Acute ischemia reperfusion injury (IRI) is a major cause of acute kidney injury (AKI). A major conceptual insight is that the total amount of renal injury is the result of not only the initial ischemic insult, by the ensuing responses. This simple insight has profound clinical implications: it suggests that therapy after the initial insult has already occurred may ameliorate the course of AKI. Some of these responses are maladaptive. Examples include inappropriate intrarenal hemodynamics, altered mitochondrial and other metabolic functions, endothelial dysfunction, and tubular obstruction and back-leak. Other responses are reparative. Examples include the production of growth factors such as Wnt7.
In addition to the above, IRI elicits an inflammatory response. Some components of this inflammatory response exacerbate injury, other components mediate repair. In either case, inflammation is a major determinant of the ultimate outcome of ischemic AKI. A complete understanding of the inflammatory response to IRI—the composition and functions of the leukocytes, the stimuli that elicit inflammation, and the regulation of inflammation—remain a fundamental unsolved problem in renal disease. The goal of this chapter to provide a perspective on our rapidly growing insights into the inflammatory response to IRI.
Introduction
Acute ischemia reperfusion injury (IRI) is a major cause of acute kidney injury (AKI). A major conceptual insight is that the total amount of renal injury is the result of not only the initial ischemic insult, by the ensuing responses. This simple insight has profound clinical implications: it suggests that therapy after the initial insult has already occurred may ameliorate the course of AKI (see Figure 88.1 ). Some of these responses are maladaptive. Examples include inappropriate intrarenal hemodynamics, altered mitochondrial and other metabolic functions, endothelial dysfunction, and tubular obstruction and back-leak. Other responses are reparative. Examples include the production of growth factors such as Wnt7.

In addition to the above, IRI elicits an inflammatory response. Some components of this inflammatory response exacerbate injury, other components mediate repair. In either case, inflammation is a major determinant of the ultimate outcome of ischemic AKI. A complete understanding of the inflammatory response to IRI—the composition and functions of the leukocytes, the stimuli that elicit inflammation, and the regulation of inflammation—remain a fundamental unsolved problem in renal disease. The goal of this chapter to provide a perspective on our rapidly growing insights into the inflammatory response to IRI.
In addition to its importance for injury of the native kidney, the inflammatory response to IRI is important for transplantation. Ischemic injury to the allograft inevitably accompanies organ harvesting, the cold storage, and the warm ischemia during the surgical creation of the vascular anastomoses between allograft and recipient. In addition, for brain dead donors, the donor kidneys are damaged by the hemodynamic instability resulting from the trauma that causes brain death. The leukocytes recruited to the allograft by ischemia exacerbate rejection. This is predicted by the “danger” theory of immunology where injury elicits inflammation, the leukocytes detect non-self at the site of injury (infectious pathogens or the allograft), and then develop an immune response against the non-self. The relation of this “injury response” to rejection has previously been reviewed.
An inflammatory response is also elicited by chronic injuries in common human diseases such as progressive renal failure from diabetes and hypertension. Understanding the inflammatory response to the “single hit” model of acute ischemia may aid our understanding of the more complicated chronic diseases. The goal of this chapter is to review our current understanding of this rapidly evolving field. Although complement and gene activation by hypoxia/reactive oxygen species are important, our focus will be on the nature of the inflammation and its regulation by injured and dying cells.
Most studies of the inflammatory response to renal ischemia have involved rodents. The potential difficulties in extrapolating such studies to the clinical setting have been discussed previously. Although inflammation is not a prominent feature of human ischemic acute renal failure, leukocytes are present. The susceptibility of patients to acute renal failure may correlate with polymorphisms of pro- and anti- inflammatory genes; and this further supports a role of inflammation in the pathogenesis of human disease. Biopsy studies during ischemic acute renal failure of native human kidneys are limited, but post-anastomosis biopsies of renal allografts are increasingly common. Most such biopsies indicate inflammation, particularly in deceased, compared to living, donors. Furthermore, intraoperative biopsies have also indicated expression of pro-inflammatory genes. Such inflammation may be a response to ischemic injury to the allograft due to the hypotension associated with the trauma that caused brain death, due to the cold storage, and due to the warm ischemia during creation of the vascular anastomoses. In addition, inflammation in the cadaver kidneys was also caused by neurohormonal effects of brain death. Inflammation during these intraoperative biopsies are not due to rejection because there is no time for immune recognition of the transplant. Furthermore, biopsies of kidneys between identical twins, where there is no allo-recognition, also shows inflammation that must be due to ischemic injury occurring during the transplant process.
Some argued that injury and damage to kidney is not the major contributor to most cases of human ischemic AKI. Instead the major factor is abnormal renal microvascular function and use of oxygen. Indeed, in those rare cases where the kidney is biopsied, the morphologic injury by conventional staining techniques are minimal. However, the longterm maladaptive effects of ischemic AKI suggests that injury does occur and the kidney never completely recovers from this injury. Indeed, recurrent episodes of ischemic AKI may be a major contributor to the current epidemic of CKD.
The medullary thick ascending limb and the S3 straight proximal tubule in the outer medulla are the tubules most vulnerable to ischemic injury in both rodents and humans. This is the area with the greatest inflammation. Although the outer medulla is the injured earliest and after the least ischemia, longer periods of ischemia result in the injury of the cortex also. Because different structures in the kidney may be injured depending on the intensity of ischemia, and because these structures produce different cytokines, chemokines, and other regulatory molecules in response to IRI, renal IRI may be a family of diseases rather than a single entity. Thus, the length of ischemia time, or the temporature of ischemia may modify the inflammatory response to IRI.
The inflammatory response to acute ischemia of the heart, and brain have been more intensively studied than ischemic acute renal failure because of the greater clinical incidence of coronary artery disease and stroke. Where renal studies are not available, we will review studies from these and other non-renal organs. Although the general principles may be the same in these various organs, the particular mechanisms of the inflammatory response to ischemic injury may be different in different organs. For example, blocking the pro-inflammatory cytokine interleukin 1α and β ameliorates ischemic injury of the rodent brain and heart, but has no effect on ischemic acute renal failure.
Leukocytes in Injured, Ischemic Tissues: Friend, and Foe.
Over a decade ago, anti-inflammatory agents were shown to ameliorate ischemic acute renal failure. These studies demonstrated the maladaptive effects of the inflammatory response to injury. Recent studies elucidate greater detail about which leukocytes are involved and how they regulate renal IRI.
Mononuclear phagocytes—monocytes, macrophages and dendritic cells : The relationship of the various members of the mononuclear phagocyte family—monoctye, macrophages, and dendritics, and there various subsets—is complex. Various mononuclear phagocytes participate in ischemic AKI. Some exacerbate injury. Others facilitate repair.
These leukocytes exacerbate the early phases of ischemic injury. Macrophages appear within hours after ischemic injury in both mice and rats; these macrophages are located adjacent to the vasa rectae of the outer medulla. This is the region of the rodent kidney that is most vulnerable to ischemic injury, and where there is endothelial injury and expression of both B7 and adhesion molecules.
Elimination of this early macrophage infiltrate prevented the increased interleukin 6 that occurs after renal ischemia. The former exacerbate ischemic renal injury because its elimination by transgenic knockout, or anti-interleukin-6 antibodies ameliorates renal injury. In situ hybridization shows that interleukin 6 is produced by macrophages in the ischemic kidneys; the construction of bone marrow chimeras where renal parenchymal cells or macrophages have their IL6 gene knocked out showed that the greatest injury occurred when macrophages were capable of making interleukin 6. Macrophages are also capable of producing a number of other molecules that might exacerbate ischemic acute renal failure. However, as discussed below, what cytokines are produced by which renal cells, and which cytokines have harmful versus helpful effects remain to be clearly delineated.
In these studies, there was an early infiltration of macrophages in the absence of neutrophils. This sequence of macrophages then neutrophils contradicts the classical paradigm which proposes that neutrophils infiltrate first, and produce molecules that recruit monocytes subsequently. However, recent data indicate that monocytes can infiltrate tissues early and, in some cases, in the absence of neutrophils. In the lung and liver, macrophage inflammation may precede neutrophilic inflammation. This may also occur in ischemic acute renal failure. Furthermore, the nature of renal cell death during ischemic AKI may regulate the type of inflammation. Apoptosis generally recruit macrophages, but necrosis recruits neutrophils. We discuss the various types of cell death and their effects in inflammation later in this chapter.
In addition to this early infiltrate, there is also a late infiltrate of macrophages and related dendritic cells during the first weeks after acute ischemic injury. Large numbers of these leukocytes are still present after the recovery of glomerular filtration has already occurred. The contribution of these leukocytes to renal injury and repair is not known. On the one hand, they may contribute to chronic injury. On the other hand, some macrophage subpopulations participate in tissue repair, perhaps through the secretion of growth factors such as Wnt7b or anti-inflammatory cytokines such as interleukin 10. These macrophages have many attributes of “M2” macrophages present late after infections.
Macrophage infiltration into the outer medulla is regulated by endothelia. Endothelia are the border between the vasculature and the renal interstitium. Thus, the quantity and composition of leukocyte traffic from blood into the renal interstitial spaces is regulated by proinflammatory genes expressed by endothelia. Ischemic endothelia in the outer medulla do increase their expression of pro-inflammatory ICAM-1 (CD54) and B7. 1 (CD80). In addition, endothelial expression of P-selectin (CD62P) and VCAM-1 (CD106) also contribute to the inflammatory response to renal ischemia, but the precise anatomical location is not known. Inactivation of ICAM-1 and selectins via antibodies, antisense oligonucleotides, or transgenic knockout ameliorates inflammation and injury after acute ischemia.
Furthermore, macrophage infiltration into the ischemic kidney is regulated by MCP 1, a chemokine that attracts macrophages are expressed by the ischemic kidneys. Transgenic knockout of the receptor for MCP 1 (CCR2) or administration of a truncated, inhibitory form of MCP 1 both ameliorate ischemic injury and inflammation.
In addition, macrophages may be recruited by molecules released by necrotic or apoptotic cells. This will be discussed in later sections of this chapter. Finally, blocking B7 on ischemic endothelia decreases macrophage infiltration and ischemic renal injury. CD28, the ligand for B7, is not known to be expressed by macrophages, but is expressed by T cells. This suggests a role for T cells in ischemic acute renal failure (see below).
Neutrophils : In contrast to the early infiltration of macrophages, some studies report that there is a later infiltrate of neutrophils. The role of these neutrophils is not clear. Early reports suggested that elimination of these neutrophils with antibodies ameliorated ischemic injury. But this may have reflected the use of polyclonal antibodies that actually recognized both neutrophils and macrophages. Recent data using monoclonal antibodies for neutrophils are controversial. Some, but not others, find that deleting neutrophils ameliorated ischemic injury. One difficulty in these studies is the use of the monoclonal antibody for Ly6C/G (Gr1). Although Ly6C/G is highly expressed on neutrophils, it is also expressed, albeit weakly, on some subsets of monocyte/ macrophages.
Renal parenchymal cells produce the neutrophil chemokines KC and MIP 2, the murine analogs for human interleukin 8, as well G-CSF that would stimulate neutrophil production by the bone marrow. In one study, antibody to the neutrophil chemokines KC and MIP 2 decreased neutrophilic infiltration and also ameliorated ischemic injury. However, antibody to the receptor for these chemokines CXCR2 unexpectedly exacerbated injury. These results need to be reconciled. One possibility is that these chemokines have both maladaptive and adaptive functions; in addition to regulating neutrophilic inflammation, KC and MIP 2 may also regulate the differentiation of renal tubular cells during the repair process after injury.
Those advocating a role for neutrophils point out that, in addition to releasing toxic molecules that might injure the kidney, neutrophils are now known to produce cytokines, chemokines, and other regulatory molecules. By producing these molecules, neutrophils may regulate any subsequent inflammation and repair. Those, who find no role for neutrophils in renal injury, might argue that the presence of neutrophils in tissue does not necessary indicate that these neutrophils are activated. Thus, extopic gene expression of the neutrophil chemokine KC results in a neutrophilic infiltrate but no tissue damage, presumably because the neutrophils are not activated to produce toxic molecules. This neutrophil infiltration may be regulated by NK T cells.
Lymphocytes : Small numbers of T cells are found in kidneys after renal ischemia. The role of these T cells is not understood. On the one hand, elimination of T cells via the foxn1 mutation (nude mice) ameliorated injury. FTY720, an immunosuppressive drug that traps lymphocytes in lymph nodes, inhibits ischemic acute renal injury. But, on the other hand, elimination of all classical T cells via mutation of the TCRα chain or via mutation of the rag gene (scid mice) did not inhibit. There is further controversy when elimination of specific subsets of T cells was examined. On the one hand, eliminating CD4 T cells with monoclonal antibodies did not ameliorate injury, but elimination with transgenic knockout did. Similar controversy surrounds the role of CD8 T cells. On the one hand, transgenic knockout of CD8 did not ameliorate ischemic injury; however, anti-CD8 antibodies used in combination with anti-CD4 antibodies did. B-lymphocytes may also contribute to ischemic AKI. This is discussed further in the section on complement and “natural” antibodies.
