrier. This model is hard to reconcile with the septin-2 data from Hu et al., the evidence for the BBSome in cilia membrane trafficking of specific GPCRs (see section “Ciliary Targeting and the BBSome” and ), and the presence of multiple membrane proteins that are freely diffusible and have not been detected in the cilium. Further, lipid content in the cilium has been reported to be distinct from that of cell membrane (reviewed in ). In the absence of a barrier, one would expect an equilibration between the two compartments.
Vesicular Trafficking of Transmembrane Proteins to the Cilium
Transmembrane proteins destined for the cilia are dependent on the activity of Rab-GTPases. One of the initial Rabs shown to be involved in ciliary targeting was Rab8. Inhibition of Rab8 leads to accumulation of vesicles at the base of the cilium, while Rab8 constitutive activation causes elongated cilia. Rab8 utilizes the ciliary localized exocyst complex and activity from its guanine nucleotide exchange factor (GEF) Rabin8 to dock and fuse vesicles at the cilia base. Although the process of targeting transmembrane proteins to the ciliary base is still being fully deduced, one of the most widely accepted models involves polarized exocytosis through a Rab11-associated trafficking pathway. Through this pathway, transmembrane proteins are shuttled from a Rab11 positive compartment to a Rab8 positive vesicle, dependent on Rab11 activation of Rabin8. Transmembrane proteins in these Rab8 positive vesicles are then transported to the cilia base, and inserted directly into the periciliary membrane domain. Importantly, although some vesicular structures have been reported in olfactory and chondrocyte cilia, vesicles are not commonly seen in most other cilia or flagella . These data indicate that if polarized exocytosis occurs, the fusion of the vesicles with the cilia membrane likely occurs at the base. In support of this are ultrastructural studies showing numerous vesicles containing putative ciliary-targeted proteins located around the base of cilia and flagella actively fusing with the membrane. How these proteins then get across a ciliary barrier, if it exists, is unknown.
It is also possible that the initial targeting of ciliary transmembrane proteins is to the plasma membrane. The ciliary protein would then translocate into the cilium by either lateral diffusion or through an endocytic pathway. This is best understood in the case of Smoothened (Smo), the transmembrane effector protein of the Sonic hedgehog-signaling pathway (Shh). In response to Shh stimulation, Smo translocates from the cell membrane into the cilium. This translocation is not inhibited by expression of a dominant negative form of dynamin, a GTPase required for scission of vesicles during endocytosis in eukaryotic cells. Thus, the data indicate that at least in this case, ciliary targeting is likely mediated through lateral diffusion, and does not require endocytosis of vesicles from the plasma membrane.
Ciliary Targeting and the BBSome
The BBSome is a protein complex involved in trafficking of specific cilia membrane proteins. Mutations affecting this complex of proteins cause Bardet–Biedl Syndrome (BBS). BBS is a rare human ciliopathy characterized by obesity, sensory defects, and cystic kidney disease (see section “Bardet-Biedl Syndrom (BBS)). The assembled BBSome shares structural similarities with the COP/clatherin family of coat proteins, suggesting the BBSome functions as a coat for ciliary cargos. The BBSome coat associates with the GTPase Arl6 (also known as BBS3) when Arl6 is in its GTP–bound, active state. The BBSome/Arl6 complex recognizes the ciliary localization sequence (CLS) in proteins such as SSTR3, and then escorts the protein into the cilium. The BBSome may also function for removal of proteins from the cilium. This is evidenced by the abnormal accumulation of specific transmembrane proteins in the cilium of bbs mutant mice.
Ciliogenesis
Cilia are complex structures that require continual maintenance and must be dissembled and subsequently reassembled at the beginning and end of each cell cycle. The process of building a cilium (ciliogenesis) requires the coordination of centriolar modification/maturation to form a basal body, the motor driven process of IFT to build the cilia axoneme, and carefully timed transcriptional events. These processes are synchronized with cell cycle progression, as cilia are assembled on quiescent cells. Likewise, during mitosis the centrioles must be released from their position below the cilia, and migrate to opposite spindle poles for proper cell division. Ciliogenesis can also be regulated by other factors, such as cell confluence, fluid flow, mechanical stimuli, and injury.
