Hypernatremia can occur with normal, increased or decreased total body sodium content. In healthy individuals and in normal conditions, the plasma concentration of sodium ranges between 136 and 143 mEq/l of plasma, despite large individual variations in the intake of salt and water. The concentration is maintained at constant levels because of the homeostatic mechanism in the body. Claude Bernard was the first to appreciate that higher animals: “have really two environments: a milieu exterieur in which the organism is situated, and a milieu interieur in which the tissue elements live.” The latter is the extracellular fluid (ECF) that bathes the cells of the body. Maintenance of this consistency of plasma sodium and solute activity is the function of the thirst–neurohypophyseal–renal axis. Thirst and urinary concentration are the main defenses against hyperosmolality, and hence hypernatremia. Hypernatremia is a relatively common problem, with prevalence in hospitalized patients of 0.5 to 2%. It is defined as plasma Na + concentration ([Na + ]) greater than 145 mEq/l. It can be produced by the administration of hypertonic sodium solutions or in almost all cases, by the loss of free water. Since [Na + ] is an effective osmole, the increase in the plasma osmolality (P osm ) induced by hypernatremia creates an osmotic gradient that results in water movement out of the cells into the ECF. It is this cellular dehydration, particularly in the brain, that is primarily responsible for the neurologic symptoms associated with hypernatremia. A similar syndrome can be produced when the plasma osmolality is elevated by hyperglycemia. However, when hyperosmolality is due to the accumulation of cell-permeable solute, such as urea or ethanol, there is no water shift in the steady-state because osmotic equilibrium is reached by solute entering the cell.
Hypernatremia can occur with normal, increased or decreased total body sodium content. In healthy individuals and in normal conditions, the plasma concentration of sodium ranges between 136 and 143 mEq/l of plasma, despite large individual variations in the intake of salt and water. The concentration is maintained at constant levels because of the homeostatic mechanism in the body. Claude Bernard was the first to appreciate that higher animals: “have really two environments: a milieu exterieur in which the organism is situated, and a milieu interieur in which the tissue elements live.” The latter is the extracellular fluid (ECF) that bathes the cells of the body. Maintenance of this consistency of plasma sodium and solute activity is the function of the thirst–neurohypophyseal–renal axis. Thirst and urinary concentration are the main defenses against hyperosmolality, and hence hypernatremia. Hypernatremia is a relatively common problem, with prevalence in hospitalized patients of 0.5 to 2%. It is defined as plasma Na + concentration ([Na + ]) greater than 145 mEq/l. It can be produced by the administration of hypertonic sodium solutions or in almost all cases, by the loss of free water. Since [Na + ] is an effective osmole, the increase in the plasma osmolality (P osm ) induced by hypernatremia creates an osmotic gradient that results in water movement out of the cells into the ECF. It is this cellular dehydration, particularly in the brain, that is primarily responsible for the neurologic symptoms associated with hypernatremia. A similar syndrome can be produced when the plasma osmolality is elevated by hyperglycemia. However, when hyperosmolality is due to the accumulation of cell-permeable solute, such as urea or ethanol, there is no water shift in the steady-state because osmotic equilibrium is reached by solute entering the cell.
Regulation of Water Homeostasis
Significance of the Plasma Sodium Concentration
The total body water (about 60% of body weight in males and 50% in females) is distributed between the intracellular fluid (ICF, 60% of body water) and extracellular fluid (ECF, 40% of body water) spaces. Flame photometry and, more recently, ion selective electrode technology have made the plasma sodium concentration one of the simplest and most frequently measured constituents of the body fluids. It is not always appreciated that a given concentration of the plasma sodium may be consistent with different functional states. The plasma sodium is simply a concentration term, and as such reflects only the relative amounts of sodium and water present in the sample. The concentration is not a measure of total body sodium content. It is determined empirically by the following relationship:
Plasma [ Na + ] = Total – body Na + + Total – body K + Total – body water
The relationship indicates the fact that hypernatremia can occur as a consequence of a decrease in total body water, an increase in total body sodium or a combination of these events. It gives no information regarding replacement or removal of sodium. When flame spectrophotometry is used to measure the amount of sodium in a plasma sample, substances such as plasma proteins, abnormally high glucose, and lipid can occupy a large fraction of the plasma volume and underestimate the actual sodium concentration. The ionic composition of the plasma is measured as milliequivalents per liter of plasma. Only about 930 ml of each liter of plasma is water. The remaining 70 ml is occupied by the plasma proteins and, to a lesser degree, lipids. In the presence of hyperlipidemia or hyperproteinemia, the plasma water content may be less than 93%.