The role of lymphocytes in ischemic injury is further complicated by observations suggesting that T cells ameliorate injury in some models. These studies fall into three groups: those involving CD4 T cells, those involving interferon gamma, and those involving “unconventional” γδ T cells.
Some CD4 T cells may ameliorate injury. CD4 knockout results in decreased HGF production and increased tubular apoptosis after ureteral obstruction. Nude mice with no classical T cells have increased injury after optic nerve injury, and injection of such T cells improves repair. CD4 T cells play a dual role of exacerbating and inhibiting inflammation after ischemic hepatic injury.
Interferon gamma may play a dual function. Interferon gamma is a cytokine associated with ischemic injury, and produced in quantity by T cells. Interferon gamma exacerbates ischemic acute renal failure in some models. However, there are interferon gamma dependent pathways of tissue repair. Whether or not there are such pathways in the kidney is not known.
Recent data suggest that unconventional T cells recognize injured tissues. Thus, some T cells with γδ T cell receptors recognized stressed epithelia and release keratinocyte growth factors that facilitate repair. Whether or not such T cells are present in the ischemic kidney is not known. NK T cells also participate in ischemic AKI and some NKT cell regulate inflammation during AKI.
The Proinflammatory Effects of Injury—Damps, Sterile Inflammation, and the “Danger/ Damage” Hypothesis
Although the above shows that the inflammatory response to ischemia may an important determinant of the extent of injury and repair, how ischemic injury is translated into inflammation is a major outstanding question. Approaches to addressing this question are reviewed in the remainder of this chapter. Several concepts and terms need to be defined before beginning our discussion. A major recent concept is that injury itself is proinflammatory. The proinflammatory signals are generated in several ways. Molecules, normally residing within cells, elicit inflammation when they are released into the extracellular space or are expressed on cell surfaces. In addition, enzymes released by injured cells or leukocytes convert extracellular matrix molecules into proinflammatory signals. Finally, intracellular stress may generate proinflammatory signals. Altogether these proinflammatory molecules have been called “danger (or damage)-associated molecular pattern” molecules (DAMPS) or Alarmins. This inflammatory response to injury has been called the “danger” response or “sterile” inflammation to differentiate it from the inflammatory response to infections.
The biology of DAMPS, their receptors, and their regulation of injury, of the inflammatory response to injury, and of repair remain to be completely elucidated. The biology is complex because the receptors are promiscuous and interact with numerous DAMPS, because each DAMP interacts with multiple receptors, because DAMPS interact with each other, and because the cellular response to a DAMP is unique to the cell and its microenvironment. We review our current understanding of this rapidly evolving field with the humble realization that this understanding will certainly change in the future.
TLR4 and HMGB1
During ischemic AKI, the best characterized receptor for DAMPS is TLR4. TLR4 is best known as the receptor for endotoxin produced by gram negative bacteria, and mediates the inflammatory response against these bacteria. It is one member of a family of “pattern recognition receptors” that recognize molecules produced by pathogens. In addition to endotoxin, these molecules include viral DNA, viral RNA, and sugar molecules unique to yeast. The TLR’s are present on most leukocytes of the “innate” immune response—macrophages and neutrophils. They are critical for the immediate inflammatory response against infections.
A major discovery was the insight that TLR4 not only recognizes endotoxin, but also recognizes DAMPS. These molecules are called “endogenous” because they are produced by mammalian cells and to differentiate them from endotoxin, the “exogenous” TLR4 ligand that is produced by gram negative bacteria.
Striking confirmation for the importance of TLR4 in ischemic disease were experiments comparing inflammation and injury in wildtype mice versus TLR4 deficient mutant mice after ischemia to the heart, liver, lung, and kidney. In all of these studies, mutant mice with non-functional TLR4 are protected from ischemic injury. Furthermore, human mutations that inactivate TLR4 ameliorate post-transplant ischemic AKI.
HMGB1 is released from injured cells and is the best documented DAMP ligand for TLR4 during ischemic AKI. The HMGB1-TLR4 interaction is one of the few DAMP-TLR4 interactions that have been confirmed by biophysical studies. Furthermore, HMGB1 has a proven role in ischemic AKI. Its expression increases in both murine ischemic AKI, and biopsies of human renal transplant grafts that had ischemic AKI during the transplant process. Antibodies that inactivate HMGB1 ameliorate murine ischemic AKI. Altogether this data show HMGB1 has maladaptive functions during ischemic AKI.
Within four hours reperfusion, reactive oxygen species produced during ischemia reperfusion increase endothelial expression of TLR4. HMGB1 released from injured renal tubulesbind the endothelial TLR4, and cause increased adhesion molecule expression. These adhesion molecules facilitate the immigration of leukocytes into the injured outer medulla. HMGB1 binds to TLR4 on these leukocytes, including macrophages, and stimulates the production of maladaptive interleukin 6. In addition, the HMGB1 also stimulates TLR4 on tubules and stimulates the production of maladaptive chemokines and cytokines. See review and Figure 88.2 .

The Complex Biology of HMGB1 and TLR4—Promiscous Molecules with Promiscous Partners and Multiple Biologic Effects
Although the HMGB1–TLR4 interaction in ischemic AKI is well documented in the above experiments, much remains to be learned.
The biology of HMGB1 is complex. HMGB1 is expressed by all eukaryotic cells and is highly conserved through evolution. It was originally described as a nuclear protein that enables interactions between DNA and nuclear proteins that regulate transcription. However, in the late 1990’s a search for mediators of shock revealed that HMGB1 elicited lethal inflammation. Antibodies against HMGB1 prevented shock. Subsequent experiments showed that HMGB1 was released by necrotic cells, and actively secreted by leukocytes of the innate immune response. The little inflammation seen after apoptosis, as opposed to necrosis, may result from sequestration of HMGB1 within the nucleus of apoptotic cells. The various protein domains HMGB1 have unique functions as shown in Figure 88.3 . The A and B boxes bind to DNA, and the C box is negatively charged. The proinflammatory effect of HMGB1 may be reproduced by recombinant B box. Recombinant A box peptide is a specific antagonist of the proinflammatory effects. Thus, there is therapeutic potential in using these genetically engineered peptides to either increase or decrease inflammation.

HMGB1 is post-translationally modified both enzymatically and by reactive oxygen species. It also binds endotoxin and nucleic acids. These may change its biological activity.
HMGB1 has seven known receptors in addition to TLR4. In addition to TLR4, only one other receptor, RAGE, has been studied in ischemic AKI. RAGE is thought not to participate in ischemic AKI in mice because its transgenic knockout does not affect disease.
The biology of TLR4 is also complex. In addition to HMGB1 it binds to other DAMPS. These include oxidation products resulting from reactive oxygen species produced during ischemia reperfusion, extracellular matrix components and heat shock proteins as discussed below.
After tissue injury, the extracellular matrix is degraded into low molecular weight fragments. Two of these fragments, heparan sulfate and hyaluronan, activate TLR4, and may participate in ischemic AKI. Low molecular weight heparan sulfates are released when neutrophil elastase degrades heparan sulfate proteoglycans in the extracellular matrix (see review ). Inhibition of neutrophil elastase ameliorated ischemic acute renal failure in rodents, possibily by inhibiting the production of heparan sulfate fragments. Hyaluronan increases in the ischemic kidney. Low molecular weight hyaluronans are released when hyaluronidases from tubules and leukocyes degrade the extracellular matrix. Small hyaluronans stimulate renal tubular cells to produce MCP-1 (a macrophage chemokine), and TNFα (a proinflammatory cytokine) in vitro . Biglycan is another extracellular matrix component that may stimulate TLR4 after tissue injury.
Heat shock proteins (Hsp’s) are major candidates for being the TLR4 ligands during ischemic AKI: different intracellular and extracellular functions during ischemic AKI?
Hsp70. 1 and Hsp70. 3 are two members of the murine heat-shock-70 family. They are over 95% identical to each other. Gp96 is the heat shock protein of the endoplasmic reticulum. The murine and human Hsp’s are highly homologous.
Although these Hsp’s have well known intracellular cytoprotective effects, they also have extracellular functions as cytokines, and particularly as TLR4-ligands. Indeed, Hsp’s have been used as adjuvants in an attempt to immunize experimental subjects against cancer.
Intracellular Heat Shock Proteins are Cytoprotective
Hsp’s70. 1 and 70. 3 expressions in the nucleus and cytoplasm is increased after cell stress. Gp96 expression is increased as well. These proteins have also been implicated in renal ischemic injury. Within one hour of renal ischemia, Hsp’s70. 1 and 70.3 increase by 5x in the S3 segment of the proximal tubule, and gp96 increases in both the S3 segment and the loop of Henle. Therefore, increased intracellular Hsp’s70. 1 & 3 and gp96 may be part of a cytoprotective mechanism during ischemic ARF (for example and see reviews ).
Extracellular heat shock proteins may be endogenous TLR4-ligands that recruit inflammatory responses during ischemic ARF.
There is also mounting evidence that extracellular Hsp’s act as cytokines, and the term “chaperokine” was coined to highlight their dual functions. Most importantly, extracellular Hsp’s activate TLR4 to recruit an inflammatory response. The possibility that extracellular Hsp’s may have a harmful pro-inflammatory role in ARF was proposed in a theoretical paper.
Source of Extracellular Hsp’s
Since Hsp’s are normally intracellular proteins, the question of how Hsp’s are released into the extracellular space during injury needs to be addressed. There are several possibilities.
Firstly, leakage from cells with morphologically evident injury (necrosis). Hsp’s may be released from severely injured and therefore leaky cells. Since Hsp’s are abundant proteins, and their cytokine functions are likely to require very low extracellular concentrations, a small amount of leakage may trigger the self-amplifying inflammatory mechanisms that induce further injury during the “extension” phase of ischemic ARF. Such necrosis would result in the release of intracellular heat shock proteins into the extracellular space where they could activate the endothelium and leukocytes.
Secondly, release from cells without evident morphologic injury. Several such mechanisms have been proposed: One is the secretion of Hsp’s by stressed cells or cells activated by cytokines. A second mechanism is the release of “exosomes.” Exosomes are formed by a process of “reverse” endocytosis. These small particles contain Hsp’s and they activate macrophages and dendritic cells (see review ). A third mechanism is the expression of membrane bound Hsp’s. Although Hsp’s normally reside in the cytoplasm, endoplasmic reticulum, or mitochondria, they may redistribute to the cell surface after stress. Such cell surface Hsp’s activate leukocytes. Altogether there is ample evidence to support the possibility that Hsp’s from injured renal tubular cells could stimulate TLR4 in the absence of morphologic features of cell death that are detectable by light microscopy.
Other endogenous molecules also interact with TLR4, but they are less well studied than HMGB1, Hsp’s and extracellular matrix components discussed above. Fibronectin IIIA is a variant fibronectin that is produced by stressed cells, and is increased during ischemic ARF. β-defensin is found in kidneys stressed by infection, but β-defensin production during ischemic ARF has not previously been examined. Hsp60 is not known to increase after renal ischemia, but might still be released during ischemic ARF and stimulate TLR4. Tamm Horsfall protein may also be a TLR4 ligand. See review. Not only does TLR4 bind to numerous ligands, each ligand may trigger unique biological effects.
Thus, endotoxin and the endogenous TLR4-ligands have different interactions with the TLR4 receptor. Endotoxin interacts with TLR4 in two ways: first, soluble endotoxin may bind the TLR4-MD2 heterodimer. Second, endotoxin may form lipid micelles that intercalate into cell membrane and then bind TLR4. In contrast, all of the endogenous TLR4 ligands identified so far are soluble molecules. Although the hydrophobicity of these ligands may be important, they are unlikely to directly intercalate into the membrane bilayer. Furthermore, some endogenous TLR4 ligands also bind other cell surface receptors in addition to activating TLR4. For example: Hsp’s 70. 1 & 3 and gp96 bind cell surface CD40 and CD91.
Because endotoxin and the endogenous TLR4-ligands have different modes of interaction with the TLR4 receptor, it is not surprising that they elicit different responses in vitro and in a model of autoimmunity in vivo .
Of particular importance for this discussion is the different effect of endotoxin and ischemia on the kidney. Both injure the kidney, but in different ways. When care is taken to prevent hypotension, endotoxin decreases GFR by over 70% in the absence of apoptosis, inflammation, and other morphological changes; this injury is not affected by transgenic knockout of inducible nitric oxide synthase (iNOS). In contrast, murine ischemic ARF, which is accompanied by the release of endogenous TLR4-ligands, is characterized by profound morphologic injury and inflammation (see for example review ). Unlike normotensive endotoxin ARF, ischemic ARF is ameliorated by transgenic knockout of iNOS.
When hypotension is not controlled, endotoxin causes glomerular, in addition to tubular, injury. In contrast, ischemic ARF with release of endogenous ligands, injures tubules, but not glomeruli. Other data shows that systemic endotoxin injures the kidney by increasing the production of extrarenal TNFα.
