The idea that the kidney is an organ needs to be tempered by the realization that it is the nephron that is the organ. Each nephron, as far as we know, is independent from other nephrons, and the kidney is to a first approximation a collection of these mini-organs put together in a complex three-dimensional assembly. The nephrons of all mammals are remarkably similar in size, function, and origin (as far as we know), and the differences among species are largely if not entirely due to the number of these units in the assembly, with mice having 10,000 and whales having 250 million in each kidney. But while the epithelial character of the nephron garners most of the attention, one needs to be reminded that the kidney has a very extensive vascular network with multiple distinct morphological and functional domains, as well as an abundant interstitial cell population that unfortunately is still poorly understood (see Kaissiling and Le Hir for a review). While the taxonomy of the renal epithelial cells is nearly complete, that of the cells of the vascular and interstitial compartments awaits detailed characterization. Hence, analysis of renal regeneration after injury, as well as search for putative renal stem cells in the adult kidney, has essentially been restricted to the epithelial compartment. However, as epithelial cells are likely instructed by mesenchymal signals and epithelia-mesenchymal cross-talk is critical for renal epithelial differentiation and function, there is a need for deeper understanding of the cell types that comprise the renal vascular and interstitial compartments, and their roles in kidney homeostasis and repair from injury. For example, in many organs including the kidney, mesenchymal cells with characteristics of precursor/stem cells have been found to reside near or in the vascular wall, but the exact origin and normal function of these cells is unknown.
Embryonic Origin of Renal Cells
The idea that the kidney is an organ needs to be tempered by the realization that it is the nephron that is the organ. Each nephron, as far as we know, is independent from other nephrons, and the kidney is to a first approximation a collection of these mini-organs put together in a complex three-dimensional assembly. The nephrons of all mammals are remarkably similar in size, function, and origin (as far as we know), and the differences among species are largely if not entirely due to the number of these units in the assembly, with mice having 10,000 and whales having 250 million in each kidney. But while the epithelial character of the nephron garners most of the attention, one needs to be reminded that the kidney has a very extensive vascular network with multiple distinct morphological and functional domains, as well as an abundant interstitial cell population that unfortunately is still poorly understood (see Kaissiling and Le Hir for a review). While the taxonomy of the renal epithelial cells is nearly complete, that of the cells of the vascular and interstitial compartments awaits detailed characterization. Hence, analysis of renal regeneration after injury, as well as search for putative renal stem cells in the adult kidney, has essentially been restricted to the epithelial compartment. However, as epithelial cells are likely instructed by mesenchymal signals and epithelia-mesenchymal cross-talk is critical for renal epithelial differentiation and function, there is a need for deeper understanding of the cell types that comprise the renal vascular and interstitial compartments, and their roles in kidney homeostasis and repair from injury. For example, in many organs including the kidney, mesenchymal cells with characteristics of precursor/stem cells have been found to reside near or in the vascular wall, but the exact origin and normal function of these cells is unknown.
The different cellular compartments of the adult kidney have been traditionally recognized by their morphological characteristics or by their embryonic origin, since it was long ago recognized that the adult (metanephric) kidney derives from two distinct elements of the intermediate mesoderm: the metanephric mesenchyme and the ureteric bud. Within the kidney, the ureteric bud gives rise to the collecting duct cells, while some metanephric mesenchyme cells give rise to the rest of the nephron. However, over the last few decades, the discovery of several genes that are expressed in the restricted group of cells of the renal anlage has allowed a different taxonomic approach that has greatly illuminated our understanding of the distinct cell populations in the adult kidney. Moreover, it has allowed development of research tools with which it is possible to probe in the adult kidney the function of specific cells, of specific genes in specific cells, and importantly for the present discussion, to identify the daughter cells of different cell types by in vivo genetic cell lineage methods. Thus, we briefly review the embryonic origin of the distinct cells in the adult kidney, emphasizing those aspects that might clarify the origin of new cells in the adult organ.
Epithelial Cells
All epithelial cells of the adult kidney are believed to derive from the intermediate mesoderm, from which both the ureteric bud (a branch of the Wolffian duct) and the metanephric mesenchyme originate. Renal morphogenesis starts when the ureteric bud invades the metanephric mesenchyme and starts branching. The cells in the tip of each ureteric bud branch give rise to the collecting duct cells and the metanephric mesenchyme cells in contact with each ureteric bud tip give rise, after a series of morphogenic steps, to the cells of the remaining nephron segments spanning from the connecting tubule to the glomerulus. The ureteric bud, like the Wolffian duct, expresses the homeobox gene HoxB7 , and transgenic mice expressing HoxB7- GFP or HoxB7- Cre recombinase have been used to label most, if not all, of the cells in the ureteric bud branches of the embryonic collecting duct, and their progeny in the adult kidney.
The metanephric mesenchymal cells undergo simultaneous differentiation (to generate a nephron for each ureteric bud tip) and growth, so that the appropriate number of nephrons will be generated for the branches of the ureteric bud. It was recently found that the metanephric mesenchymal cells that are in contact with the tips of the ureteric bud, referred to as the cap mesenchyme, are the progenitor cells of all nephron epithelia (except the collecting duct). These cells were found to express the transcription factors Cited1 and Six2 , thereby allowing generation of transgenic mice that label all nephron epithelial cells except those of the collecting ducts. More relevant to the present discussion is that these mice can be used to permanently identify the progeny of adult nephron epithelial cells, thus providing an invaluable tool for analysis of epithelial cell regeneration after kidney injury and/or disease, as discussed below.
Mesenchymal/Stromal Cells
Like renal epithelial cells, the vast majority of the stroma cells in the adult kidney derive from the intermediate mesoderm that in the kidney gives rise to a cell population that expresses the forkhead transcription factor Foxd1 . These cells generate many renal interstitial cells, as well as mesangial cells, vascular smooth muscle, pericytes, and renal capsule, and likely mesenchymal stem cells. Foxd1 -expressing cells are absolutely required for normal kidney development, and their adult progeny is of extreme interest because it likely contains pluripotent MSC and pericytes, although a clear-cut distinction between these two cell types is not yet possible. In addition, identification of Foxd1 as marker of these cells has made it possible to develop transgenic mice that can be used to label the stroma cell progeny in the adult kidney.