Generation of Hypernatremia
Since Na + and its accompanying anions are the major effective ECF osmoles, hypernatremia is a state of hyperosmolality. As a result of the fixed number of ICF particles, maintenance of osmotic equilibrium in hypernatremia results in ICF volume-contraction. The increase in the plasma osmolality induced by hypernatremia creates an osmotic gradient that results in water movement out of the cells into the ECF. A similar syndrome can be produced when plasma osmolality is elevated by hyperglycemia. When hyperosmolality is due to the accumulation of a cell-permeable solute, such as urea or ethanol, there is no water shift because osmotic equilibrium is reached by solute entry into cells. Therefore, both urea and ethanol are ineffective osmoles. Plasma osmolality can be measured directly by determining freezing point depression or vapor pressure. Variable changes in the plasma sodium concentration occur with hyperglycemia. Since glucose enters cells slowly, an increase in the plasma glucose concentration raises effective plasma osmolality and causes water to move from the cells into the ECF. By dilution, this lowers the plasma Na + concentration. In theory, every 62 mg/dl increment in the plasma glucose concentration should draw enough water out of the cells to reduce the plasma Na + concentration by 1 mEq/l.
The number of particles per gram of water determines the osmolality of a solution. Since sodium salts (particularly NaCl and NaHCO 3 ), glucose, and urea are primary extracellular osmoles, the plasma osmolality can be approximated from:
Plasma osmolality ( P osm ) = 2 × Plasma [ Na + ] + [ Glucose ] 18 + BUN 2.8
where 2 reflects the osmotic contribution of the anion accompanying Na + , and 18 and 2.8 represent the conversion of the plasma glucose concentration and blood urea nitrogen (BUN) from units of milligrams per deciliter (mg/dl) into millimoles per liter (mmol/l).
Although urea contributes to the absolute value of the P osm , it does not act to hold water within the extracellular space because of its membrane permeability. Therefore, urea is an ineffective osmole and does not contribute to the effective P osm .
In general, the effective plasma osmolality can be calculated from or estimated from:
Effective plasma osmolality = Measured plasma osmolality − BUN 2.8
Effective plasma osmolality = 2 × Plasma [ Na + ] + [ Glucose ] 18
Under normal circumstances, glucose and urea contribute less than 10 mOsm/kg H 2 O, and the plasma Na + concentration is the main determinant of the plasma osmolality, the osmolality of body fluids can be estimated to be twice the plasma sodium concentration.
The major ECF particles are Na + and its accompanying anions Cl − and HCO 3 − ; a high plasma sodium concentration is always associated with a high osmolality. This indicates that water is needed to restore isotonicity. The water deficit can be estimated from the plasma sodium level. The percentage increase in sodium concentration approximates the percentage decrease in total body water. The water deficit can be estimated by the equation:
Water deficit = Total – body Water × ( Plasma [ Na + ] 140 − 1 )
Total body water varies with body size and fat content. It is approximately 60% of body weight in young men, 50% of body weight in old men and young women, and only 40% in elderly women.
Defense Mechanisms Against Water Depletion
Two primary mechanisms defend the body against water depletion and hyperosmolality of extracellular fluid space. These two defense mechanisms are the capacity of the kidney to excrete a concentrated urine, and stimulation of thirst to increase water intake. Each pathway is very effective and disturbance of the urinary concentrating mechanism alone generally does not cause hyperosmolality if the thirst mechanism is intact.