Note that ARF induced by endotoxin differs from ischemic ARF in another fundamental way: endotoxin is injected systemically and induces massive cytokine production in many organs; on the other hand, during ischemia the postulated TLR4 ligands are released locally in the kidney.
Another example of differences between endotoxin and endogenous TLR4 ligands is a model of autoimmune isletis where endotoxin alone induced diabetes, but Hsp’s 70.1 and 70.3 (both endogenous TLR4 ligands) required additional activating anti-CD40 to induce diabetes. Still another relevant example is the TLR4-dependent abilities of LPS to decrease, but HMGB1, another TLR4 ligand, to increase, the carcinogenicity of croton oil.
In addition to differences between the response of TLR4 to endotoxin versus DAMPS, individual DAMPS may each elicit a unique response through TLR4.
As discussed above, TLR4 recognizes both DAMPs and endotoxin produced by bacteria. Microbial products, such as endotoxin, have hydrophobic domains; these domains are also found on proteins denatured during cellular injury, and on DAMPs such as HMGB1 that are released into the extracellular space. Because DAMP receptors appeared early during evolution, they may have evolved to recognize injury, organize repair, and then later acquired a host-defense role by recognizing microbes. However, the responses to infection and repair have different goals. After recognizing microbes, TLR4 triggers an aggressive inflammatory response where elimination of the pathogen is the overwhelming priority, and collateral damage to self tissue by toxic microbicidal molecules is an acceptable price for survival of the organism; ultimately, repair occurs after cure of the infection. In contrast, sterile injury such as ischemia/ reperfusion, has tissue repair as the priority. The initial maladaptive inflammation may reflect the fact that tissue injury and infection often occur together, especially after trauma and disruption of the skin. A “shoot first, ask questions later” strategy may have evolved because an initial inflammatory response against the possibility of infection best served survival despite some collateral tissue damage. A fundamental question is how, after either infection or sterile injury, the mononuclear phagocytes change from aggressively proinflammatory (maladaptive for ischemic injury) to reparative. This question may be answered, at least in part, by the presence of CD24-Siglec 10 on mammalian but not bacterial cel surfaces. CD24/ Siglec 10 binds DAMPSand turns off the TLR4 response. Bacteria do not express CD24/Siglec 10 and the TLR4 response would therefore not be inhibited. See Figure 88.4 .

Less widely appreciated are recent studies showing that TLR4 participates in repair of non-infectious colitis. Some types of non-infectious pulmonary injury, skin wounding and partial hepatectomy. Whether TLR4 has similar repair functions in the kidney is not known.
Other Multi-Ligand Receptors for other Multi-Receptor DAMPS may Detect Ischemic Injury?
In addition to TLR4, a number of other receptors recognize DAMPS. These recognize injury by a number of pathways including the detection of reactive oxygen species, uric acid, glycolipids, and intracellular stress. These receptors include
CD91 : This is also a receptor for heat shock proteins released by injured cells. It has been targeted as a means of increasing immunity against tumors. In addition to binding heat shock proteins, CD91 also binds α2-macroglobulin, collectin and calreticulin, and is known as LDL receptor related protein. These recognize injury by a number of pathways including the detection of reactive oxygen species, uric acid, glycolipids, and intracellular stress.
TLR2 : TLR2 is related to TLR4 and may also recognize Hsp’s. Mice with non-functional TLR2 are protected from ischemic renal failure. Renal tubules express both TLR2 and TLR4 after severe ischemic injury. TLR2 may also be important in ischemic injury to the liver and heart.
RAGE : Although RAGE is best known as the receptor for advanced glycation endproducts and for its contribution to the secondary complications of diabetes mellitus, including diabetic nephropathy, RAGE also detects molecules released by injured cells, and triggers an inflammatory response.
Uric acid : Weibel Paladi bodies, NALP3, and Pyronecrosis. Uric acid is released from injured cells. During ischemic AKI this may induce endothelia to release proinflammatory Weibel-Palade bodies. Uric acid may also activate Nlrp3, possibly by activating the inflammasome and triggering pyronecrosis.
Complement : Renal IRI does activate complement. Tubular injury decreases expression of basolateral expression of Crry, the murine homolog of human MCP (membrane cofactor protein) and DAF (decay accelerating factor); Crry normally prevents amplification of the alternative complement pathway after “C3 tickover”. In the absence of basolateral Crry during renal IRI, alternative complement activation continues unrestrained and renal tubular injury results.
Why there should be so many ways to detect molecules released by injured cells is not clear. Do all injured cells release the same molecules, are different molecules released after different types of death and injury, do different molecules and receptors elicit different inflammatory responses? In very broad strokes, we will discuss the different modes of programmed cell death and the different inflammation elicited by different types of death later in the chapter. However, a profound understanding of these questions remains to be elucidated by future research.
When Death is no Accident: Necrosis as a Programmed Event
The above section shows that molecules released from necrotic cells elicit an inflammatory response. Necrosis is often considered accidental death. However, a growing body of data indicates that necrosis may also be a programmed event. This suggests that when inflammation is desirable, a cell may be programmed to die a necrotic death, and thus release the pro-inflammatory molecules discussed above.
Poly (ADP-Ribose) Polymerase [PARP] and Programmed Necrosis
The PARP’s are a family of 18 genes. PARP-1 regulates necrosis. That an enzyme regulates necrosis indicates that death is no accident, but is programmed. Pharmacologic inhibition of PARP ameliorates ischemic acute renal injury in rodents. Transgenic knockout of PARP-1 also decreases injury after acute renal ischemia. Inhibition of PARP-1 also ameliorates ischemic injury of the brain and liver.
The best known function of PARP-1 is to repair DNA damage, such as occurs in response to oxidative stress during ischemic acute renal failure. Renal PARP-1 levels increase during ischemic acute renal failure.
It is not intuitively obvious why such a repair enzyme should be required for necrosis. One possibility is that, in the face of massive DNA damage, PARP depletes intracellular NAD + and thus ATP stores. This leads to necrosis, especially in the setting of mitochondrial damage as discussed later in this section. However, necrosis is not necessarily correlated with intracellular energy stores in all model systems. Another possibility is that PARP-1 enhances the activity of NFκB and other pro-inflammatory transcription factors. PARP may also increase mitochondrial release of AIF.
Some suggest that caspases degrade PARP and thus direct cell death down an apoptotic pathway. However, there is decreased ischemic acute renal injury in mice expressing a genetically engineered PRAP-1 that cannot be degraded by caspases.
Cyclophilin D, Mitochondria, and Programmed Necrosis
Another argument that necrosis is regulated comes from studies of mice with transgenic knockout of cyclophilin D. Such mice have decreased necrosis during ischemic acute renal failure. Cerebral ischemia was similarly ameliorated in these knockout animals. These results extend data that cyclosporine, by inhibiting cyclophilin D, ameliorates ischemic injury in some tissues.
Cyclophilin D regulates the mitochondrial permeability transition pore, and the subsequent release of mitochondrial molecules that regulate cell death. The above data suggest an important role for mitochondria in regulating necrosis. Whether opening this pore results in necrosis or apoptosis may depend upon several factors. One is the length of time that the pore is open—transient opening might result in apoptosis; longer opening, necrosis. In addition the availability of ATP may switch the mitochondrial signal from necrosis to apoptosis. This is in line with data showing that lower, more prolonged decreases in ATP are associate with necrosis, while shorter and lesser ATP depletion result in necrosis in renal cells, and that lesser oxidant injury also leads to apoptosis instead of necrosis. Finally, intracellular pH also regulates. The return of the pH from acidic to more alkaline levels with reperfusion makes necrosis more likely.
Additional Examples of Programmed Necrosis in Vivo
We will now review three additional striking examples of the importance of programmed necrosis in vitro .
One example is the host defense against murine vaccinia virus. This virus protects itself by preventing apoptotic programs within infected host cells. In mice with wild type TNFR2, infected cells die by programmed TNFα-mediated necrosis, and elicit a protective inflammatory response. Mice with TNFR2 knockout have a reduced programmed necrosis and thus reduced anti-viral inflammatory response and decreased viral clearance.
The second example is the difference between cerulean pancreatitis in rats versus mice. The worse outcome in the latter is due to greater programmed necrosis. Rats have high apoptosis and low necrosis and thus a better clinical outcome. Mice have low apoptosis and high necrosis and thus a worse outcome with more inflammation. This difference was due to different function of the X-linked inhibitor of caspases (XIAP) in these two species. There was less inhibition of caspases, and thus less inhibition of apoptosis, in the rat by XIAP.
The third example is the exacerbation of shock when apoptosis is inhibited in mice given TNFα. In this case, switching programmed cell death from apoptosis to necrosis had fatal consequences.
After the Suicide, Disposal of the Corpse: Regulation of Inflammation by Macrophages after they Phagocytose Apoptotic Cells
Apoptosis occurs during ischemic acute renal failure. The goal of this discussion is not the regulation of this apoptosis but rather the effect of apoptosis on inflammation. In other words, we discuss phagocytic clearance of the apoptotic cells before their loss of membrane integrity and leakage of the proinflammatory molecules discussed in the previous section. Such clearance is regulated by “eat me,” “don’t eat me,” “come get me” signals.
The surface of the apoptotic cell has “eat me” signals that trigger phagocytosis by macrophages. A major signal is phosphatidylserine that has somehow “flipped” from the intracellular leaflet to the extracellular leaflet of the plasma membrane where it is recognized by macrophage receptors including the phosphatidylserine receptor after bridging by Annexin I. Other less well understood interactions between apoptotic cell and macrophage also contribute to the “eat me” signal. These include sites also capable of binding collectins such as mannose binding protein, C1q, C3b/bi, oxidized LDL, and thrombospondin 1. In addition, the apoptotic cell surface has decreased “don’t eat me” signals such as CD31. Furthermore, phosphatidylcholine on apoptotic cell surfaces is cleaved by phospholipase A2 to form lysophosphatidyl choline which is the best understood chemoattractant “come get me” signal issued by apoptotic cells to macrophages. [See review ].
Under many circumstances, macrophages, which have engulfed apoptotic cells, release anti-inflammatory molecules that prevent further inflammation (for example ). The phosphatidylserine receptor on macrophages may trigger the release of inhibitory cytokines, but this begs the question of why this receptor is not triggered when macrophage phagocytose necrotic debris, including phosphatidylserine on the intracellular side of cell membrane fragments. In the absence of a receptor for phosphatidylserine, macrophages cannot ingest apoptotic cells, and the lungs of such mice fill with cellular debris and inflammation. This may reflect the consequences of overwhelming the phagocytotic system with too many apoptotic corpses as perhaps occurs during ischemic acute renal failure. This situation may reflect “post-apoptotic necrosis” and the release of proinflammatory mediators.
However, there are a number of experimental circumstances where phagocytosis of apoptotic cells results in the release of pro-inflammatory molecules by macrophages, and where ingestion of necrotic debris results in the release of anti-inflammatory molecules. This may reflect the influence of cytokines in the microenvironment, or the redox potential of the microenvironment that can oxidize phospholipids and turn them into macrophage activating signals.
Efferocytosis is the phagocytosis of apoptotic cells and does not cause the release of proinflammatory cytokines. If efferocytosis cannot dispose of all the apoptotic cells, then some will degenerate and release DAMPS. Increasing efferocytos by injections of MFG-E8 (milk fat globule-EGF factor 8/ lactadherin) ameliorates ischemic AKI in rodents; MFG-E8 is a “bridging molecule” that links the apoptotic cells to phagocytes, and thus increases efferocytosis.