Another population of renal stromal cells derives from the paraxial mesoderm, but the precise contribution of these cells to the interstitial and mesenchymal cell populations of the adult kidney remains to be defined. Finally, an area of the intermediate mesoderm located ventro–lateral to the dorsal aorta generates renal interstitial cells that express the stem cell factor receptor ( c-kit ). During embryogenesis, these cells appear to be involved in the maintenance of the metanephric mesenchyme-derived cells, but identification of their progeny in the adult kidney remains to be established.
Endothelial Cells
The renal circulation is both anatomically and functionally complex, and likely contains many types of endothelial cells. Their exact origin is still poorly-understood. It is clear that once kidney development starts, the renal anlage contains angioblats that give rise to endothelial cells. It is currently unknown whether these endothelial precursors migrate into the developing kidney or differentiated from cells that reside in the metanephric mesenchyme. The latter appears more likely, as we found that many cells in the renal anlage express tyrosine kinases that are characteristic of adult endothelial cells. Interestingly, in addition to being endothelial precursors, angioblasts in the renal anlage appear to provide signals important for development and differentiation of the metanephric mesenchyme. It is currently unknown if there exists interaction in the adult kidney between endothelial cells and either interstitial or epithelial cells that might be involved in maintaining kidney homeostasis or repair from injury. Recent work by Lin et al. suggests that endothelial-to-pericyte cross-talk is involved in the generation of kidney myofibroblats, as detailed below.
New Cells in the Adult Kidney
Normal Conditions
As assayed by a variety of methods, the normal adult kidney has a low rate of cellular proliferation. Using antibodies to Ki67, a nuclear protein expressed in cycling cells during G0 and G1, between 0.4–1% of all cells were cycling in the adult rat kidney. Interestingly, age has a profound effect in the abundance of proliferating cells found in the kidney. Vogesterder et al. found that while only ~0.4% of all renal cells were positive for Ki67 in the kidneys of 16- to 20-week-old rats, in animals that were only 4 weeks old the number of cycling cells was ~5%. This suggests that in the kidney there is an age-dependent progressive decline in the number of cycling cells, and that workers examining renal cell proliferation should take into account the age of the animal as an important variable.
Proliferating cells in the renal cortex of 4-week-old rats were found preferentially located to the S3 segment of the proximal tubule, when compared to the S1 and S2 segments. This interesting result might have important implications in designing strategies to identify renal stem cells, a subject to which we will return below. In contrast, in 1-year-old rats, we found that most of the kidney parenchyma had a homogeneous fraction of ~1% of Ki67 positive cells Figure 29.1 . There were two exceptions to this, however, the body of the papilla where there were extremely low numbers of cycling cells (<0.1% of the cells were positive for Ki67), and the upper part of the papilla (at the papilla–medullary junction), where we found the highest frequency of cycling cells (~2.5%), indicating that it is an area of privileged cellular proliferation in the adult rat. Detailed morphological observations indicate that terminally differentiated tubular epithelia cells can generate new cells, but these observations don’t exclude the possibility that there are epithelial stem cells.

Organ Repair from Injury
In contrast to normal conditions, the kidney displays a remarkable proliferation capacity shortly after transient injury. For example, injury induced by 30–45 minutes of complete renal artery occlusion in rodents causes functional failure, and widespread cellular apoptosis and necrosis that are followed by diffuse cellular proliferation and functional recovery. What is the origin of these new cells? While the kidney has multiple cell types, studies on the generation of new cells after injury have fundamentally focused on the epithelial cells, likely because of their better-understood functional importance and easier identification. It is now established that the new epithelial cells after kidney injury develop from within the parenchyma, rather than being derived from extrarenal sources such as the bone marrow, and thus three possibilities appear likely: (1) any surviving terminally differentiated epithelial cell can generate identical cells; (2) there exist kidney epithelial stem cells capable of generating any epithelial cell type, similar to what was found in the interfollicular epidermis; and (3) pluripotent renal stem cells generate epithelial as well as other cell types, as in the case of the stem cells in the bulge of the skin.
Morphological observations and functional studies with nucleotide analogs have provided strong evidence that terminally-differentiated epithelial cells generate new epithelial cells after injury. More recent elegant genetic cell fate-mapping studies have confirmed this suspicion; Humpreys et al. used reporter mice in which the renal epithelial cell compartment was labeled by a Cre recombinase driven by the promoter of Six2 , a gene that is specifically expressed in embryonic epithelial precursors (see above), and examined their response to acute kidney injury. They found that injury induced massive cellular proliferation, and that all new epithelial cells expressed the reporter gene ( Figure 29.2 ). Since RT-PCR of adult kidney tissue could neither detect Six2 nor Cre , it is apparent that the labeled cells originated from epithelial cells labeled previously during kidney development, thus excluding the interstitial/stroma cell compartment as the origin of new epithelial cells. Needless to say, this experiment does not address whether there exists a group of restricted epithelial cells that are responsible for all the new epithelia generated after injury; these cells could function as adult renal epithelial stem cells. In a recent study, Humphreys et al. examined this possibility by labeling cycling cells after transient kidney injury with two different thymidine analogs administered sequentially. Since the number of epithelial cells that were positive for both nucleotides was very low, these workers concluded that surviving epithelial cells repopulate the nephron epithelium in a stochastic manner, suggesting that the nephron epithelia has no stem cells. However, while control experiments clearly showed appropriate specificity of both antibodies, detection of closely related thymidine analogs during conditions that, unlike in the control experiments, probably result in incorporation of very different amounts of the two analogs in a given cell are fraught with potential problems. More importantly, their conclusion is based on the implicit assumption that a putative population of epithelial stem cells would be a small fraction of the total number of cells. Under these conditions, to repopulate the damaged epithelia the stem cell progeny would need to divide rapidly, and would thus incorporate both nucleotide analogs. However, as detailed below, stem cells in Drosophila Malpighian tubules are a very large fraction of the total cells, and there is no reason why this may not also be the case in mammalian kidneys. Similarly, in organs other than the kidney such as the adult airway epithelia, stem cells account for about one-third of the total number of cells.