Control of ADH Secretion
Hypernatremia results in the stimulation of both the antidiuretic hormone (ADH) release and thirst by the hypothalamic osmoreceptors ( Figure 45.1 ). Argenine vasopressin is the ADH in humans. Argenine vasopressin binds to specific receptors on collecting ducts (V 2 receptors), which are coupled to cyclic AMP (cAMP) formation. The regulation of ADH release from the posterior pituitary is dependent primarily on two mechanisms: osmotic and nonosmotic pathways ( Figure 45.2 ). The osmotic regulation of ADH is dependent on osmoreceptor cells in the anterior hypothalamus. These cells, most likely by altering their volume, recognize changes in ECF osmolality. Cell volume is decreased readily by substances that are restricted to the ECF, such as hypertonic saline or hypertonic mannitol. These substances are effective in stimulating ADH release. In contrast, urea moves readily into cells, and therefore does not alter cell volume and does not effectively stimulate ADH release. A similar response pattern is evident when vasopressin release is studied in the hypothalamo–neurohypophyseal complex in organ culture. Specifically, sodium chloride, sucrose, and mannitol at 310 mOsm/kg H 2 O cause a three-fold increase in argenine vasopressin release, while urea and glucose fail to stimulate vasopressin. These studies also support the view that the receptor responds to changes in osmolality rather than sodium. The effects of increased osmolality on vasopressin release are associated with a measurable increase in vasopressin precursor messenger RNA (mRNA) in the hypothalamus and salt-loading increases vasopressin RNA in the pituitary. Vasopressin release can also occur in the absence of changes in plasma osmolality. Physical pain, emotional stress, hypoglycemia, and a decrease in blood pressure or blood volume are important nonosmotic stimuli for vasopressin release. A 7 to 10% decrement in blood pressure or blood volume causes the prompt release of vasopressin ( Figure 45.2 ). Although there are considerable genetically-determined individual variations in both the threshold and sensitivity, a close correlation between argenine vasopressin and plasma osmolality has been demonstrated in subjects with various states of hydration ( Figure 45.3 ).



The secretion of ADH generally begins when the plasma osmolality exceeds 275 to 285 mOsm/kg H 2 O. The threshold for thirst appears to be approximately 10 mOsm/kg H 2 O above that of vasopressin release. Prevention of a total body water deficit is thus largely dependent on water intake as modulated by thirst. The thirst center appears to be closely associated anatomically with the osmoreceptors in the region of the hypothalamus. Defects in thirst response may involve either organic or generalized central nervous system lesions, and can lead to severe water deficit even in the presence of a normal concentrating mechanism. The water deficit will occur more promptly if renal concentrating ability is also impaired.
Thirst and the Maintenance of Hypernatremia
Thirst is, in fact, so effective that even patients with complete diabetes insipidus avoid hypernatremia by fluid intake in excess of 10 l/day. Hypernatremia supervenes, therefore, only when hypotonic fluid losses occur in combination with a disturbance in water intake. This is most commonly seen in the aged with an alteration in level of consciousness, in the very young with inadequate access to water or in a rare subject with a primary disturbance in thirst. Prevention of a total body water deficit is thus largely dependent on water intake as modulated by thirst. The thirst center appears to be closely associated anatomically with the osmoreceptors in the region of the hypothalamus. Defects in thirst response may involve either organic or generalized central nervous system lesions, and can lead to severe water deficit even in the presence of a normal concentrating mechanism.
In summary, persistent hypernatremia does not occur in normal subjects, because the ensuing rise in plasma osmolality stimulates both the releases of ADH, thereby minimizing further water loss and, more importantly, thirst. The associated increase in water intake then lowers the plasma sodium concentration back to normal. This regulatory system is so efficient that the plasma osmolality is maintained within a range of 1% to 2%, despite wide variations in sodium and water intake. Even patients with diabetes insipidus, who often have marked polyuria due to diminished ADH effect, maintain a near-normal plasma sodium concentration by appropriately increasing water intake. The net effect is that hypernatremia primarily occurs in those patients who cannot express thirst normally: infants and adults with impaired mental status. The latter most often occurs in the elderly, who also appear to have diminished osmotic stimulation of thirst. A patient with a plasma sodium concentration of 150 mEq/l or more who is alert but not thirsty has, by definition, a hypothalamic lesion affecting the thirst center.
Cellular Response to Hypernatremia
Volume Regulation
When exposed to a change in extracellular osmolality, a cell shrinks or swells and subsequently exhibits either a regulatory volume increase or a regulatory volume decrease. When exposed to hypertonicity, a cell loses water until the intracellular and extracellular osmolality are equal. Recovery of water is mediated by accumulation of inorganic and organic solutes known as osmolytes. The principle inorganic osmolytes are Na + , K + , and Cl − . The principle organic osmolytes may be classified into three general groups: polyols; methylamines; and amino acids. A comparison of major and minor organic osmolytes for the kidney and brain are depicted in Table 45.1 .