References
- [1]. Havasi A., and Borkan S.C.: Apoptosis and acute kidney injury. Kidney Int 2011; undefined:
- [2]. Legrand M., Mik E.G., Johannes T., Payen D., and Ince C.: Renal hypoxia and dysoxia after reperfusion of the ischemic kidney. Mol Med 2008; 14: pp. 502-516
- [3]. Feldkamp T., Park J.S., Pasupulati R., Amora D., Roeser N.F., Venkatachalam M.A., et al: Regulation of the mitochondrial permeability transition in kidney proximal tubules and its alteration during hypoxia-reoxygenation. Am J Physiol Renal Physiol 2009; 297: pp. F1632-F1646
- [4]. Brooks C., Wei Q., Cho S.G., and Dong Z.: Regulation of mitochondrial dynamics in acute kidney injury in cell culture and rodent models. J Clin Invest 2009; 119: pp. 1275-1285
- [5]. Basile D.P., Friedrich J.L., Spahic J., Knipe N.L., Mang H.E., Leonard E.C., et al: Impaired endothelial proliferation and mesenchymal transition contribute to vascular rarefaction following acute kidney injury. Am J Physiol Renal Physiol 2011; 2011: pp. F721-F733
- [6]. Goligorsky M.S., Patschan D., and Kuo M.C.: Weibel-Palade bodies–sentinels of acute stress. Nat Rev Nephrol 2009; 5: pp. 423-426
- [7]. Schrier R.W., Wang W., Poole B., and Mitra A.: Acute renal failure: definitions, diagnosis, pathogenesis, and therapy. J Clin Invest 2004; 114: pp. 5-14
- [8]. Lin S.L., Li B., Rao S., Yeo E.J., Hudson T.E., Nowlin B.T., et al: Macrophage Wnt7b is critical for kidney repair and regeneration. Proc Natl Acad Sci U S A 2010; 107: pp. 4194-4199
- [9]. Seong S.Y., and Matzinger P.: Hydrophobicity: an ancient damage-associated molecular pattern that initiates innate immune responses. Nat Rev Immunol 2004; 4: pp. 469-478
- [10]. Gallucci S., and Matzinger P.: Danger signals: SOS to the immune system. Curr Opin Immunol 2001; 13: pp. 114-119
- [11]. Matzinger P.: The danger model: a renewed sense of self. Science 2002; 296: pp. 301-305
- [12]. Matzinger P.: Tolerance, danger, and the extended family. Annual Review Immunology 1994; 12: pp. 991-1045
- [13]. Medzhitov R., and Janeway C.: Innate immunity. N Engl J Med 2000; 343: pp. 338-344
- [14]. Halloran P.F., Homik J., Goes N., Lui S.L., Urmson J., Ramassar V., et al: The “injury response”: a concept linking nonspecific injury, acute rejection, and long-term transplant outcomes. Transplant Proc 1997; 29: pp. 79-81
- [15]. Nadeau K.C., Azuma H., and Tilney N.L.: Cytokines in the pathophysiology of acute and chronic allograft rejection. Transplant Rev 1996; 10: pp. 99-107
- [16]. Orosz C.G., Bergese S.D., Wakely E., Xia D., Gordillo G.M., and VanBuskirk A.M.: Acute versus chronic graft rejection: related manifestations of allosensitization in graft recipients. Transplant Rev 1997; 11: pp. 38-50
- [17]. Samaniego M., Baldwin W.M., and Sanfilippo F.: Delayed graft function: immediate and late impact. Curr Opin Nephrol Hypertens 1997; 6: pp. 533-537
- [18]. Land W., and Messmer K.: The impact of ischemia/ reperfusion injury on specific and non-specific, early and late chronic events after organ transplantation. Transplant Rev 1996; 10: pp. 108-127
- [19]. Land W.G.: Emerging role of innate immunity in organ transplantation Part II: potential of damage-associated molecular patterns to generate immunostimulatory dendritic cells. Transplant Rev (Orlando) 2011; undefined:
- [20]. Lu C.Y., Penfield J.G., Kielar M.L., Vazquez M.A., and Jeyarajah D.R.: Hypothesis: is renal allograft rejection initiated by the response to injury sustained during the transplant process? Kidney Int 1999; 55: pp. 2157-2168
- [21]. Boros P., and Bromberg J.S.: New cellular and molecular immune pathways in ischemia/reperfusion injury. Am J Transplant 2006; 6: pp. 652-658
- [22]. LaRosa D.F., Rahman A.H., and Turka L.A.: The innate immune system in allograft rejection and tolerance. J Immunol 2007; 178: pp. 7503-7509
- [23]. Kim I.K., Bedi D.S., Denecke C., Ge X., and Tullius S.G.: Impact of innate and adaptive immunity on rejection and tolerance. Transplantation 2008; 86: pp. 889-894
- [24]. Tuttle K.R.: Linking metabolism and immunology: diabetic nephropathy is an inflammatory disease. J Am SocNephrol 2005; 16: pp. 1537-1538
- [25]. Remuzzi G., and Bertani T.: Pathophysiology of progressive nephropathies. N Engl J Med 1998; 339: pp. 1448-1456
- [26]. Rodriguez-Iturbe B., Vaziri N.D., Herrera-Acosta J., and Johnson R.J.: Oxidative stress, renal infiltration of immune cells, and salt-sensitive hypertension: all for one and one for all. AJP – Renal Physiol 2004; 286: pp. F606-F616
- [27]. Nigam S.K., Lieberthal W., Hammerman M.R., Safirstein R., and Harris R.C.: Acute renal failure. III. The role of growth factors in the process of renal regeneration and repair. Am J Physiol Renal Physiol 2000; 279: pp. F3-F11
- [28]. Molitoris B.A., Weinberg J.M., Venkatachalam M.A., Lieberthal W., Nigam S.K., Zager R.A., et al: Acute renal failure. II. Experimental models of acute renal failure: imperfect but indispensable. Am J Physiol 2000; 278: pp. F1-F12
- [29]. Lieberthal W., Nigam S.K., Bonventre J.V., Brezis M., Siegel N., Rosen S., et al: Acute renal failure. I. Relative importance of proximal vs. distal tubular injury. Am J Physiol 1998; 275: pp. F623-F631
- [30]. Marcussen N., Lai R., Olsen T.S., and Solez K.: Morphometric and immunohistochemical investigation of renal biopsies from patients with transplant ATN , native ATN , or acute graft rejection. Transplant Proc 1996; 28: pp. 470-476
- [31]. Jaber B.L., Pereira B.J., Bonventre J.V., and Balakrishnan V.S.: Polymorphism of host response genes: implications in the pathogenesis and treatment of acute renal failure. Kidney Int 2005; 67: pp. 14-33
- [32]. Hoffmann S.C., Kampen R.L., Amur S., Sharaf M.A., Kleiner D.E., Hunter K., et al: Molecular and immunohistochemical characterization of the onset and resolution of human renal allograft ischemia-reperfusion injury. Transplantation 2002; 74: pp. 916-923
- [33]. Koo D.D., and Fuggle S.V.: Impact of ischemia/ reperfusion injury and early inflammatory responses in kidney transplantation. Transplant Rev 2000; 14: pp. 210-224
- [34]. Koo D.D., Welsh K.I., Roake J.A., Morris P.J., and Fuggle S.V.: Ischemia/reperfusion injury in human kidney transplantation: an immunohistochemical analysis of changes after reperfusion. Am J Pathol 1998; 153: pp. 557-566
- [35]. Turunen A.J., Lindgren L., Salmela K.T., Kyllonen L.E., Makisalo H., Siitonen S.M., et al: Association of graft neutrophil sequestration with delayed graft function in clinical renal transplantation. Transplantation 2004; 77: pp. 1821-1826
- [36]. Gaber L.W., Gaber A.O., Tolley E.A., and Hathaway D.K.: Prediction by postrevascularization biopsies of cadaveric kidney allografts of rejection, graft loss, and preservation nephropathy. Transplant 1992; 53: pp. 1219-1225
- [37]. Avihingsanon Y., Ma N., Pavlakis M., Chon W.J., Uknis M.E., Monaco A.P., et al: On the intraoperative molecular status of renal allografts after vascular reperfusion and clinical outcomes. J Am SocNephrol 2005; 16: pp. 1542-1548
- [38]. Hauser P., Schwarz C., Mitterbauer C., Regele H.M., Muhlbacher F., Mayer G., et al: Genome-wide gene-expression patterns of donor kidney biopsies distinguish primary allograft function. Lab Invest 2004; 84: pp. 353-361
- [39]. Schwarz C., Regele H., Steininger R., Hansmann C., Mayer G., and Oberbauer R.: The contribution of adhesion molecule expression in donor kidney biopsies to early allograft dysfunction. Transplantation 2001; 71: pp. 1666-1670
- [40]. Araki M., Fairchild R., et al: The clinical impact of chemokine and receptor gene expression during ischemia/reperfusion injury in renal allografts. Am J Transpl 2004; 8: pp. 74
- [41]. Pratschke J., Wilhelm M.J., Kusaka M., Basker M., Cooper D.K., Hancock W.W., et al: Brain death and its influence on donor organ quality and outcome after transplantation. Transplant 1999; 67: pp. 343-348
- [42]. Porter K.A., and Heptinstall R.H.: Renal transplantation. Boston: Little, Brown & Co., 1992.
- [43]. Rosen S., and Stillman I.E.: Acute tubular necrosis is a syndrome of physiologic and pathologic dissociation. J Am Soc Nephrol 2008; 19: pp. 871-875
- [44]. Venkatachalam M.A., Griffin K.A., Lan R., Geng H., Saikumar P., and Bidani A.K.: Acute kidney injury: a springboard for progression in chronic kidney disease. Am J Physiol Renal Physiol 2010; 298: pp. F1078
- [45]. Kelly K.J., and Dominguez J.H.: Rapid progression of diabetic nephropathy is linked to inflammation and episodes of acute renal failure. Am J Nephrol 2010; 32: pp. 469-475
- [46]. Kashgarian M.: Acute tubular necrosis an ischemic renal injury. In (eds): , 5th edition. Philadelphia New York: Lippincott – Raven, 1999. pp. 863-889
- [47]. Lucke B.: Lower nephron nephrosis. Mil Surg 1946; 99: pp. 371-396
- [48]. Oliver J., Mac D.M., and Tracy A.: The pathogenesis of acute renal failure associated with traumatic and toxic injury; renal ischemia, nephrotoxic damage and the ischemic episode. J Clin Invest 1951; 30: pp. 1307-1439
- [49]. Solez K.: Pathogenesis of acute renal failure. Int Rev Exp Pathol 1983; 24: pp. 277-333
- [50]. Brezis M., Rosen S., Silva P., and Epstein F.H.: Renal ischemia: a new perspective. Kidney Int 1984; 26: pp. 375-383
- [51]. De Greef K.E., Ysebaert D.K., Persy V., Vercauteren S.R., and De Broe M.E.: ICAM-1 expression and leukocyte accumulation in inner stripe of outer medulla in early phase of ischemic compared to HgCl2-induced ARF. Kidney Int 2003; 63: pp. 1697-1707
- [52]. De Greef K.E., Ysebaert D.K., Dauwe S.E., Persy V.P., Vercauteren S., Mey D., et al: Anti-B7-1 blocks mononuclear cell adherence in vasa recta after ischemia. Kidney Int 2001; 60: pp. 1415-1427
- [53]. Fukuzawa N., Schenk A.D., Petro M., Nonomura K., Baldwin W.M., and Fairchild R.L.: High renal ischemia temperature increases neutrophil chemoattractant production and tissue injury during reperfusion without an identifiable role for CD4 T cells in the injury. Transpl Immunol 2009; 22: pp. 62-71
- [54]. Boutin H., LeFeuvre R.A., Horai R., Asano M., Iwakura Y., and Rothwell N.J.: Role of IL-1alpha and IL-1beta in ischemic brain damage. J Neurosci 2001; 21: pp. 5528-5534
- [55]. Shito M., Wakbayashi G., Ueda M., Shimazu M., Shirasugi N., Endo M., et al: Interleukin 1 receptor blockade reduces tumor necrosis factor production, tissue injury, and mtality after hepatic iscehmia reperfusion in e rat. Transplant 1997; 63: pp. 143-148
- [56]. Haq M., Norman J., Saba S.R., Ramirez G., and Rabb H.: Role of IL-1 in renal ischemic reperfusion injury. J Am Soc Nephrol 1998; 9: pp. 614-619
- [57]. Valentin J.F., Bruijn J.A., and Paul L.C.: Donor treatment with mycophenolate mofetil: protection against ischemia-reperfusion injury in the rat. Transplant 2000; 69: pp. 344-350
- [58]. Ventura C.G., Coimbra T.M., de Campos S.B., de C.I., Yu L., and Seguro A.C.: Mycophenolate mofetil attenuates renal ischemia/reperfusion injury. J Am Soc Nephrol 2002; 13: pp. 2524-2533