Identification of the site where cellular proliferation first starts after transient injury could potentially facilitate identification of precursor/stem cells. Unfortunately, for most insults that cause acute kidney injury with functional failure, cellular proliferation has most often been examined one or a few days afterwards, at which time cell proliferation, while very prominent in the S3 segment of the proximal tubule, is also widespread in other parts of the kidney parenchyma, particularly the medulla.
Following acute kidney injury by renal artery occlusion we could not detect proliferating cells until ~24 hours later when we examined kidney sections of ~5 μm thickness, as is done routinely. However, with 100 μm vibrotome sections, one hour after injury we found that the upper part of the papilla had more proliferative cells than other parts of the kidney (see Figure 29.3 ), suggesting that this is the site of initial cellular proliferation after kidney injury, which we previously found to be the site of enhanced cell cycling under normal conditions (see above). Interestingly, Vinsonneau et al. reported that the first cells that they found cycling after ischemia reperfusion injury to the kidney were uro-epithelial cells in the upper part of the urinary (intrarenal) space and neighboring interstitial cells; detailed analysis with Ki67 and BrdU incorporation showed that these cells were proliferating ~16 hours after ischemic injury and ~4 hours before proliferation could be detected elsewhere in the kidney. The site identified by Vinsonneau et al. is where the base of the papilla attaches to the medulla, it is in close proximity to the cortex, and appears to be the same proliferating site we identified in the upper papilla. Needless to say, identification of these “early” proliferating cells and of their progeny would be of extreme interest.

An additional observation merits mention. In the few studies that identified the cells that first started proliferating after injury, either after transient ischemic injury or aminoglycoside toxicity, it is remarkable that in all instances they were interstitial cells, perhaps suggesting that some interstitial cell plays a critical role in initiating epithelial regeneration. Indeed, the likelihood of renal functional recovery after injury was found to correlate with increases in the number of interstitial cells, many of which were likely myofibroblasts. Although these results raise the possibility that myofibroblasts might be involved in epithelial regeneration, other interstitial cells such as macrophages are known to be involved in kidney repair, and a detailed characterization of the interstitial cell compartment following transient kidney injury is lacking.
Effect of Age
For many organs, including the kidney, the capacity to recover from injury decreases with age, an observation familiar to most practicing nephrologists. In most organs, it remains to be determined whether this is due to a decrease in the number of organ-specific stem cells or to the inability of the stem cells to be activated, but recent work suggest that the latter is more likely (reviewed by Liu and Rando ). For example, it was recently found that aging muscle had a normal number of satellite cells, but the cells failed to activate in response to exercise. Interestingly, progenitor/stem cells in advanced age can display a “young” response by exposure to a young systemic environment, indicating that the changes responsible for the functional decrease of precursor/stem cells are potentially reversible.
While the effect of age on the renal proliferative capacity after injury has not been studied in detail, it appears that cell proliferation after acute kidney injury decreases with age, in agreement with the poor prognosis of recovery from acute kidney injury in elderly patients. Mechanisms that could account for these observations include telomere shortening and increased expression by renal epithelial cells of zinc-α 2 -glycoprotein (Zag), an adipokine associated with cachexia. To examine whether the poor regenerative capacity of the aging kidney is due to a decrease in the numbers or in the functionality of putative renal stem/progenitor cells awaits definitive identification of these cells.
In sum, both during normal conditions and particularly after transient kidney injury, the S3 segment of the proximal tubule is a site of intense cell proliferation, suggesting the presence of progenitor cells in this part of the nephron. In addition, the upper part of the papilla and close to the urinary space is also a site of robust cell cycling, both under normal conditions and after transient kidney injury.
Identification of Potential Precursor/Stem Cells in the Adult Kidney
Studies from several laboratories have presented evidence characterizing renal precursor cells in the adult kidney, but none of these cells meet strict criteria for traditional adult, organ-specific stem cells; i.e., asymmetric cell division and multipotency. Nonetheless, since our ultimate aim is to understand the origin of new cells in the adult kidney so that the responsible mechanisms might be manipulated, these studies are worth reviewing. In addition, the results of these studies are likely to be useful for future work to identify adult renal stem cells. Several strategies have been used to identify and characterize adult kidney precursor/stem cells, and the robustness of the obtained results varies widely. In our view, the methodological approach used for cell identification and/or isolation of the cells best separates these studies.
Cellular Markers
Several workers have isolated cells from the adult kidney using a candidate gene approach. For example, Busolatti et al. used the transmembrane glycoprotein Cd133, which is expressed in hematopoietic stem cells, endothelial progenitor cells, neuronal, glial, and glioblastoma stem cells, as well as some other cell types (see Mizrak et al. for a review), to select cells from the adult human kidney. The isolated cells showed strong clonogenic capacity, and when subcutaneously transplanted to SCID mice formed tubules which expressed some renal epithelial markers. Finally, when the cells were injected intravenously into mice with glycerol-mediated acute kidney injury, they integrated into tubules. However, the functional significance of the integration was not explored. Using a somewhat similar approach, Dekel et al. used Sca-1, a protein identified in several progenitor cells, to isolate a cell population from mouse kidneys. However, because Sca-1 is also expressed in renal epithelia, these workers used a collagenase digestion approach that appeared to yield interstitial cells. The in vitro differentiation of these cells suggested that they were mesenchymal-like stem cells, since they differentiated into myogenic, osteogenic, adipogenic, and neural lineages. When injected into kidneys with acute kidney injury, they also incorporated into tubules.
In Vitro Growth Behavior
Several laboratories have isolated cells from adult kidneys based on their characteristics when cultured in vitro . For example, cells were selected because of their high clonogenic potential, ability to outlast all other cultured cells or their ability to change their phenotype. For example, Gupta et al., cultured cells from collagenase digested rat kidneys, and after ~5 weeks in culture they obtained a cell population with substantial proliferative potential (which in fact was the characteristic that lead to isolation of the cells) and expressing transcription factors such as Pax-2 and Oct4 that are either involved in renal development or in determining cell fate. When the cells were injected into the subcapsular space of normal kidneys, some of them incorporated into tubules.
Kitamura et al. used a slightly different strategy by isolating single nephrons from adult rat kidneys and culturing them in vitro . Cells growing out from these nephrons were harvested and individually cultured; and the clone with the fastest proliferation capacity was characterized. These clonal cells expressed several proteins important in kidney development and proteins characteristic of mature nephrons. When the cells were injected in the subcapsular region of a kidney with acute kidney injury, the cells engrafted into renal tubules.