Transcriptional Control of Ciliogenesis
Cilia are extremely dynamic structures that grow and reabsorb depending on physiological conditions. Ciliogenesis is in part regulated through transcriptional control. This is demonstrated in Chlamydomonas , where experimentally induced deflagellation results in a large increase in the expression of flagellar genes, including the IFT proteins. In addition, in C. elegans and mammalian systems, transcription factors of the RFX (regulatory factor X) family have been shown to coordinately control expression of several IFT genes through a motif called the X-box located in their promoters. This process is evolutionarily conserved, and was first reported in C. elegans. The RFX transcription factor DAF-19 was needed for expression of several IFT proteins, as well as a few proteins known to be involved in human syndromes with cystic kidney disease phenotypes. This includes transition zone proteins such as the NPHP genes nphp-1 and nphp-4 , and the MKS genes mks-1 , b9d1 ( mksr1 ), and b9d2 ( mksr2 ), as well as multiple BBS genes including bbs-1 , bbs-2 , bbs-5 , bbs-8 , and bbs-9 (reviewed in ).
Cilia and the Cell Cycle
The basal body (see section “Centrosomes and Basal Bodies”) is generated from the centrioles that are also components of mitotic machinery. Therefore, cell division, cell differentiation, and ciliogenesis are inherently linked. As cells exit mitosis, ciliogenesis is initiated in the G 1 or G 0 phase of the cell cycle. This can be modeled in vitro , as cells will become increasingly ciliated as they reach confluence. Additionally, ciliogenesis can be induced precociously in subconfluent cells when cultured in serum-free medium. These data indicate that cilia formation and mitosis are intertwined, and has led to the proposal that the presence of the cilium may act as a G 0 checkpoint and thus as a tumor suppressor. In addition, rapid or aberrantly dividing cells, such as some tumor cells, frequently do not have a cilium. Despite this connection, increased tumor rates are uncommon in human ciliopathy patients or mouse cilia mutants. An exception to this was recently shown in the skin of cilia mutant mouse models in tumors associated with Shh signaling, where cilia have a direct role in pathway regulation.
As cells re-enter the cell cycle, cilia begin to be dismantled. This usually occurs by the G2 phase. Deconstruction of the cilium can occur by either cilia severing or resorbtion. These mechanism(s) regulating deciliation are still poorly understood. Work from Chlamydomonas suggests that katanin, a microtubule-severing protein, cleaves microtubules at the junction between the axoneme and the basal body to facilitate cilia release. In Chlamydomonas , the kinase activity of Nek8 (NPHP9) is required for deflagellation. In mammalian systems, HEF1 (human enhancer of filamentation 1) in association with Aurora A kinase and the tubule deacetylase (HDAC6) reduce axoneme microtubule stability and induce deciliation.
Cilia Extension
At the initiation of ciliogenesis, the centriole migrates towards the cell surface and proteins required for ciliogenesis, including the IFT proteins, begin to aggregate at a preciliary patch . There appear to be at least two mechanisms in which cilia initially form. The first method requires the formation of a ciliary vesicle (CV), a Golgi-derived structure that forms at the distal tip of the mother centriole. As the CV- mother centriole nears the cell surface, the cilium axoneme extends into the CV. The CV fuses with the plasma membrane, allowing the cilium to extend from the cell surface and emerge into the extracellular space. As a consequence, the basal body and the cilium remain in a small depression in the cell called the ciliary pocket . The functional importance of the pocket is not certain, but it closely resembles the flagella pocket present in some protists. In these organisms, the pocket serves as a critical sight for vesicular trafficking and endo- and exocytic activity. In the second method, the cell forgoes forming a CV, and the centrosome complex migrates to the cell surface. It is at the cell surface that the mother centriole recruits materials to begin forming the cilia from plasma membrane components.