- [59]. Geissmann F., Manz M.G., Jung S., Sieweke M.H., Merad M., and Ley K.: Development of monocytes, macrophages, and dendritic cells. Science 2010; 327: pp. 656-661
- [60]. Velazquez P., Dustin M.L., and Nelson P.J.: Renal dendritic cells: an update. Nephron Exp Nephrol 2009; 111: pp. e67-e71
- [61]. Hochheiser K., Tittel A., and Kurts C.: Kidney dendritic cells in acute and chronic renal disease. Int J Exp Pathol 2010; undefined:
- [62]. Mosser D.M., and Edwards J.P.: Exploring the full spectrum of macrophage activation. Nat Rev Immunol 2008; 8: pp. 958-969
- [63]. Geissmann F., Gordon S., Hume D.A., Mowat A.M., and Randolph G.J.: Unravelling mononuclear phagocyte heterogeneity. Nat Rev Immunol 2010; 10: pp. 453-460
- [64]. Jo S.K., Sung S.A., Cho W.Y., Go K.J., and Kim H.K.: Macrophages contribute to the initiation of ischaemic acute renal failure in rats. Nephrol Dial Transplant 2006; undefined:
- [65]. Day Y.J., Huang L., Ye H., Linden J., and Okusa M.D.: Renal ischemia-reperfusion injury and adenosine 2a receptor-mediated tissue protection: the role of macrophages. AJP – Renal Physiol 2004; undefined:
- [66]. Kielar M.L., John R., Bennett M., Richardson J.A., Shelton J.M., Chen L., et al: Maladaptive role of IL-6 in ischemic acute renal failure. J Am Soc Nephrol 2005; 16: pp. 3315-3325
- [67]. Molitoris B.A., and Sutton T.A.: Endothelial injury and dysfunction: role in the extension phase of acute renal failure. Kidney Int 2004; 66: pp. 496-499
- [68]. Basile D.P., Donohoe D., Roethe K., and Osborn J.L.: Renal ischemic injury results in permanent damage to peritubular capillaries and influences long-term function. Am J Physiol Renal Physiol 2001; 281: pp. F887-F899
- [69]. Molitoris B.A., Sandoval R., and Sutton T.A.: Endothelial injury and dysfunction in ischemic acute renal failure. Crit Care Med 2002; 30: pp. S235-S240
- [70]. Li L., and Okusa M.D.: Macrophages, dendritic cells, and kidney ischemia-reperfusion injury. Semin Nephrol 2010; 30: pp. 268-277
- [71]. Lemay S., Rabb H., Postler G., and Singh A.K.: Prominent and sustained up-regulation of gp130 – signaling cytokines and of the chemokine MIP 2 in murine renal ischemia—reperfusion injury. Transplant 2000; 69: pp. 959-963
- [72]. Takada M., Nadeau K.C., Shaw G.D., Marquette K.A., and Tilney N.L.: The cytokine-adhesion molecule cascade in ischemia/ reperfusion injury the rat kidney—inhibition by a soluble P-selectin ligand. J Clin Invest 1997; 99: pp. 2682-2690
- [73]. Patel N.S., Chatterjee P.K., Di Paola R., Mazzon E., Britti D., De Sarro A., et al: Endogenous interleukin-6 enhances the renal injury, dysfunction, and inflammation caused by ischemia/reperfusion. J Pharmacol Exp Ther 2005; 312: pp. 1170-1178
- [74]. Hurst S.M., Wilkinson T.S., McLoughlin R.M., Jones S., Horiuchi S., Yamamoto N., et al: Il-6 and its soluble receptor orchestrate a temporal switch in the pattern of leukocyte recruitment seen during acute inflammation. Immunity 2001; 14: pp. 705-714
- [75]. Henderson R.B., Hobbs J.A., Mathies M., and Hogg N.: Rapid recruitment of inflammatory monocytes is independent of neutrophil migration. Blood 2003; 102: pp. 328-335
- [76]. Maus U.A., Waelsch K., Kuziel W.A., Delbeck T., Mack M., Blackwell T.S., et al: Monocytes are potent facilitators of alveolar neutrophil emigration during lung inflammation: role of the CCL2-CCR2 axis. J Immunol 2003; 170: pp. 3273-3278
- [77]. Maus U.A., Koay M.A., Delbeck T., Mack M., Ermert M., Ermert L., et al: Role of resident alveolar macrophages in leukocyte traffic into the alveolar air space of intact mice. Am J Physiol Lung Cell Mol Physiol 2002; 282: pp. L1245-L1252
- [78]. Gregory S.H., and Wing E.J.: Neutrophil-Kupffer-cell interaction in host defenses to systemic infections. Immunol Today 1998; 19: pp. 507-510
- [79]. Penfield J.G., Wang Y., Li S., Kielar M.L., Sicher S.C., Jeyarajah D.R., et al: Transplant surgery injury recruits recipient MHC class II-positive leukocytes into the kidney. Kidney Int 1999; 56: pp. 1759-1769
- [80]. Penfield J.G., Dawidson I.A., Ar’Rajab A., Kielar M.L., Jeyarajah D.R., and Lu C.Y.: Syngeneic renal transplantation increases the number of renal dendritic cells in the rat. Transpl Immunol 1999; 7: pp. 197-200
- [81]. Shoskes D.A., Parfrey N.A., and Halloran P.F.: Increased major histocompatibility complex antigen expression in unilateral ischemic acute tubular necrosis in the mouse. Transplant 1990; 49: pp. 201-207
- [82]. Heemann U.W., Tullius S.G., Tamatami T., Miyasaka M., Milford E., and Tilney N.L.: Infiltration patterns of macrophages and lymphocytes in chronically rejecting rat kidney allografts. TransplInt 1994; 7: pp. 349-355
- [83]. Duffield J.S., Forbes S.J., Constandinou C.M., Clay S., Partolina M., Vuthoori S., et al: Selective depletion of macrophages reveals distinct, opposing roles during liver injury and repair. J Clin Invest 2005; 115: pp. 56-65
- [84]. Wilson H.M., Walbaum D., and Rees A.J.: Macrophages and the kidney. Curr Opin Nephrol Hypertens 2004; 13: pp. 285-290
- [85]. Park J.E., and Barbul A.: Understanding the role of immune regulation in wound healing. Am J Surg 2004; 187: pp. 11S-16S
- [86]. Saville L.R., Pospisil C.H., Mawhinney L.A., Bao F., Simedrea F.C., Peters A.A., et al: A monoclonal antibody to CD11d reduces the inflammatory infiltrate into the injured spinal cord: a potential neuroprotective treatment. J Neuroimmunol 2004; 156: pp. 42-57
- [87]. Duffield J.S.: The inflammatory macrophage: a story of Jekyll and Hyde. Clin Sci (Lond) 2003; 104: pp. 27-38
- [88]. Humes H.D.: Potential molecular therapy for acute renal failure. Cleve Clin J Med 1993; 60: pp. 166-168
- [89]. Basile D.P., Rovak J.M., Martin D.R., and Hammerman M.R.: Increased transforming growth factor-beta 1 expression in regenerating rat renal tubules following ischemic injury. Am J Physiol 1996; 270: pp. F500-F509
- [90]. Lee S., Huen S., Nishio H., Nishio S., Lee H.K., Choi B.S., et al: Distinct macrophage phenotypes contribute to kidney injury and repair. J Am Soc Nephrol 2011; 22: pp. 317-326
- [91]. Lassen S., Lech M., Rommele C., Mittruecker H.W., Mak T.W., and Anders H.J.: Ischemia reperfusion induces IFN regulatory factor 4 in renal dendritic cells, which suppresses postischemic inflammation and prevents acute renal failure. J Immunol 2010; 185: pp. 1976-1983
- [92]. Afzali B., Mitchell P., Lechler R.I., John S., and Lombardi G.: Translational mini-review series on Th17 cells: induction of interleukin-17 production by regulatory T cells. Clin Exp Immunol 2010; 159: pp. 120-130
- [93]. Mantovani A., Sica A., and Locati M.: Macrophage polarization comes of age. Immunity 2005; 23: pp. 344-346
- [94]. Springer T.A.: Traffic signals for lymphocyte recirculation and leukocyte emigration. Cell 1994; 76: pp. 301
- [95]. Butcher E.C., and Picker L.J.: Lymphocyte homing and homeostasis. Science 1996; 272: pp. 60-66
- [96]. Imhof B.A., and Aurrand-Lions M.: Adhesion mechanisms regulating the migration of monocytes. Nat RevImmunol 2004; 4: pp. 432-444
- [97]. Dustin M.: A supercode for inflammation. Immunity 2004; 20: pp. 361-362
- [98]. Kelly K.J., Williams W.W., Colvin R.B., and Bonventre J.V.: Antibody to intercellular adhesion molecule 1 protects the kidney against ischemic injury. Proc Natl Acad Sci USA 1995; 91: pp. 812-816
- [99]. Rabb H., Mendiola C.C., Saba S.R., Dietz J.R., Smith C.W., Bonventre J.V., et al: Antibodies to ICAM 1 protect kidneys in severe ischemic reperfusion injury. Biochem Biophys Res Commun 1995; 211: pp. 67-73
- [100]. Miura M., Fu X., Zhang Q.W., Remick D.G., and Fairchild R.L.: Neutralization of Gro alpha and macrophage inflammatory protein-2 attenuates renal ischemia/reperfusion injury. Am J Pathol 2001; 159: pp. 2137-2145
- [101]. Burne M.J., Daniels F., El Ghandour A., Mauiyyedi S., Colvin R.B., O’Donnell M.P., et al: Identification of the CD4(+) T cell as a major pathogenic factor in ischemic acute renal failure. J Clin Invest 2001; 108: pp. 1283-1290
- [102]. Nemoto T., Burne M.J., Daniels F., O’Donnell M.P., Crosson J., Berens K., et al: Small molecule selectin ligand inhibition improves outcome in ischemic acute renal failure. Kidney Int 2001; 60: pp. 2205-2214
- [103]. Takada M., Nadeau K.C., Shaw G.D., and Tilney N.L.: Prevention of late renal changes after initial ischemia/reperfusion injury by blocking early selectin binding. Transplant 1997; 64: pp. 1520-1525
- [104]. Rabb H., Mendiola C.C., Dietz J., Saba S.R., Issekutz T.B., Abanilla F., et al: Role of CD11a and CD11b in ischemic acute renal failure in rats. Am J Physiol 1994; 267: pp. F1052-F1058
- [105]. Burne M.J., and Rabb H.: Pathophysiological contributions of fucosyltransferases in renal ischemia reperfusion injury. J Immunol 2002; 169: pp. 2648-2652
- [106]. Gasser M., Waaga A.M., Kist-van Holthe J.E., Lenhard S.M., Laskowski I., Shaw G.D., et al: Normalization of brain death-induced injury to rat renal allografts by recombinant soluble P-selectin glycoprotein ligand. J Am Soc Nephrol 2002; 13: pp. 1937-1945
- [107]. Dragun D., Tullius S.G., Park J.K., Maasch C., Lukitsch I., Lippoldt A., et al: ICAM-1 antisense oligodesoxynucleotides prevent reperfusion injury and enhance immediate graft function in renal transplantation. Kidney Int 1998; 54: pp. 590-602
- [108]. Safirstein R., Megyesi J., Saggi S.J., Price P.M., Poon M., Rollins B.J., et al: Expression of cytokine-like genes JE and KC is increased during renal ischemia. Am J Physiol 1991; 261: pp. F1095-F1100
- [109]. Furuichi K., Wada T., Iwata Y., Kitagawa K., Kobayashi K., Hashimoto H., et al: CCR2 signaling contributes to ischemia-reperfusion injury in kidney. J Am Soc Nephrol 2003; 14: pp. 2503-2515
- [110]. Furuichi K., Wada T., Iwata Y., Kitagawa K., Kobayashi K., Hashimoto H., et al: Gene therapy expressing amino-terminal truncated monocyte chemoattractant protein-1 prevents renal ischemia-reperfusion injury. J Am SocNephrol 2003; 14: pp. 1066-1071
- [111]. De Greef K.E., Ysebaert D.K., Vercauteren S.R., Persy V.P., and De Broe M.E.: Upregulation of B7-1 and B7-2 along the vasa recta after renal ischemia/reperfusion injury. Am J Transplantation 2001; 1: pp. A484
- [112]. Takada M., Chandraker A., Ndeau K.C., Sayegh M., and Tilney N.L.: The role of the B7 costimulatory pathway in experimental cold ischemia/ reperfusion injury. J Clin Invest 1997; 100: pp. 1199-1203
- [113]. Zhang Y., Woodward V.K., Shelton J.M., Richardson J.A., Link D.C., Zhou X.J., et al: Ischemia/ reperfusion induces G-CSF gene expression by renal medullary thick ascending limb cells in vivo and in vitro. Am J Physiol Renal Physiol 2004; 286: pp. F1193-F1201
- [114]. Lauriat S., and Linas S.L.: The role of neutrophils in acute renal failure. Semin Nephrol 1998; 18: pp. 498-504
- [115]. De Greef K.E., Ysebaert D.K., Ghielli M., Vercauteren S., Nouwen E.J., Eyskens E.J., et al: Neutrophils and acute ischemia-reperfusion injury. J Nephrol 1998; 11: pp. 110-122
- [116]. Miura M., Fu X., Zhang Q., Remick D.G., and Fairchild R.L.: Neutralization of kc and macrophage inflammatory protein-2 attenuates renal ischemia/reperfusion injury. Am J Transplantation 2001; 1: pp. A1292