More recently, Lee et al. used a mouse with a targeted mutation in which GFP is expressed under the control of Myh9 , a gene expressed in interstitial cells (among others). After isolation of the GFP + cells from the kidney and subsequent culture for eight weeks, they isolated a cell line that expressed renal embryonic or stem cell markers such as Oct-4 , Pax-2 , Wnt-4 , and WT-1 . Immunolocation of the endogenous Oct-4 + and GFP + cells in the kidney found them in the interstitial space of the medulla and papilla. Finally, when the cells were injected directly into kidneys after acute kidney injury, they incorporated into tubules and partially decreased the functional consequences of the injury.
In Vivo Growth Behavior
The hypothesis that adult, organ-specific stem cells divide infrequently has been a powerful tool in locating and identifying several stem/precursor cells. According to this hypothesis, due to their low cycling behavior, stem cells retain S-phase labels (traditionally 3 H-Thymidine or a thymidine analog such as BrdU) when given as a short pulse followed by a long time of chase. Recent work has, however, demonstrated that there is more complexity than originally thought (see Fuchs for an informed discussion), as not all stem cells are low cycling and “label retention” experiments identify a heterogeneous group of cells. Nonetheless, populations of “label-retaining cells” from several organs are enriched for stem cells, and identification of a “label-retaining” group of cells is of great interest. To detect low cycling cells, an S-phase label is only administered during a short period of intense growth (e.g., embryonic life or shortly after birth), so that all cells are cycling and incorporate the label; during the subsequent period of chase the label is diluted and lost by the progeny of dividing cells and selectively retained by low- or non-cycling cells. Cells cycling infrequently were thus named “label-retaining cells” (LRC). Several investigators have used this approach in the kidney.
Maeshima et al. administered BrdU for one week to adult rats that were then “chased” for two weeks, and found that there were many BrdU-retaining cells the kidney parenchyma, particularly in the proximal tubules. Following transient ischemic injury, most of the tubular cells that were proliferating (expressing proliferating nuclear antigen; PCNA) were also BrdU-labeled cells. These results led Maeshima et al. to conclude that a “label-retaining” cell population was the precursor of new cells during kidney repair from injury. However, the long pulse and short period of chase complicate the interpretation of these results. In addition, the increased number of BrdU-labeled cells after injury was likely due to detection of the cells loaded with BrdU during the “pulse” plus their immediate progeny after injury. Nonetheless, because a relatively small population of cells were labeled during the one-week “pulse” and many of these cells were also PCNA positive after injury, this experiment suggests that both during normal conditions and during repair from injury a group of renal epithelial cells cycles at a much higher frequency that most other epithelial cells. This raises the possibility that these cells could be adult, renal epithelial precursors.
We exploited the low cycling frequency of adult stem cells by giving BrdU to newborn rats or mice during a short pulse, and “chased” the animals to adulthood. In addition, to circumvent the use of BrdU, we also followed the suggestion of Tumbar et al. and used transgenic mice that expressed a fusion protein of histone 2B and GFP (referred as H2B-GFP mice) under the control of a tetracycline-responsive element. Pregnant females given doxycycline give birth to pups where all cells are GFP + cells, but when these pups are chased to adulthood, only low-cycling cells remain GFP + . As shown in the Figure 29.4 , after 2–3 months of chase, label-retaining cells were only found in the kidney papilla. When isolated, the cells showed pluripotency and, like other stem cells, formed spheres.

Under normal conditions, most of these papillary label-retaining cells (LRC) in the adult kidney are, unsurprisingly, in growth arrest, but we found in the upper papilla a small population of them that were cycling (see Figure 29.5 ), indicating that they generate new cells and explaining that the number of papillary LRC slowly decreased with age. In marked contrast to the normally low-cycling frequency of the papillary LRC, many of these cells proliferated shortly after acute kidney injury, likely generating many daughter cells, since after a few weeks the S-phase label disappeared from the papilla (see Figure 29.6 ). Hence, the cells we identified in the kidney papilla showed two cycling characteristics: first, during normal homeostasis, the cells are low-cycling (and thus they are LRC) except for a small population of them that are cycling in the upper papilla; second, and in marked contrast to normal conditions, may of the papillary LRC start cycling shortly after injury. These results strongly suggest that the population of LRC of the kidney papilla is involved in kidney repair, and that within the LRC cells are renal stem/precursor cells.


Because the renal capsule contains a population of nestin-expressing cells, and this intermediate filament protein has been found in a variety of precursor cells, Park and co-workers searched for low-cycling cells in this part of the kidney. They administered a short pulse of BrdU to 4-week-old mice, and followed the pulse by a chase period of two months. They found that the kidney capsule had a sparse population of BrdU-retaining cells which further characterization showed them to be CD29 + , Sca-1 + , vimentin + , and nestin + , but that did not express hematopoietic or endothelial markers. These cells showed marked proliferative and clonogenic capacities, and could differentiate towards adipogenic, chondrogenic, and osteogenic lineages, suggesting that they were MSC or MSC-like. The function of these cells in vivo remains unknown, and it would be of great interests to determine whether kidney injury induces their proliferation.
Cellular Function
Since first used to enrich for hematopoietic stem cells, the Side Population discrimination assay has been used to identify stem cells and progenitor cells in a variety of organs and tumors. The assay can be useful when stem cell-specific markers are not available, and it is based on the differential ability of cells to efflux a lipophilic fluorescent dye via the ATP-binding cassette transporter protein ABCG2. Many stem/precursor cells rapidly efflux the Hoechst 33342 dye, a process that can be blocked with ABC transporter inhibitors. By flow cytometry, such cells are recognized as being “Hoechst low” (versus “Hoechst high”) and as they are a very small fraction of the total cells, they were termed side population (SP) cells. Because the assay is dependent on normal cellular metabolic function in freshly isolated cells, it is extremely challenging (for a detailed discussion see Golebiewska et al. ), and uncertainty exists about the significance of some of the results. Nonetheless several workers have isolated SP cells from the adult kidney. The frequency of these cells has been reported to vary from ~5% to ~0.1%. In one study, exogenous administration of the isolated cells to mice with cisplatin-induced acute kidney injury improved the kidney’s functional response. These provocative results need further analysis, particularly because the SP of the kidney appears to show considerable heterogeneity. However, the kidney is a transporting organ with a large number of ABC-type transporters expressed in several of its segments including ABCG2, the putative transporter of the Hoechst33342 dye. Hence, the presence of cells that pump the dye out may not be the best marker for progenitor cells.