Post Ciliogenesis Growth Control
Cilia length is an actively controlled process, and is important for normal function (reviewed in ). In motile cilia, the increase in cilia length alters beating frequency and pattern, and thus disrupts fluid movement. In primary cilia, it is less clear how changes in length may influence many of the signaling activities. Normal control of cilia length is important, as both excessively short or elongated cilia in the kidney have been shown to cause cysts.
Cilia length control is best understood from studies in Chlamydomonas , where genetic mutants have been isolated that produce either short or long flagella. Several genes involved in this process have been identified and encode kinases, such as cyclin-dependant kinase, and a mitogen-activated protein kinase (MAPK) member. The homologs for these proteins are present in mammals, suggesting evolutionarily conserved pathways; however, the targets of the kinases are not known.
One of the initial functions assigned to primary cilia in the kidney and several other tissues is that of a mechanosensor (see section “Renal Cilia and Mechanosensation”). Thus, cilia length could greatly influence the response or sensitivity of the cell to mechanical stimuli. As in some ciliated protists, mammalian cells are also able to modulate cilia length. Recent studies have revealed that decreased Ca 2+ or increased levels of cAMP can cause a marked increase in cilia length. This length increase is in part dependent on protein kinase A (PKA), and is associated with increased rate of anterograde IFT. Shear stress across the surface of many cell types is able to reduce cilia length. This was also shown to decrease cAMP levels. Importantly, this adaptive response to shear stress was not observed in cells that lack the polycystins. Thus, it has been proposed that dynamic regulation of cilia length is an important regulatory process that controls sensitivity to extracellular stimuli, and that in PKD this has been disconnected.
In addition, cilia length in the kidney, pancreas, and other tissues can be influenced by injury. Since renal injury causes rapid cyst formation in the inducible adult cilia mutant mice but not their controls, it is tempting to speculate that the cilium may have a function in regulating a pathway involved in a tissue repair process.
Other studies have revealed that cilia length can be influenced by changes in the actin and microtubule cytoskeleton. In a genomic screen for modulators of cilia length, Gleeson and colleagues identified numerous genes required for ciliogenesis, as well as for the maintenance of cilia length. Among these were genes involved in actin dynamics and organization. This included ARP3, a protein necessary for actin polymerization at filament branches, that when knocked-down caused cilia elongation. This effect was further demonstrated using the actin polymerization inhibitor cytochalasin D. These effects on actin are believed to interrupt vesicular docking and stabilization of the pericilary membrane domain at the ciliary base. Further, Sharma et al. demonstrated that actin disruption leads to a concurrent increase in the levels of soluble tubulin that can now be incorporated into the elongating ciliary axoneme. This cilia elongation was inhibited by pretreatment with the microtubule-stabilizing drug paclitaxel. This phenomenon is further supported by data showing that low levels of nocodazole, a microtubule-depolymerizing agent, could recapitulate the effects observed with actin destabilization.