- [117]. Melnikov V.Y., Faubel S., Siegmund B., Lucia M.S., Ljubanovic D., and Edelstein C.L.: Neutrophil-independent mechanisms of caspase-1- and IL-18-mediated ischemic acute tubular necrosis in mice. J Clin Invest 2002; 110: pp. 1083-1091
- [118]. de Vries B., Kohl J., Leclercq W.K., Wolfs T.G., van Bijnen A.A., Heeringa P., et al: Complement factor C5a mediates renal ischemia-reperfusion injury independent from neutrophils. J Immunol 2003; 170: pp. 3883-3889
- [119]. Taylor P.R., and Gordon S.: Monocyte heterogeneity and innate immunity. Immunity 2003; 19: pp. 2-4
- [120]. Araki M., Fairchild R.L., et al: [1461] Role of cxcr2 in renal ischemia/reperfusion injury. Am J Transpl 2004; undefined: pp. 41461
- [121]. Grone H.J., Cohen C.D., Grone E., Schmidt C., Kretzler M., Schlondorff D., et al: Spatial and temporally restricted expression of chemokines and chemokine receptors in the developing human kidney. J Am Soc Nephrol 2002; 13: pp. 957-967
- [122]. Luan J., Furuta Y., Du J., and Richmond A.: Developmental expression of two CXC chemokines, MIP-2 and KC, and their receptors. Cytokine 2001; 14: pp. 253-263
- [123]. Scapini P., Lapinet-Vera J.A., Gasperini S., Calzetti F., Bazzoni F., and Cassatella M.A.: The neutrophil as a cellular source of chemokines. Immunol Rev 2000; 177: pp. 195-203
- [124]. El Sawy T., Fahmy N.M., and Fairchild R.L.: Chemokines: directing leukocyte infiltration into allografts. Curr Opin Immunol 2002; 14: pp. 562-568
- [125]. Mehrad B., Wiekowski M., Morrison B.E., Chen S.C., Coronel E.C., Manfra D.J., et al: Transient lung-specific expression of the chemokine KC improves outcome in invasive aspergillosis. Am J RespirCrit Care Med 2002; 166: pp. 1263-1268
- [126]. Li L., Huang L., Vergis A.L., Ye H., Bajwa A., Narayan V., et al: IL-17 produced by neutrophils regulates IFN-gamma-mediated neutrophil migration in mouse kidney ischemia-reperfusion injury. J Clin Invest 2010; 120: pp. 331-342
- [127]. Li L., Huang L., Sung S.S., Lobo P.I., Brown M.G., Gregg R.K., et al: NKT cell activation mediates neutrophil IFN-{gamma} production and renal ischemia-reperfusion injury. J Immunol 2007; 178: pp. 5899-5911
- [128]. Faubel S.G., Ljubanovic D., Poole B., Dursun B., Cushing S., He Z., et al: Peripheral CD4 T cell depletion is not sufficient to prevent ischemic acute renal failure. Transplantation 2005; 80: pp. 643-649
- [129]. Dragun D., Bohler T., Nieminen-Kelha M., Waiser J., Schneider W., Haller H., et al: FTY720-induced lymphocyte homing modulates post-transplant preservation/reperfusion injury. Kidney Int 2004; 65: pp. 1076-1083
- [130]. Park P., Haas M., Cunningham P.N., Bao L., Alexander J.J., and Quigg R.J.: Injury in renal ischemia-reperfusion is independent from immunoglobulins and T lymphocytes. Am J Physiol Renal Physiol 2002; 282: pp. F352-F357
- [131]. Yokota N., Daniels F., Crosson J., and Rabb H.: Protective effect of T cell depletion in murine renal ischemia-reperfusion injury. Transplant 2002; 74: pp. 759-763
- [132]. Burne-Taney M.J., Ascon D.B., Daniels F., Racusen L., Baldwin W., and Rabb H.: B cell deficiency confers protection from renal ischemia reperfusion injury. J Immunol 2003; 171: pp. 3210-3215
- [133]. Rouschop K.M., Sewnath M.E., Claessen N., Roelofs J.J., Hoedemaeker I., van der N.R., et al: CD44 deficiency increases tubular damage but reduces renal fibrosis in obstructive nephropathy. J Am Soc Nephrol 2004; 15: pp. 674-686
- [134]. Florquin S., and Rouschop K.M.: Reciprocal functions of hepatocyte growth factor and transforming growth factor-beta1 in the progression of renal diseases: a role for CD44? Kidney Int Suppl 2003; undefined: pp. S15-S20
- [135]. Kipnis J., Mizrahi T., Hauben E., Shaked I., Shevach E., and Schwartz M.: Neuroprotective autoimmunity: naturally occurring CD4+CD25+ regulatory T cells suppress the ability to withstand injury to the central nervous system. Proc Natl Acad Sci USA 2002; 99: pp. 15620-15625
- [136]. Caldwell C.C., Okaya T., Martignoni A., Husted T., Schuster R., and Lentsch A.B.: Divergent functions of CD4+ T lymphocytes in acute liver inflammation and injury after ischemia-reperfusion. Am J Physiol Gastrointest Liver Physiol 2005; 289: pp. G969-G976
- [137]. Yokota N., Burne-Taney M., Racusen L., and Rabb H.: Contrasting roles for STAT4 and STAT6 signal transduction pathways in murine renal ischemia-reperfusion injury. Am J Physiol Renal Physiol 2003; 285: pp. F319-F325
- [138]. Cario E., Becker A., Sturm A., Goebell H., and Dignass A.U.: Peripheral blood mononuclear cells promote intestinal epithelial restitution in vitro through an interleukin-2/interferon-gamma-dependent pathway. Scand J Gastroenterol 1999; 34: pp. 1132-1138
- [139]. Ahdieh M., VandenBos T., and Youakim A.: Lung epithelial barrier function and wound healing are decreased by IL-4 and IL-13 and enhanced by IFN-gamma. AJP – Cell Physiol 2001; 281: pp. C2029-C2038
- [140]. Jameson J., Ugarte K., Chen N., Yachi P., Fuchs E., Boismenu R., et al: Role for Skin gamma delta T Cells in Wound Repair. Science 2002; 296: pp. 747-749
- [141]. Lai L.W., Yong K.C., and Lien Y.H.: Pharmacologic recruitment of regulatory T cells as a therapy for ischemic acute kidney injury. Kidney Int 2011; undefined:
- [142]. Kinsey G.R., Huang L., Vergis A.L., Li L., and Okusa M.D.: Regulatory T cells contribute to the protective effect of ischemic preconditioning in the kidney. Kidney Int 2010; undefined:
- [143]. Linfert D., Chowdhry T., and Rabb H.: Lymphocytes and ischemia-reperfusion injury. Transplant Rev (Orlando) 2009; 23: pp. 1-10
- [144]. Matzinger P.: Friendly and dangerous signals: is the tissue in control? Nat Immunol 2007; 8: pp. 11-13
- [145]. Oppenheim J.J., Tewary P., de la Rosa G., and Yang D.: Alarmins initiate host defense. Adv Exp Med Biol 2007; 601: pp. 185-194
- [146]. Rock K.L., Latz E., Ontiveros F., and Kono H.: The sterile inflammatory response. Annu Rev Immunol 2010; 28: pp. 321-342
- [147]. Mollen K.P., Anand R.J., Tsung A., Prince J.M., Levy R.M., and Billiar T.R.: Emerging paradigm: toll-like receptor 4-sentinel for the detection of tissue damage. Shock 2006; 26: pp. 430-437
- [148]. Takeda K., Kaisho T., and Akira S.: Toll-like receptors. Annu Rev Immunol 2003; 21: pp. 335-376
- [149]. Shigeoka A.A., Holscher T.D., King A.J., Hall F.W., Kiosses W.B., Tobias P.S., et al: TLR2 is constitutively expressed within the kidney and participates in ischemic renal injury through both MyD88-dependent and -independent pathways. J Immunol 2007; 178: pp. 6252-6258
- [150]. Chen J., John R., Richardson J.A., Shelton J.M., Zhou X.J., Wang Y., et al: Toll-like receptor 4 regulates early endothelial activation during ischemic acute kidney injury. Kidney Int 2011; 79: pp. 288-299
- [151]. Beutler B., Hoebe K., Du X., and Ulevitch R.J.: How we detect microbes and respond to them: the Toll-like receptors and their transducers. J Leukoc Biol 2003; 74: pp. 479-485
- [152]. Akira S., and Takeda K.: Toll-like receptor signalling. Nat Rev Immunol 2004; 4: pp. 499-511
- [153]. Iwasaki A., and Medzhitov R.: Toll-like receptor control of the adaptive immune responses. Nat Immunol 2004; 5: pp. 987-995
- [154]. Matsuguchi T., Takagi K., Musikacharoen T., and Yoshikai Y.: Gene expressions of lipopolysaccharide receptors, toll-like receptors 2 and 4, are differently regulated in mouse T lymphocytes. Blood 2000; 95: pp. 1378-1385
- [155]. Liew F.Y., Komai-Koma M., and Xu D.: A toll for T cell costimulation. Ann Rheum Dis 2004; 63: pp. ii76-ii78
- [156]. Asea A., Kraeft S.K., Kurt-Jones E.A., Stevenson M.A., Chen L.B., Finberg R.W., et al: HSP70 stimulates cytokine production through a CD14-dependant pathway, demonstrating its dual role as a chaperone and cytokine. Nat Med 2000; 6: pp. 435-442
- [157]. Asea A., Rehli M., Kabingu E., Boch J.A., Bare O., Auron P.E., et al: Novel signal transduction pathway utilized by extracellular HSP70: role of toll-like receptor (TLR) 2 and TLR4. J Biol Chem 2002; 277: pp. 15028-15034
- [158]. Beg A.A.: Endogenous ligands of Toll-like receptors: implications for regulating inflammatory and immune responses. Trends Immunol 2002; 23: pp. 509-512
- [159]. Vabulas R.M., Ahmad-Nejad P., Ghose S., Kirschning C.J., Issels R.D., and Wagner H.: HSP70 as endogenous stimulus of the Toll/interleukin-1 receptor signal pathway. J Biol Chem 2002; 277: pp. 15107-15112
- [160]. Vabulas R.M., Braedel S., Hilf N., Singh-Jasuja H., Herter S., Ahmad-Nejad P., et al: The endoplasmic reticulum-resident heat shock protein Gp96 activates dendritic cells via the Toll-like receptor 2/4 pathway. J Biol Chem 2002; 277: pp. 20847-20853
- [161]. Vabulas R.M., Wagner H., and Schild H.: Heat shock proteins as ligands of toll-like receptors. Curr Top Microbiol Immunol 2002; 270: pp. 169-184
- [162]. Johnson G.B., Brunn G.J., Kodaira Y., and Platt J.L.: Receptor-mediated monitoring of tissue well-being via detection of soluble heparan sulfate by Toll-like receptor 4. J Immunol 2002; 168: pp. 5233-5239
- [163]. Johnson G.B., Brunn G.J., and Platt J.L.: Cutting edge: an endogenous pathway to systemic inflammatory response syndrome (SIRS)-Like reactions through toll-like receptor 4. J Immunol 2004; 172: pp. 20-24
- [164]. Oyama J., Blais C., Liu X., Pu M., Kobzik L., Kelly R.A., et al: Reduced myocardial ischemia-reperfusion injury in toll-like receptor 4-deficient mice. Circulation 2004; 109: pp. 784-789
- [165]. Chong A.J., Shimamoto A., Hampton C.R., Takayama H., Spring D.J., Rothnie C.L., et al: Toll-like receptor 4 mediates ischemia/reperfusion injury of the heart. J Thorac Cardiovasc Surg 2004; 128: pp. 170-179
- [166]. Peng Y., Gong J.P., Liu C.A., Li X.H., Gan L., and Li S.B.: Expression of toll-like receptor 4 and MD-2 gene and protein in Kupffer cells after ischemia-reperfusion in rat liver graft. World J Gastroenterol 2004; 10: pp. 2890-2893
- [167]. Wu H.S., Zhang J.X., Wang L., Tian Y., Wang H., and Rotstein O.: Toll-like receptor 4 involvement in hepatic ischemia/reperfusion injury in mice. Hepatobiliary Pancreat Dis Int 2004; 3: pp. 250-253
- [168]. Zhai Y., Shen X.D., O’Connell R., Gao F., Lassman C., Busuttil R.W., et al: Cutting Edge: TLR4 activation mediates liver ischemia/reperfusion inflammatory response via IFN regulatory factor 3-dependent MyD88-independent pathway. J Immunol 2004; 173: pp. 7115-7119
- [169]. Barsness K.A., Arcaroli J., Harken A.H., Abraham E., Banerjee A., Reznikov L., et al: Hemorrhage-induced acute lung injury is TLR-4 dependent. Am J Physiol RegulIntegr Comp Physiol 2004; 287: pp. R592-R599
- [170]. Wu H., Chen G., Wyburn K.R., Yin J., Bertolino P., Eris J.M., et al: TLR4 activation mediates kidney ischemia/reperfusion injury. J Clin Invest 2007; 117: pp. 2847-2859
- [171]. Pulskens W.P., Teske G.J., Butter L.M., Roelofs J.J., van der Poll T., Florquin S., et al: Toll-like receptor-4 coordinates the innate immune response of the kidney to renal ischemia/reperfusion injury. PLoS ONE 2008; 3: pp. e3596