Organic Osmolyte | Kidney | Brain |
---|---|---|
Major | Glutamate | Glutamate |
Glutamine | Glutamine | |
Taurine | Taurine | |
Myoinositol | Myoinositol | |
Urea | Urea | |
Alanine | Alanine | |
Sorbitol | Aspartate | |
GPC | Glycine | |
Betaine | GABA | |
Minor | Val/Leu/Isoleu | Theonine |
Phosphocreatine | GPC | |
Creatine | Betaine | |
Choline | ||
Phosphocreatine | ||
Lysine | ||
Serine |
While hypernatremia in mammals affects all tissues, the greatest potential for harm is to the brain and kidney. Of these two organs, even modest changes in serum osmolality can have severe consequences to the brain, resulting in volume changes. In the brain, acute hypernatremia is associated with a rapid decrease in water content and a corresponding increase in solute concentration. In a study of rats by Cserr and co-workers, acute hypernatremia (plasma Na + =180 mEq/l) was accompanied by a prompt decrease (7%) in total brain water content. The fall in water content was less than expected for simple osmotic behavior, indicating significant volume regulation had occurred. Moreover, the decrease in total brain water was the result of a fall in extracellular volume. Intracellular volume was not significantly changed at 30 and 90 minutes. The rapid regulatory volume increase was mediated by increases in Na + , K + , and Cl − . Most of these ions were derived from bulk flow of NaCl from cerebral spinal fluid (CSF). There was also a lesser contribution of electrolytes from the blood. During experimental hypernatremia in rabbits, intracellular brain water content also decreased by 12% at 1 hour and by 17% at 4 hours, accompanied by a corresponding increase in intracellular osmolality. Most of the acute increase in brain osmolality was due to an increase in intracellular sodium and potassium concentration. Although a substantial component of the increase in electrolyte concentration resulted from transcellular water loss, whole brain electrolyte content also increased, with the sodium content increasing from 268±9 mm/kg dry weight in control animals to 321±19 mmol/kg dry weight after 4 hours of hypernatremia. After sustained hypernatremia of 7 days duration, brain water and volume values were restored to normal. More prolonged hypernatremia results in accumulation of organic osmolytes to restore total brain water content to normal levels.
In the kidney, the general mechanism for cellular osmoregulation in the face of hypertonic conditions involves the accumulation of organic rather than inorganic osmolytes. This strategy for the transport of organic rather than inorganic osmolytes across the cellular membrane has been explained in at least three hypothesized mechanisms, as detailed in Table 45.2 . However, before mounting a coordinated osmoresponse, cells experience a “molecular mayhem” due to the initial decrease in cellular volume, resulting in crowding of macromolecules and an increase in ionic osmolytes. These early changes in the cellular status, as well as the loss of water which can affect a variety of biochemical processes, can result in a variety of deleterious effects on normal cellular functions and viability, as depicted in Table 45.3 .
Name | Details | Reference |
---|---|---|
Compatible osmolyte principle | Cells accumulate high levels of polyols or certain amino acids that do not affect protein function in contrast to NaCl or KCl | |
Counteracting osmolyte principle | Cells accumulate methylamines (i.e., GPC or betaine) to attenuate the destabilizing effect of urea on protein structure | |
Constant transmembrane gradient | Cells maintain constant transmembrane gradients for sodium and potassium, thus the driving force for sodium gradient-coupled transport systems for organic and inorganic solutes |
General Function | Reference |
---|---|
DNA: Damage, inhibition of repair, dissociation of protein from chromatin, induction of p53 | |
Metabolism, cell growth: Disruption of mitochondria function, cell cycle arrest, growth factor-dependent signaling inhibition, alteration in cytoskeletal structure, inhibition of protein translation | |
Secondary stress, apoptosis: Induction of secondary oxidative stress, apoptosis |
Osmolytes
Organic molecules serve an important biologic function in the process of cellular osmoregulation. When extracellular osmolality increases, organic molecules accumulate in the cells, thus maintaining cell volume and counteracting the perturbation of enzyme function and protein structure by high concentrations of inorganic ions and other molecules such as urea. Lien and his co-workers studied the effect of varying degrees and duration of hypernatremia on the concentrations of substances believed to be important idiogenic brain osmoles in rats using conventional biochemical assays, nuclear magnetic resonance spectroscopy, and high performance liquid chromatography. Idiogenic osmoles have been postulated to develop in the brain cells of patients suffering from chronic elevations in the osmolality of ECF. Moreover, the rapid correction of the osmolality in such patients is associated with the development of cerebral edema.