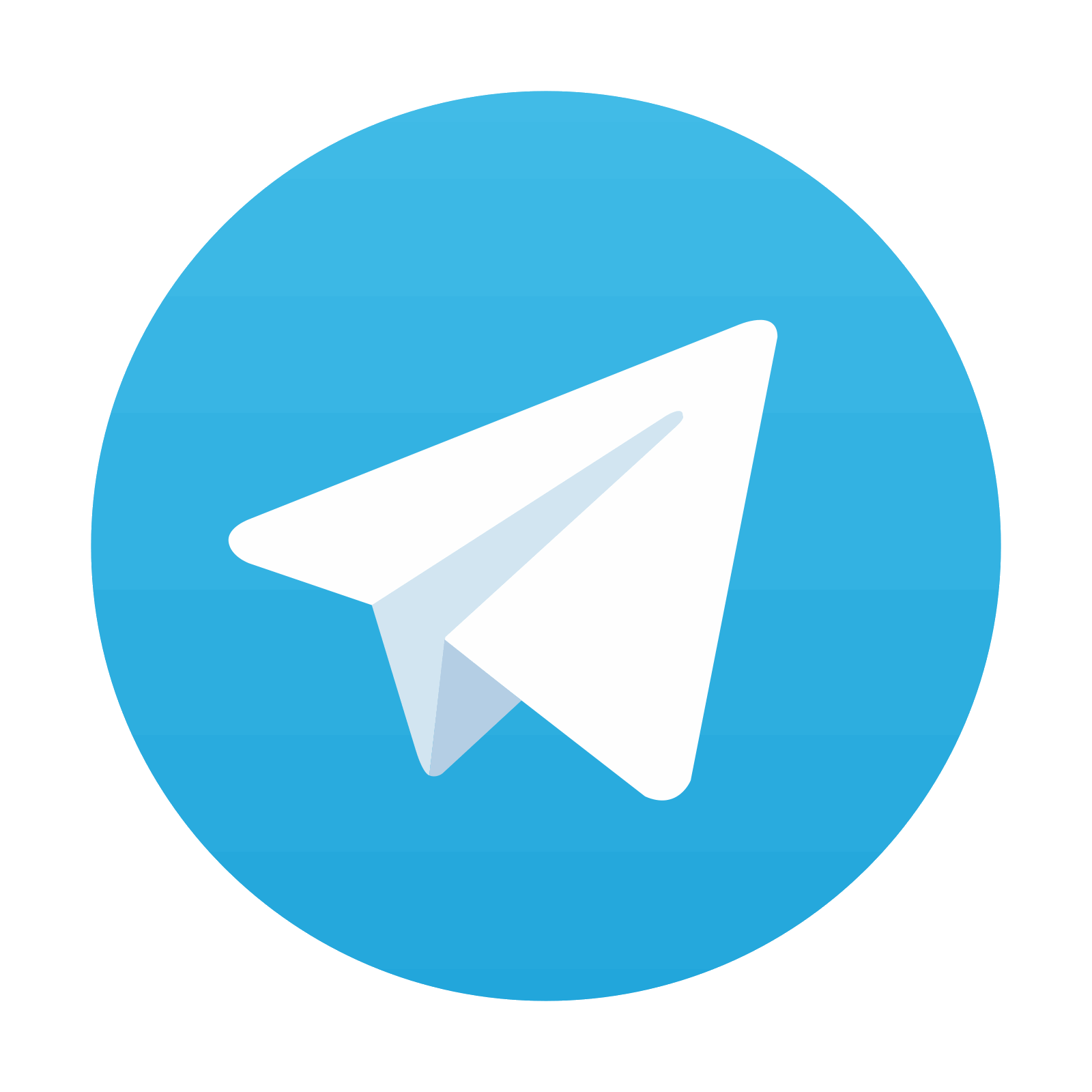
Stay updated, free articles. Join our Telegram channel
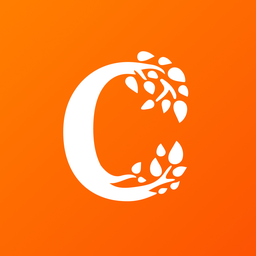
Full access? Get Clinical Tree
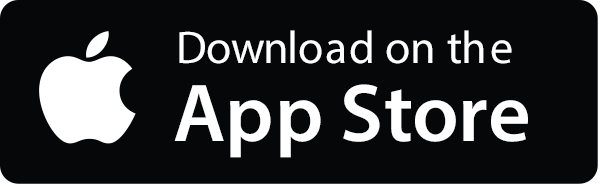
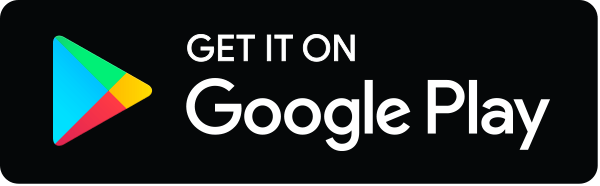