Another cellular function that has been exploited to isolate putative renal stem/precursor cells is the aldehyde dehydrogenase activity (ALDH). This enzyme oxidizes intracellular aldehydes (for a review see Sophos and Vasiliou ), and it is believed to have a role in cellular differentiation by its ability to oxidize retinol to retinoic acid. Hematopoietic, neural, and some cancer stem/precuror cells were found to have high ALDH enzymatic activity. Similarly, Lindgren et al. recently isolated from the human kidneys cells with progenitor characteristics. Using an ALDH enzymatic assay in suspensions of renal cortical cells deprived of glomeruli, they separated the cells into ALDH low and ALDH high populations. Interestingly, transcription analysis of the populations showed that one of the most upregulated genes in the ALDH high cells was Cd133, a membrane protein found in some progenitor/stem cells and used to isolate other renal progenitors (see above and the section on podocyte precursors next). In vitro , the cells showed some stem/precursor cell characteristics, but of particular interest is their finding by immunohistochemistry that there is a population of Cd133-positive cells scattered through the proximal tubule, clearly showing that there is some heterogeneity in the proximal tubular cells. Analyses of kidney biopsies of patients with acute tubular necrosis showed that in areas of regeneration tubular cells positive for Cd133 in their luminal membrane were frequently found to be also positive for vimentin in their basolateral membrane. Since tubular epithelial cells expressing vimentin have been observed during the recovery phase of acute kidney injury, it is possible that these Cd133- and vimentin-expressing cells might be specialized epithelial precursors.
Podocyte Precursors
It had long been assumed that in adult kidneys podocytes, the specialized epithelial cells that surround the glomerular capillary loops, have extremely low or no cycling activity. Yet, these cells are clearly renewed because they can be captured in the urine of normal subjects. Interest in the origin of podocytes and their life cycle has been spurred by observations that their numbers can decrease during diseases caused by several mutations that disrupt their proteins, leading to glomerular sclerosis (reviewed by D’Agati ). In addition, it was recently found that a variable fraction of the cells in the glomerular “crescents” (layers of abnormal cells attached to the Bowman’s capsule) often present in glomerulonephritis were found to express nestin. Since normal podocytes express nestin, these results raised the possibility that cells in crescents might derive from podocytes or that podocytes and cells in crescents share a common lineage. Regardless, these interesting observations suggested that both podocyte loss and/or their uncontrolled proliferation might be involved in renal diseases.
Analyzing human kidney sections, Sagrinati et al. found that a subpopulation of the parietal epithelial cells of Bowman’s capsule expressed Cd24 and Cd133, two cell surface proteins found in a variety of adult stem cells. Isolation of these cells from glomerular cultures showed them to possess high cloning efficiency and some differentiation potential. In addition, when the cells were intravenously administered to mice with acute kidney injury, there was improvement in the morphological and functional renal response. The observation that some epithelial cells in the parietal side of the Bowman’s capsule had progenitor characteristics led to the hypothesis that these cells could be podocyte precursors. With this in mind, Ronconi et al. analyzed human kidneys sections, and confirmed that a group of cells in the parietal epithelia of the Bowman’s capsule expressed Cd133 and Cd24 and that, in contrast, fully differentiated podocytes only expressed proteins used to identify them such as nestin, complement receptor-1, and podocalyxin. Interestingly, they also identified a third cell population that expressed Cd133, Cd24, and podocyte markers, suggesting that it could be a podocyte precursor. Isolation of these three different cell populations by FACS using Cd133, Cd24, and podocalyxin and their culture in vitro showed that a cell clone derived from a cell only positive for Cd133 and Cd24 contained cells that expressed both tubular epithelial markers and podocyte markers. In contrast, clones of cells derived from cells positive for Cd133, Cd24 plus podocalyxin only expressed podocyte markers. When these two different types of cells were intravenously administered to mice that had received the podocyte toxin adriamycin, they found that cells only positive for Cd133 and Cd24, but not cells positive for Cd133, Cd24, and podocalyxin, were capable of reducing urinary protein excretion and morphologic damage. Finally, labeling the cells with vital dyes showed that the former, but not the latter, localized to glomeruli. These results were interpreted as indicating that in adult kidney, podocytes derive from a precursor cell population that resides in the parietal epithelium of Bowman’s capsule. One might conclude that the study shows heterogeneity in the parietal epithelial cells of Bowman’s capsule, and that in vitro these cell types express different genes. The mechanism responsible for the functional recovery after cell injection and its significance is harder to define, since the large majority of epithelial cells administered intravenously are likely to lodge into the lung vascular bed.
The hypothesis that parietal epithelial cells might migrate into the glomerular capillaries and differentiate into podocytes was also examined by Appel et al. in a detailed and elegant study. These authors reported that in adult rats, cells that had morphological characteristics of both glomerular parietal epithelial cells and podocytes could be detected by electron microscopy in the glomerular vascular stacks. That these cells could be transitional cells between parietal epithelial cells and podocytes was examined in 10-day-old mice with a variety of approaches. By immune-detection they found that some cells had markers of both parietal epithelial cells and podocytes. Administration of BrdU to young rats for two weeks showed that, while a substantial number of parietal epithelial cells were labeled with BrdU, only ~0.5% of podocytes were labeled. However, as the animals aged, the number of BrdU + parietal epithelial cells decreased, while that of BrdU + podocytes increased, suggesting that the latter derived from the former. In a final genetic fate-mapping experiment, these workers generated triple-transgenic mice in which labeling of the parietal epithelial cells and their progeny could be temporarily controlled by doxycycline administration. In 5-day-old and 10-day-old mice they found that the number of podocytes that derived from parietal epithelial cells increased as the animals aged. This elegant study provides strong evidence that podocytes derive from parietal epithelial cells, at least in the young. This is a very exciting result, because even if this process only occurs at very young ages, understanding of its controlling mechanisms might allow its modulation. An additional conclusion appears also important; it is clear that as our understanding of the homeostasis of the renal cell populations advances, the distinction between embryonic and adult lives is becoming less clear-cut than previously believed (i.e., “development” appears to be a life-long process), a subject to which we return below.