It has been known for more than 35 years that changes in intracellular brain sodium and potassium concentrations cannot account for all of the observed changes in brain osmolality that occur during chronic exposure to extracellular hypernatremia. The solutes that develop to maintain equality between extracellular and intracellular brain osmolality during this adaptation to hyperosmotic stress have been investigated by several groups. Arieff and his associates have demonstrated that osmoles accumulated in the brain of chronic hypernatremic, but not in acute hypernatremic, rabbits. Other investigators have shown that the level of amino acids and their derivatives, such as glutamine, taurine, and urea, rise in the brain of chronic hypernatremic animals, and account for about half of the increment of brain osmoles. However, many of the idiogenic osmoles have not yet been characterized in brain tissue. Polyols and trimethylamines accumulate intracellularly in marine animals, plants, and bacteria when extracellular or environmental osmolality is significantly increased. In this study, it was found that the inorganic osmolytes account for 50% to 60% of the increase in solute content, whereas organic osmolytes account for the remainder during adaptation to chronic hypernatremia ( Figure 45.4 ).

Recovery from chronic hypernatremia involves a small transient rise in brain water, which is restored to normal within 48 hours. With the exception of myoinositol, all the electrolytes and organic osmoles fall to normal levels within 24 to 48 hours. The cellular mechanisms responsible for the loss of intracellular organic osmolytes are poorly understood. In vitro studies of brain tissue found that cell swelling causes rapid loss of electrolytes and organic osmolytes. The losses of cellular K + and Cl − are likely mediated by swelling-activated ion channels. Characterization of the organic osmolyte efflux pathways suggests that they represent pores or