- [172]. Rusai K., Sollinger D., Baumann M., Wagner B., Strobl M., Schmaderer C., et al: Toll-like receptors 2 and 4 in renal ischemia/reperfusion injury. Pediatr Nephrol 2010; 25: pp. 853-860
- [173]. Kruger B., Krick S., Dhillon N., Lerner S.M., Ames S., Bromberg J.S., et al: Donor Toll-like receptor 4 contributes to ischemia and reperfusion injury following human kidney transplantation. Proc Natl Acad Sci U S A 2009; 106: pp. 3390-3395
- [174]. Chen J., Hartono J., John R., Bennett M., Zhou X., Wang Y., et al: Interleukin 6 production by leukocytes during ischemic acute kidney injury is regulated by TLR4. Kidney Int 2011; 80: pp. 504-515
- [175]. John R., Chen L., Bennett M., Richardson J.A., Zhou X.J., Shelton J.M., et al: Potential roles for collecting duct and endothelial TLR4 in murine ischemic acute renal injury. Under Review 2006; undefined:
- [176]. Yang H., Hreggvidsdottir H.S., Palmblad K., Wang H., Ochani M., Li J., et al: A critical cysteine is required for HMGB1 binding to Toll-like receptor 4 and activation of macrophage cytokine release. Proc Natl Acad Sci U S A 2010; 107: pp. 11942-11947
- [177]. Li J., Gong Q., Zhong S., Wang L., Guo H., Xiang Y., et al: Neutralization of the extracellular HMGB1 released by ischaemic damaged renal cells protects against renal ischaemia-reperfusion injury. Nephrol Dial Transplant 2010; 26: pp. 469-478
- [178]. Wu H., Ma J., Wang P., Corpuz T.M., Panchapakesan U., Wyburn K.R., et al: HMGB1 Contributes to kidney ischemia reperfusion injury. J Am Soc Nephrol 2010; 21: pp. 1878-1890
- [179]. Li C., and Jackson R.M.: Reactive species mechanisms of cellular hypoxia-reoxygenation injury. Am J Physiol Cell Physiol 2002; 282: pp. C227-C241
- [180]. Chandel N.S., Maltepe E., Goldwasser E., Mathieu C.E., Simon M.C., and Schumacker P.T.: Mitochondrial reactive oxygen species trigger hypoxia-induced transcription. Proc Natl Acad Sci USA 1998; 95: pp. 11715-11720
- [181]. Nath K.A., and Norby S.M.: Reactive oxygen species and acute renal failure. Am J Med 2000; 109: pp. 665-678
- [182]. Kelly K.J., Williams W.W., Colvin R.B., Meehan S.M., Springer T.A., Gutierrez-ramos J.C., et al: Intercellular adhesion molecule-1-deficient mice are protected against ischemic renal injury. J Clin Invest 1996; 97: pp. 1056-1063
- [183]. Kiew L.V., Munavvar A.S., Law C.H., Azizan A.N., Nazarina A.R., Sidik K., et al: Effect of antisense oligodeoxynucleotides for ICAM-1 on renal ischaemia-reperfusion injury in the anaesthetised rat. J Physiol 2004; 557: pp. 981-989
- [184]. Haller H., Dragun D., Miethke A., Park J.K., Weis A., Lippoldt A., et al: Antisense oligonucleotides for ICAM 1 attenuate reperfusion injury and renal failure in the rat. Kidney Int 1996; 50: pp. 473-480
- [185]. Lu C.Y., Winterberg P.D., Chen J., and Hartono J.R.: Acute kidney injury: a conspiracy of toll-like receptor 4 on endothelia, leukocytes, and tubules. Pediatr Nephrol 2011; undefined:
- [186]. Wang H., Bloom O., Zhang M., Vishnubhakat J.M., Ombrellino M., Che J., et al: HMG-1 as a late mediator of endotoxin lethality in mice. Science 1999; 285: pp. 248-251
- [187]. Andersson U., and Tracey K.J.: HMGB1 is a therapeutic target for sterile inflammation and infection. Annu Rev Immunol 2011; 29:
- [188]. Bianchi M.E., and Celona B.: Ancient news: HMGBs are universal sentinels. J Mol Cell Biol 2010; 2: pp. 116-117
- [189]. Tang D., Kang R., Zeh H.J., and Lotze M.T.: High-mobility group box 1, oxidative stress, and disease. Antioxid Redox Signal 2011; 14: pp. 1315-1335
- [190]. Dumitriu I.E., Baruah P., Manfredi A.A., Bianchi M.E., and Rovere-Querini P.: HMGB1: guiding immunity from within. Trends Immunol 2005; 26: pp. 381-387
- [191]. Lotze M.T., and Tracey K.J.: High-mobility group box 1 protein (HMGB1): nuclear weapon in the immune arsenal. Nat Rev Immunol 2005; 5: pp. 331-342
- [192]. Dessing M.C., Pulskens W.P., Teske G.J., Butter L.M., van der Poll T., Yang H., et al: RAGE does not contribute to renal injury and damage upon ischemia/reperfusion-induced injury. J Innate Immun 2011; undefined:
- [193]. Miller Y.I., Choi S.H., Wiesner P., Fang L., Harkewicz R., Hartvigsen K., et al: Oxidation-specific epitopes are danger-associated molecular patterns recognized by pattern recognition receptors of innate immunity. Circ Res 2011; 108: pp. 235-248
- [194]. Gill R., Tsung A., and Billiar T.: Linking oxidative stress to inflammation: toll-like receptors. Free Radic Biol Med 2010; 48: pp. 1121-1132
- [195]. Taylor K.R., Trowbridge J.M., Rudisill J.A., Termeer C.C., Simon J.C., and Gallo R.L.: Hyaluronan fragments stimulate endothelial recognition of injury through TLR4. J BiolChem 2004; 279: pp. 17079-17084
- [196]. Jiang D., Liang J., Fan J., Yu S., Chen S., Luo Y., et al: Regulation of lung injury and repair by Toll-like receptors and hyaluronan. Nat Med 2005; 11: pp. 1173-1179
- [197]. Kawabata K., Hagio T., and Matsuoka S.: The role of neutrophil elastase in acute lung injury. EurJ Pharmacol 2002; 451: pp. 1-10
- [198]. Linas S.L., Whittenburg D., Parsons P.E., and Repine J.E.: Mild renal ischemia activates primed neutrophils to cause acute renal failure. Kidney Int 1992; 42: pp. 610-616
- [199]. Johnsson C., Tufveson G., Wahlberg J., and Hallgren R.: Experimentally-induced warm renal ischemia induces cortical accumulation of hyaluronan in the kidney. Kidney Int 1996; 50: pp. 1224-1229
- [200]. Wuthrich R.P.: The proinflammatory role of hyaluronan-CD44 interactions in renal injury. Nephrol Dial Transplant 1999; 14: pp. 2554-2556
- [201]. Schaefer L., Babelova A., Kiss E., Hausser H.J., Baliova M., Krzyzankova M., et al: The matrix component biglycan is proinflammatory and signals through Toll-like receptors 4 and 2 in macrophages. J Clin Invest 2005; 115: pp. 2223-2233
- [202]. Gunther E., and Walter L.: Genetic aspects of the hsp70 multigene family in vertebrates. Experientia 1994; 50: pp. 987-1001
- [203]. Hunt C.R., Gasser D.L., Chaplin D.D., Pierce J.C., and Kozak C.A.: Chromosomal localization of five murine HSP70 gene family members: Hsp70-1, Hsp70-2, Hsp70-3, Hsc70t, and Grp78. Genomics 1993; 16: pp. 193-198
- [204]. Cornell L.D., and Colvin R.B.: Chronic allograft nephropathy. Curr Opin Nephrol Hypertens 2005; 14: pp. 229-234
- [205]. Peelman L.J., Van de Weghe A.R., Coppieters W.R., Van Zeveren A.J., and Bouquet Y.H.: Complete nucleotide sequence of a porcine HSP70 gene. Immunogenetics 1992; 35: pp. 286-289
- [206]. Walter L., Rauh F., and Gunther E.: Comparative analysis of the three major histocompatibility complex-linked heat shock protein 70 (Hsp70) genes of the rat. Immunogenetics 1994; 40: pp. 325-330
- [207]. Robert J., Menoret A., Basu S., Cohen N., and Srivastava P.R.: Phylogenetic conservation of the molecular and immunological properties of the chaperones gp96 and hsp70. Eur J Immunol 2001; 31: pp. 186-195
- [208]. Maki R.G., Old L.J., and Srivastava P.K.: Human homologue of murine tumor rejection antigen gp96: 5′-regulatory and coding regions and relationship to stress-induced proteins. Proc Natl Acad Sci USA 1990; 87: pp. 5658-5662
- [209]. Zitvogel L., Casares N., Pequignot M.O., Chaput N., Albert M.L., and Kroemer G.: Immune response against dying tumor cells. Adv Immunol 2004; 84: pp. 131-179
- [210]. Van Why S.K., Hildebrandt F., Ardito T., Mann A.S., Siegel N.J., and Kashgarian M.: Induction and intracellular localization of HSP-72 after renal ischemia. Am J Physiol 1992; 263: pp. F769-F775
- [211]. Emami A., Schwartz J.H., and Borkan S.C.: Transient ischemia or heat stress induces a cytoprotectant protein in rat kidney. Am J Physiol 1991; 260: pp. F479-F485
- [212]. Schober A., Muller E., Thurau K., and Beck F.X.: The response of heat shock proteins 25 and 72 to ischaemia in different kidney zones. Pflugers Arch 1997; 434: pp. 292-299
- [213]. Morita K., Wakui H., Komatsuda A., Ohtani H., Miura A.B., Itoh H., et al: Induction of heat-shock proteins HSP73 and HSP90 in rat kidneys after ischemia. Ren Fail 1995; 17: pp. 405-419
- [214]. Mao H., Wang Y., Li Z., Ruchalski K.L., Yu X., Schwartz J.H., et al: HSP72 interacts with paxillin and facilitates the reassembly of focal adhesions during recovery from ATP depletion. J Biol Chem 2004; 279: pp. 15472-15480
- [215]. Van Why S.K., and Siegel N.J.: Heat shock proteins in renal injury and recovery. Curr Opin Nephrol Hypertens 1998; 7: pp. 407-412
- [216]. Beck F.X., Neuhofer W., and Muller E.: Molecular chaperones in the kidney: distribution, putative roles, and regulation. AJP – Renal Physiol 2000; 279: pp. F203-F215
- [217]. Kelly K.J., Baird N.R., and Greene A.L.: Induction of stress response proteins and experimental renal ischemia/reperfusion. Kidney Int 2001; 59: pp. 1798-1802
- [218]. Bonventre J.V.: Kidney ischemic preconditioning. Curr Opin Nephrol Hypertens 2002; 11: pp. 43-48
- [219]. Asea A.: Chaperokine-induced signal transduction pathways. ExercImmunol Rev 2003; 9: pp. 25-33
- [220]. Wallin R.P., Lundqvist A., More S.H., von Bonin A., Kiessling R., and Ljunggren H.G.: Heat-shock proteins as activators of the innate immune system. Trends Immunol 2002; 23: pp. 130-135
- [221]. Land W.: Allograft injury mediated by reactive oxygen species: from conserved proteins of Drosophila to acute and chronic rejection of human transplants. Part III: Interaction of (oxidative) stress-induced heat shock proteins with Toll-like receptor-bearing cells of innate immunity and its consequences forthe development of acute and chronic allograft rejection. Transplant Rev 2003; 17: pp. 67-86
- [222]. Skokos D., Botros H.G., Demeure C., Morin J., Peronet R., Birkenmeier G., et al: Mast cell-derived exosomes induce phenotypic and functional maturation of dendritic cells and elicit specific immune responses in vivo. J Immunol 2003; 170: pp. 3037-3045
- [223]. Wubbolts R., Leckie R.S., Veenhuizen P.T., Schwarzmann G., Mobius W., Hoernschemeyer J., et al: Proteomic and biochemical analyses of human B cell-derived exosomes. Potential implications for their function and multivesicular body formation. J Biol Chem 2003; 278: pp. 10963-10972
- [224]. Morelli A.E., Larregina A.T., Shufesky W.J., Sullivan M.L., Stolz D.B., Papworth G.D., et al: Intracellular Sorting and Processing of Exosomes by Dendritic Cells. Blood 2004; 104: pp. 3257-3266
- [225]. Wand-Wurttenberger A., Schoel B., Ivanyi J., and Kaufmann S.H.: Surface expression by mononuclear phagocytes of an epitope shared with mycobacterial heat shock protein 60. Eur J Immunol 1991; 21: pp. 1089-1092
- [226]. Soltys B.J., and Gupta R.S.: Cell surface localization of the 60 . Cell Biol Int 1997; 21: pp. 315-320
- [227]. Soltys B.J., and Gupta R.S.: Immunoelectron microscopic localization of the 60-kDa heat shock chaperonin protein (Hsp60) in mammalian cells. Exp Cell Res 1996; 222: pp. 16-27
- [228]. Gross C., Koelch W., DeMaio A., Arispe N., and Multhoff G.: Cell surface-bound heat shock protein 70 (Hsp70) mediates perforin-independent apoptosis by specific binding and uptake of granzyme B. J Biol Chem 2003; 278: pp. 41173-41181