Because a variety of glomerulonephritis types are associated with hyperplasia of Bowman’s capsule (a histological finding long ago termed “crescents”), the possibility that unregulated proliferation of a precursor cell could be responsible for their generation was examined. In a study of human kidney biopsies, the hypercellular lesions of crescentic glomerulonephritis were analyzed with antibodies and RT-PCR after laser capture of the lesions. The authors found that these lesions contained three kinds of cells: Cd133 + , Cd24 + , podocalyxin − and nestin − (presumed podocyte precursors), Cd133 + , Cd24 + , podocalyxin + and nestin + (named intermediate cells), and finally, Cd133 − , Cd24 − but podocalyxin + and nestin + (same profile as that of differentiated podocytes). When the lesions were examined for the presence of proliferating cells with a Ki67 antibody, most of the cells that were cycling were only positive for Cd133 + , Cd24 + . Further, in vitro , Cd133 + , Cd24 + , podocalyxin − and nestin − cells responded robustly to TGFβ induction of extracellular proteins. These results were taken as evidence that generation of glomerular crescents is likely due to proliferation of “podocyte progenitors.” In a related experimental study, Smeets et al. induced crescentic glomerulonephritis in triple-transgenic mice in which parietal epithelial cells and their progeny could be labeled by the administration of doxycycline. In these mice, “crescents” could be detected to be positive by enzymatic X-gal staining, strongly suggesting that these cells derived from epithelial cells of the Bowman’s capsule. In contrast, podocyte genetic labeling experiments did not show that the cells in the crescents derived from podocytes. These interesting results strongly suggest that some cells in the parietal epithelium of the Bowman’s capsule, and not the podocytes, proliferate in response to injury.
However, in a fascinating study, Ding et al. found that deletion of the product of the von Hippel-Lindau gene ( Vhlh , encoding VHL) specifically in podocytes caused glomerulonephritis with crescent formation. Loss of VHL stabilizes the hypoxia-inducible factor (HIF) α-subunit and activates hypoxia-response genes. Of great interest is their finding that the cells in the crescents were derived from podocytes; this was elegantly shown by laser-capture of the crescents and PCR demonstrating the rearrangement of the genomic DNA induced in the podocytes (a tour de force control experiment which unfortunately is rarely done). In addition, because the gene expression profile revealed induction of Cxcr4 expression in glomeruli, Ding and co-workers overexpressed this receptor in podocytes; this resulted in podocyte proliferation and glomerulonephritis. This extremely interesting study therefore indicates that under some conditions, podocytes can proliferate, likely by the effect of Cxcl12 (SDF-1) the ligand of Cxcr4, and that their proliferation can lead to “crescent” formation.
Renal Mesenchymal Stem Cells and/or Pericytes
Mesenchymal stem cells (MSC) from the bone marrow were originally characterized by their ability to adhere to culture surfaces and when cultured with appropriate differentiation media, by their differentiation potential into adipocytes, osteoblasts, chondrocytes, and, sometimes, myoblasts. In other organs including the kidneys, MSC identification and characterization remains to be formalized, since they might be a heterogeneous population that expresses a variety of cell surface markers. Nonetheless, there is widespread interest in MSC because, in addition to their differentiation potential, they appear to be present in most if not all organs including the kidney, and their precise function remains to be defined. They preferentially locate in the perivascular space of even large blood vessels such as the aorta and vena cava, and have pericycte-like characteristics. In the kidney, mesangial cells also have MSC-like characteristics, and might be the glomerular cells that express Cd146 + and Cd133 − found by Bruno et al. in glomeruli. Because of their perivascular location and mesenchymal origin, pericytes are difficult to distinguish from MSCs, and how these two cells differ and relate is still poorly-understood. Although true renal pericytes can only be unambiguously identified by their anatomical location surrounding capillary endothelial cells, these cells have been implicated in myofibrobalst generation during renal fibrogenesis, and are discussed in detail below. In organs other than the kidney, pericytes have been found to have MSC-like characteristics, and in the most detailed study to date, perivascular cells from skeletal muscle, bone marrow, and adipose tissue were found to express several pericyte markers, as well as MSC markers and MSC differentiation potential, suggesting that MSC and pericytes might be the same cells in different locations. It has been shown that during tooth growth and in response to injury, pericytes can give rise to MSC, although in the same organ MSC have an additional, pericyte-independent origin, so that lineage analysis between MSC and pericytes is likely to be complex. In the kidney, mesangial cells, vascular smooth muscle, pericytes, and likely, MSC derive from the Foxd1 embryonic cellular compartment described above but as stated, the exact relationship among these different cells remains to be defined.
It is currently unknown whether renal pericytes/MSCs generate daughter cells important for normal kidney homeostasis or for repair from injury. However, recent studies showing that pericytes characterized as Cd73 + , platelet-derived growth factor receptor + and smooth muscle actin negative, give rise to myofibroblasts during renal fibrosis have generated a great deal of interest, a finding that we discuss below. However, morphological observations indicating the transient presence of myofibroblasts after transient kidney injury suggest that these cells, long studied from a pathogenic perspective, might also be involved in organ repair, a possibility that we believe deserves attention. Regardless, since like other organs, the kidney contains perivascular cells that are pericytes, MSC or their immediate progenitors, their full characterization and role in normal renal homeostasis and if any, in organ repair from injury, would be of great interest.
Potential Sites Harboring Renal Stem Cells
Given the kidney’s anatomical complexity, identification of stem cells within it might be facilitated by focusing on sites that might harbor them. Several strategies are possible to locate candidate sites, but we believe that current evidence already suggests the presence of sites likely to contain stem cells.