channels in the membrane that are permeable to Cl − , as well as small organic salts. Roy and Banderali described a chloride channel that can transport small organic solutes, such as taurine and amino acids. No specific inhibitors of the pathways are known. However, inhibitors of arachidonic acid metabolism are able to prevent activation of the efflux pathway, implicating eicosanoids in the signal transduction mechanism.
Lien et al. reviewed previous studies to estimate the percentage of the osmolality that is still idiogenic. The contributions of electrolytes and other solutes to the changes in brain osmolality for various durations and degrees of hypernatremia were reported in different studies ( Table 45.4 ).
Contribution to Osmolality Change | |||||||
---|---|---|---|---|---|---|---|
Duration | Animal Species | Changes of Osmolality (mmol/kg) | Na + | K + | Cl + | Other Solutes | Reference |
Acute hypernatremia (hours) | |||||||
1 | Rat | 139 | 24 | 24 | ND | Amino acids Urea | |
1 | Rabbit | 60 | 38 | 32 | 18 | ND | |
2 | Rat | 101 | ND | ND | ND | Urea | |
3 | Rat | 117 | 30 | 19 | 30 | ND | |
4 | Rat | 55 | ND | ND | ND | Amino acids | |
4 | Rabbit | 96 | 21 | 26 | 24 | Amino acids | |
9 | Rabbit | 118 | 29 | 19 | 25 | ND | |
Chronic hypernatremia (days) | |||||||
3 | Rat | 74 | ND | ND | ND | Polyols | |
4 | Rat | 83 | ND | ND | ND | Amino acids | |
4 | Mouse | 80 | 19 | 26 | ND | Amino acids Urea | |
5 | Rat | 50 | ND | ND | ND | Amino acids Methylamines polyols | |
7 | Rat | 80 | 25 | 1 | 25 | ND | |
7 | Rabbit | 80 | 8 | 5 | 15 | Amino acids | |
58 | 28 | 21 | 19 | ND | |||
7 | Rat | 102 | ND | ND | ND | Amino acids Methylamines Polyols urea |
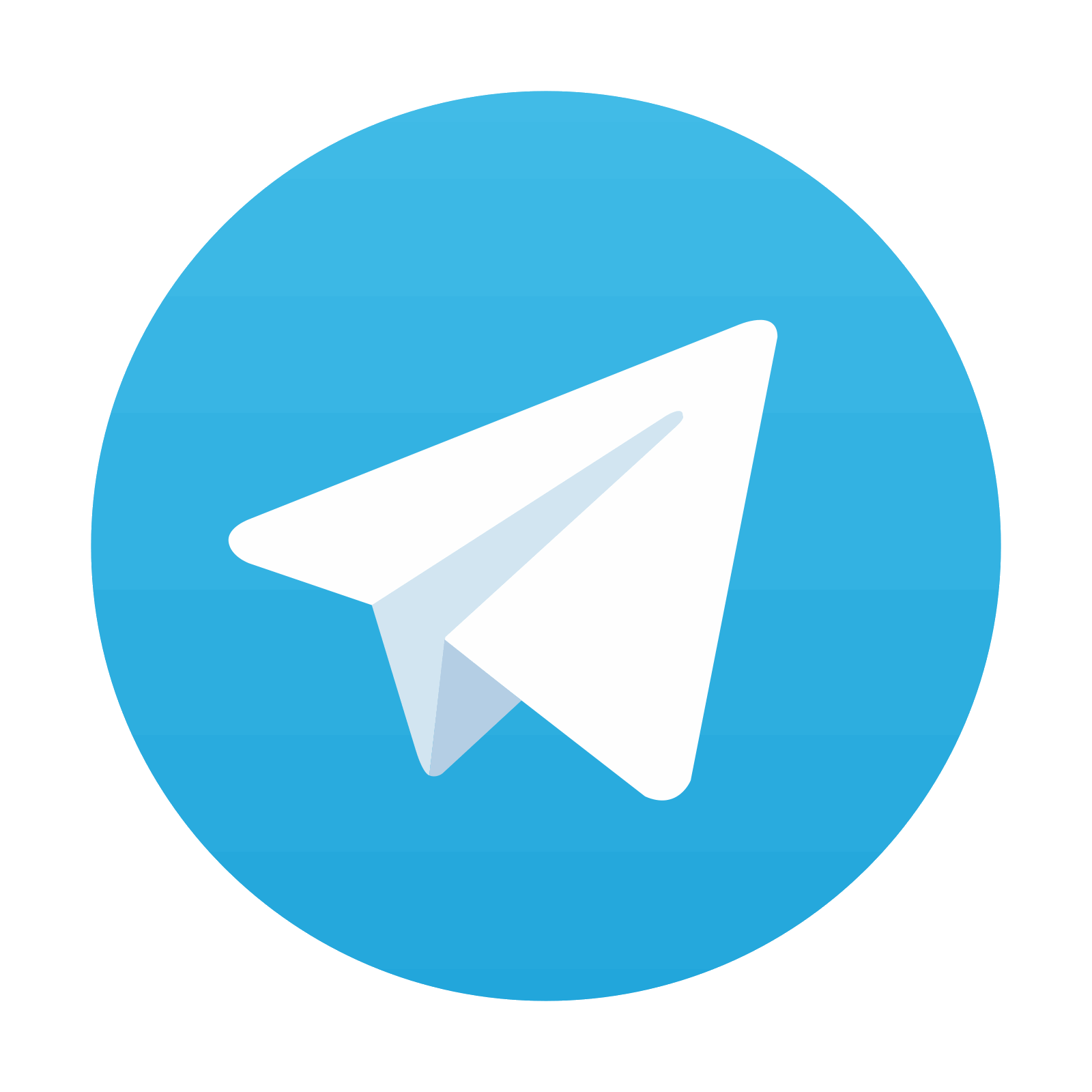
Stay updated, free articles. Join our Telegram channel
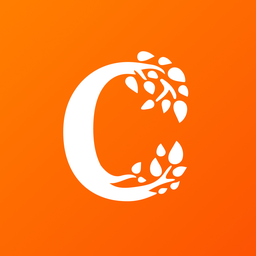
Full access? Get Clinical Tree
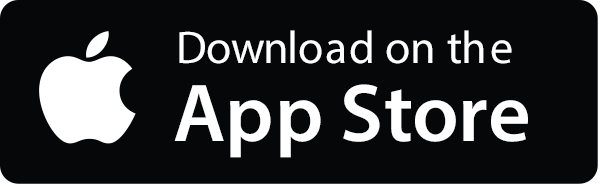
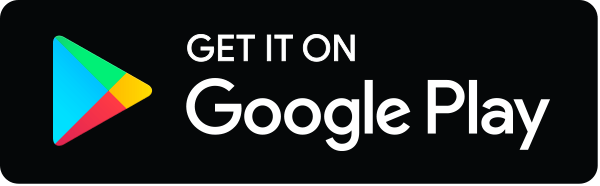
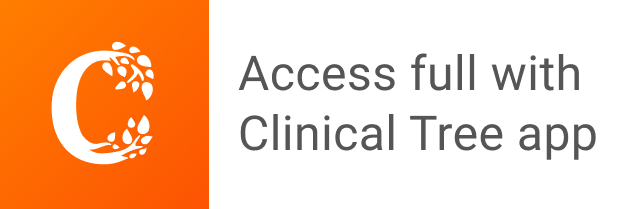