Highly Proliferative Regions
Because many adult stem cells are low-cycling (see for recent reviews), to generate needed new cells they frequently give rise to an immediate progeny that experience several “transit amplifying” (TA) divisions prior to their differentiation. In this way, many new cells can be regenerated from infrequent stem cell cycling. Hence, adult stem cells can be located close to sites of increased cell cycling. Indeed a seminal discovery in this field showed that there was postnatal neurogenesis in the granular zone of the dentate gyrus in the hippocampus, and led to the discovery that the subgranular zone harbored neural stem cells (reviewed in Zhao et al. ). Hence, proliferative regions during normal homeostasis or after kidney injury are good candidates to harbor renal stem cells.
After nephrogenesis is completed in young rats and during normal homeostasis, it was found that the S3 segment of the proximal tubule displayed high cycling activity : over a two-week period in 4-week-old rats, 90% of the cells of the S3 segment had cycled, a remarkable rate of proliferation. While this may reflect morphogenetic requirements of a growing kidney, the high proliferative capacity of these cells raises the possibility that they could be “transit amplifying” daughter cells of a proximal tubule stem cell. Other findings support that the S3 segment of the proximal tubule might contain stem cells. Shortly after several types of kidney injury, high proliferation activity was particularly prominent in cells from the S3 segment. Moreover, Gupta et al. found cells in the cortico–medullary junction which expressed Oct-4 and had progenitor characteristics, and Kitamura et al. isolated a highly proliferative cell type from the S3 segment of rat kidneys that, at least in vitro , also expressed precursor cell markers. Whether these putative stem cells relate to the recently described podocyte precursor (see above) or renal proximal tubule progenitors remains to be determined.
The S3 segment of the proximal tubule is of additional interest as a potential site to harbor renal stem cells, because indirect determinations of oxygen tension have shown that this part of the nephron surprisingly exists in a hypoxic environment quite different from other parts of the kidney cortex. A low oxygen tension is characteristic of sites where stem cells in other organs reside, a subject that we discuss below.
To determine whether the adult kidney contained regions of increased cell proliferation, we used kidneys of one-year-old rats, because we reasoned that fully grown older animals would not have proliferating cells needed for organ growth. We found cycling cells by staining for Ki67, a marker of cellular proliferation. As shown in Figure 29.1 , Ki67-positive cells were rare in the cortex and medulla, and were solitary and of a similar frequency (~1 % of the total cells). In contrast, the papilla showed marked heterogeneity in its abundance of Ki67-positive cells; they were extremely rare in its tip and middle part (~0.1 %), but were readily apparent in the upper part of the peripheral papilla, adjacent to the urinary space (US) where the kidney parenchyma forms a narrow angle which provides an easily identifiable landmark and the papilla meets the medulla; it is also very close to the kidney cortex. In this region, and unlike other areas of the kidney, Ki67-positive cells frequently formed chain-like structures and accounted for ~2.5 % of the total cells, a significant difference from other parts of the kidney. When cellular proliferation was assayed 24 hours after labeling cells in S-phase by administration of a single dose of BrdU, proliferating cells were very rarely detected in the cortex and medulla but again, the lateral part of the upper papilla consistently contained cycling cells; i.e., BrdU-labeled (not shown). We also obtained similar results in kidney of six-month-old rats.
Interestingly, after acute kidney injury, the lateral part of the upper papilla is also the site in which we first detected the increased cell cycling that follows injury; rats were subjected to 45 minutes of unilateral kidney ischemia, following which were given a single dose of BrdU and sacrificed one hour later. Thus, only cells that were in S-phase during this hour could be labeled, and using 5 μm kidney sections, we saw only equally rare labeled cells in normal kidneys or in the kidneys subjected to ischemia. However, examination of 100 μm vibratome sections showed abundant cells in S-phase in the upper papilla ( Figure 29.3 ), next to the urinary space (US) only in the kidneys subjected to ischemia. In the aggregate, these results indicate that the lateral upper part of the kidney papilla contains more proliferating cells than other parts of the kidney under normal conditions, and that it contains the first cells that start cycling in response to transient injury. As reviewed above, Vinsonneau et al. found that the first intrarenal area that contains Ki67 positive cells after ischemia reperfusion injury was the uro-epithelium and adjacent interstitial space in the upper part of the urinary space at the papilla–medullary junction.
In sum, because of their proliferative behavior, both under homeostatic conditions and during repair from several types of kidney injury, the S3 segment of the proximal tubule and the lateral part of the upper papilla are domains of the kidney where stem cells appear likely to reside.
Stem Cell “Niches”
Adult stem cells receive critical signaling from their immediate microenvironment, a domain that is distinct from the rest of the organ (see Morrison and Spradling for a review). In these locations (referred to as “niches”), stem cells interact with other cells and the extracellular matrix, a process that is best understood in the Drosophila germline stem cells (see Fuller and Spradling for a review ). The stem cell “niche” provides positional information that regulates both proliferation activity and cell fate identity, and in fact it can even redirect cell fate similar to what often happens during embryogenesis. While characterization of mammalian stem cell niches is still very limited, some common motifs are emerging that might be useful to identify the location of renal stem cells. Among those, a hypoxic environment is a prominent characteristic. Several organ-specific stem cells such as hematopoietic stem cells and neural stem cells reside in hypoxic regions, and a large body of in vitro work has shown the critical role of oxygen on stem cell regulation (see Mohyeldin et al. for a review). Moreover, in both hematopoietic stem cells and neural stem cells, it was recently found that regulation of the oxygen-sensitive gene HIF-1α is critical for the cells’ maintenance and fate decisions.
The kidney parenchyma possesses steep oxygen gradients, and variations in renal oxygenation are undoubtedly sensed as they regulate erythropoietin synthesis. While direct determinations of oxygen tension in the kidney papilla have not been reported, it is likely that papillary pO 2 ranges are ~4–10 mmHg. The presence of low-cycling cells in this very hypoxic environment and that many of these cells express nestin, an intermediate filament protein identified in a variety of progenitor/stem cells, suggests that the papilla harbors renal stem cells. Interestingly, using a nestin-GFP-expressing mouse, nestin + cells were detected in large clusters within the papilla, along the vasa rectae and, at least in vitro , these papillary nesting-expressing cells were found to migrate upwards in the papilla towards the cortex, an observation compatible with our in vivo experiments with vital dyes in which we found upward migration of papillary cells, some of which were “label-retaining cells”.
Several other findings also suggest that the kidney papilla is a niche for renal stem/precursor cells. Dekel and co-workers isolated a population of purportedly renal stem cells from the renal interstitium that expressed the stem cell antigen-1 (Sca-1) and were enriched for β1-integrin; when the cells were located in vivo , they were found to be particularly abundant in the renal papilla. Using a different approach, Lee et al. identified a renal interstitial cell that expressed several potential renal progenitor cell markers, such as Oct-4 , Pax-2 , Wnt-4 , and WT-1 . In vivo , Oct-4 + cells were located in the medulla as well as in the papilla, leading these authors to suggest that the interstitium in these regions of the kidney is a “niche” for renal stem cells. Finally, and with a very different approach, Curtis et al. examined whether cells from different regions of the kidneys were equally capable of contributing to cell regeneration after acute kidney injury. These workers isolated from mice constitutively expressing β-galactosidase in certain cells from the kidney cortex, medulla or papilla, and implanted them under the capsule of kidneys subjected to ischemia-reperfusion injury. Seven days later, implanted cells deriving from the three different regions of the kidney could be detected in the tubules of the renal medulla, but cells obtained from the papilla exhibited the most robust engraftment. These results suggest that cells deriving from all regions of the kidney might contribute to the reparative response of the organ after injury, and that some cells in the papilla are particularly capable of contributing to repair and might be stem cells.
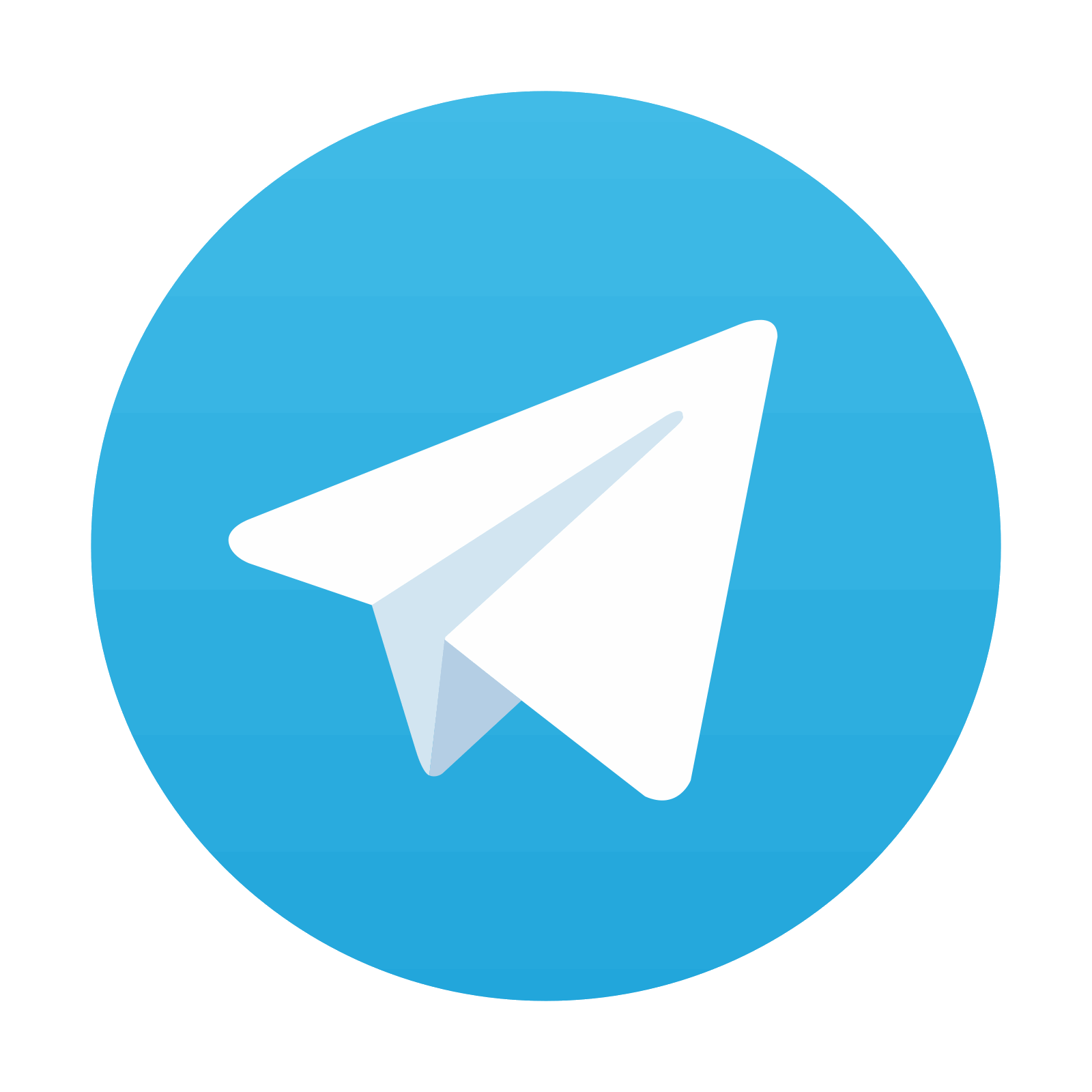
Stay updated, free articles. Join our Telegram channel
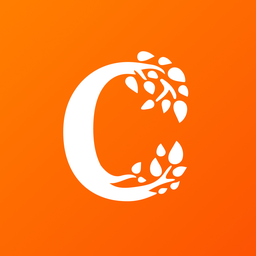
Full access? Get Clinical Tree
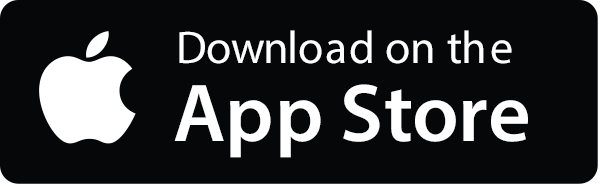
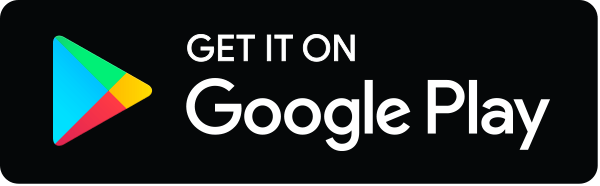