- [229]. Okamura Y., Watari M., Jerud E.S., Young D.W., Ishizaka S.T., Rose J., et al: The extra domain A of fibronectin activates Toll-like receptor 4. J Biol Chem 2001; 276: pp. 10229-10233
- [230]. Zuk A., Bonventre J.V., and Matlin K.S.: Expression of fibronectin splice variants in the postischemic rat kidney. AJP – Ren Physiol 2001; 280: pp. F1037-F1053
- [231]. Biragyn A., Ruffini P.A., Leifer C.A., Klyushnenkova E., Shakhov A., Chertov O., et al: Toll-like receptor 4-dependent activation of dendritic cells by beta-defensin 2. Science 2002; 298: pp. 1025-1029
- [232]. Saemann M.D., Weichhart T., Zeyda M., Staffler G., Schunn M., Stuhlmeier K.M., et al: Tamm-Horsfall glycoprotein links innate immune cell activation with adaptive immunity via a Toll-like receptor-4-dependent mechanism. J Clin Invest 2005; 115: pp. 468-475
- [233]. El-Achkar T.M., McCracken R., Rauchman M., Heitmeier M.R., Al-Aly Z., Dagher P.C., et al: Tamm-Horsfall protein-deficient thick ascending limbs promote injury to neighboring S3 segments in an MIP-2-dependent mechanism. Am J Physiol Renal Physiol 2011; 300: pp. F999-1007
- [234]. Rosin D.L., and Okusa M.D.: Dangers within: DAMP responses to damage and cell death in kidney disease. J Am Soc Nephrol 2011; 22: pp. 416-425
- [235]. Nishitani C., Mitsuzawa H., Hyakushima N., Sano H., Matsushima N., and Kuroki Y.: The Toll-like receptor 4 region Glu24-Pro34 is critical for interaction with MD-2. Biochem Biophys Res Commun 2005; 328: pp. 586-590
- [236]. Triantafilou M., and Triantafilou K.: Lipopolysaccharide recognition: CD14, TLRs and the LPS-activation cluster. Trends Immunol 2002; 23: pp. 301-304
- [237]. Triantafilou M., Morath S., Mackie A., Hartung T., and Triantafilou K.: Lateral diffusion of Toll-like receptors reveals that they are transiently confined within lipid rafts on the plasma membrane. J Cell Sci 2004; 117: pp. 4007-4014
- [238]. Underhill D.M.: Toll-like receptors: networking for success. Eur J Immunol 2003; 33: pp. 1767-1775
- [239]. Muller M., Scheel O., Lindner B., Gutsmann T., and Seydel U.: The role of membrane-bound LBP, endotoxin aggregates, and the MaxiK channel in LPS-induced cell activation. J Endotoxin Res 2003; 9: pp. 181-186
- [240]. Becker T., Hartl F.U., and Wieland F.: CD40, an extracellular receptor for binding and uptake of Hsp70-peptide complexes. J Cell Biol 2002; 158: pp. 1277-1285
- [241]. Stebbing J., Savage P., Patterson S., and Gazzard B.: All for CD91 and CD91 for all. J Antimicrob Chemother 2004; 53: pp. 1-3
- [242]. Termeer C., Benedix F., Sleeman J., Fieber C., Voith U., Ahrens T., et al: Oligosaccharides of Hyaluronan activate dendritic cells via toll-like receptor 4. J Exp Med 2002; 195: pp. 99-111
- [243]. Millar D.G., Garza K.M., Odermatt B., Elford A.R., Ono N., Li Z., et al: Hsp70 promotes antigen-presenting cell function and converts T-cell tolerance to autoimmunity in vivo. Nat Med 2003; 9: pp. 1469-1476
- [244]. Knotek M., Rogachev B., Wang W., Ecder T., Melnikov V., Gengaro P.E., et al: Endotoxemic renal failure in mice: Role of tumor necrosis factor independent of inducible nitric oxide synthase. Kidney Int 2001; 59: pp. 2243-2249
- [245]. Bonventre J.V., and Zuk A.: Ischemic acute renal failure: an inflammatory disease? Kidney Int 2004; 66: pp. 480-485
- [246]. Noiri E., Peresleni T., Miller F., and Goligorsky M.S.: In vivo targeting of inducible NO synthase with oligodeoxynucleotides protects rat kidney against ischemia. J Clin Invest 1996; 97: pp. 2377-2383
- [247]. Ling H., Edelstein C., Gengaro P., Meng X., Lucia S., Knotek M., et al: Attenuation of renal ischemia-reperfusion injury in inducible nitric oxide synthase knockout mice. Am J Physiol Renal Physiol 1999; 277: pp. F383-F390
- [248]. Donnahoo K.K., Meng X., Ao L., Ayala A., Shames B.D., Cain M.P., et al: Differential cellular immunolocalization of renal tumour necrosis factor-alpha production during ischaemia versus endotoxaemia. Immunology 2001; 102: pp. 53-58
- [249]. Cunningham P.N., Wang Y., Guo R., He G., and Quigg R.J.: Role of toll-like receptor 4 in endotoxin-induced acute renal failure. J Immunol 2004; 172: pp. 2629-2635
- [250]. Mittal D., Saccheri F., Venereau E., Pusterla T., Bianchi M.E., and Rescigno M.: TLR4-mediated skin carcinogenesis is dependent on immune and radioresistant cells. EMBO J 2010; undefined:
- [251]. Midwood K., Sacre S., Piccinini A.M., Inglis J., Trebaul A., Chan E., et al: Tenascin-C is an endogenous activator of Toll-like receptor 4 that is essential for maintaining inflammation in arthritic joint disease. Nat Med 2009; 15: pp. 774-780
- [252]. Spear A.M., Loman N.J., Atkins H.S., and Pallen M.J.: Microbial TIR domains: not necessarily agents of subversion? Trends Microbiol 2009; 17: pp. 393-398
- [253]. Gauthier M.E., Du Pasquier L., and Degnan B.M.: The genome of the sponge Amphimedon queenslandica provides new perspectives into the origin of Toll-like and interleukin 1 receptor pathways. Evol Dev 2010; 12: pp. 519-533
- [254]. Barton G.M.: A calculated response: control of inflammation by the innate immune system. J Clin Invest 2008; 118: pp. 413-420
- [255]. Chen G.Y., Tang J., Zheng P., and Liu Y.: CD24 and Siglec-10 selectively repress tissue damage-induced immune responses. Science 2009; 323: pp. 1722-1725
- [256]. Liu Y., Chen G.Y., and Zheng P.: CD24-Siglec G/10 discriminates danger- from pathogen-associated molecular patterns. Trends Immunol 2009; 30: pp. 557-561
- [257]. Ungaro R., Fukata M., Hsu D., Hernandez Y., Breglio K., Chen A., et al: A novel Toll-like receptor 4 antagonist antibody ameliorates inflammation but impairs mucosal healing in murine colitis. Am J Physiol Gastrointest Liver Physiol 2009; 296: pp. G1167-G1179
- [258]. Rakoff-Nahoum S., Paglino J., Eslami-Varzaneh F., Edberg S., and Medzhitov R.: Recognition of commensal microflora by toll-like receptors is required for intestinal homeostasis. Cell 2004; 118: pp. 229-241
- [259]. Putnins E.E., Sanaie A.R., Wu Q., and Firth J.D.: Induction of keratinocyte growth factor 1 Expression by lipopolysaccharide is regulated by CD-14 and toll-like receptors 2 and 4. Infect Immun 2002; 70: pp. 6541-6548
- [260]. Sanale A.R., Firth J.D., Uitto V.J., and Putnins E.E.: Keratinocyte growth factor (KGF)-1 and -2 protein and gene expression in human gingival fibroblasts. J Periodontal Res 2002; 37: pp. 66-74
- [261]. Sugiyama A., Ogawa T., Daikuhara Y., and Takada H.: Enhancement of hepatocyte growth factor (scatter factor) production by human gingival fibroblasts in culture stimulated with Porphyromonas gingivalis fimbriae. J Med Microbiol 2000; 49: pp. 319-325
- [262]. Yoshioka T., Morimoto Y., Iwagaki H., Itoh H., Saito S., Kobayashi N., et al: Bacterial lipopolysaccharide induces transforming growth factor beta and hepatocyte growth factor through toll-like receptor 2 in cultured human colon cancer cells. J Int Med Res 2001; 29: pp. 409-420
- [263]. Spix J.K., Chay E.Y., Block E.R., and Klarlund J.K.: Hepatocyte growth factor induces epithelial cell motility through transactivation of the epidermal growth factor receptor. Exp Cell Res 2007; 313: pp. 3319-3325
- [264]. Hsu D., Fukata M., Hernandez Y.G., Sotolongo J.P., Goo T., Maki J., et al: Toll-like receptor 4 differentially regulates epidermal growth factor-related growth factors in response to intestinal mucosal injury. Lab Invest 2010; 90: pp. 1295-1305
- [265]. Zeng F., Singh A.B., and Harris R.C.: The role of the EGF family of ligands and receptors in renal development, physiology and pathophysiology. Exp Cell Res 2009; 315: pp. 602-610
- [266]. Zhang X., Shan P., Jiang G., Cohn L., and Lee P.J.: Toll-like receptor 4 deficiency causes pulmonary emphysema. J Clin Invest 2006; 116: pp. 3050-3059
- [267]. Qureshi S.T., Zhang X., Aberg E., Bousette N., Giaid A., Shan P., et al: Inducible activation of TLR4 confers resistance to hyperoxia-induced pulmonary apoptosis. J Immunol 2006; 176: pp. 4950-4958
- [268]. Zhang X., Shan P., Qureshi S., Homer R., Medzhitov R., Noble P.W., et al: Cutting edge: TLR4 deficiency confers susceptibility to lethal oxidant lung injury. J Immunol 2005; 175: pp. 4834-4838
- [269]. Paun A., Fox J., Balloy V., Chignard M., Qureshi S.T., and Haston C.K.: Combined Tlr2 and Tlr4 deficiency increases radiation-induced pulmonary fibrosis in mice. Int J Radiat Oncol Biol Phys 2010; 77: pp. 1198-1205
- [270]. Goren I., Allmann N., Yogev N., Schurmann C., Linke A., Holdener M., et al: A transgenic mouse model of inducible macrophage depletion: effects of diphtheria toxin-driven lysozyme M-specific cell lineage ablation on wound inflammatory, angiogenic, and contractive processes. Am J Pathol 2009; 175: pp. 132-147
- [271]. Campbell J.S., Riehle K.J., Brooling J.T., Bauer R.L., Mitchell C., and Fausto N.: Proinflammatory cytokine production in liver regeneration is Myd88-dependent, but independent of Cd14, Tlr2, and Tlr4. J Immunol 2006; 176: pp. 2522-2528
- [272]. Shigeoka A.A., Kambo A., Mathison J.C., King A.J., Hall W.F., da Silva Correia J., et al: Nod1 and nod2 are expressed in human and murine renal tubular epithelial cells and participate in renal ischemia reperfusion injury. J Immunol 2010; 184: pp. 2297-2304
- [273]. Shigeoka A.A., Mueller J.L., Kambo A., Mathison J.C., King A.J., Hall W.F., et al: An inflammasome-independent role for epithelial-expressed nlrp3 in renal ischemia-reperfusion injury. J Immunol 2010; undefined:
- [274]. Basu S., Binder R.J., Ramalingam T., and Srivastava P.K.: CD91 is a common receptor for heat shock proteins gp96, hsp90, hsp70, and calreticulin. Immunity 2001; 14: pp. 303-313
- [275]. Binder R.J., Han D.K., and Srivastava P.K.: CD91: a receptor for heat shock protein gp96. Nat Immunol 2000; 1: pp. 151-155
- [276]. Bhattacharjee G., Misra U.K., Gawdi G., Cianciolo G., and Pizzo S.V.: Inducible expression of the alpha2-macroglobulin signaling receptor in response to antigenic stimulation: a study of second messenger generation. J Cell Biochem 2001; 82: pp. 260-270
- [277]. Misra U.K., Gawdi G., Gonzalez-Gronow M., and Pizzo S.V.: Coordinate regulation of the alpha(2)-macroglobulin signaling receptor and the low density lipoprotein receptor-related protein/alpha(2)-macroglobulin receptor by insulin. J Biol Chem 1999; 274: pp. 25785-25791
- [278]. Gardai S.J., Xiao Y.Q., Dickinson M., Nick J.A., Voelker D.R., Greene K.E., et al: By binding SIRPalpha or calreticulin/CD91, lung collectins act as dual function surveillance molecules to suppress or enhance inflammation. Cell 2003; 115: pp. 13-23
- [279]. Herz J., and Hui D.Y.: Lipoprotein receptors in the vascular wall. Curr Opin Lipidol 2004; 15: pp. 175-181
- [280]. Kirschning C.J., and Schumann R.R.: TLR2: cellular sensor for microbial and endogenous molecular patterns. Curr Top Microbiol Immunol 2002; 270: pp. 121-144
- [281]. Sabroe I., Read R.C., Whyte M.K., Dockrell D.H., Vogel S.N., and Dower S.K.: Toll-like receptors in health and disease: complex questions remain. J Immunol 2003; 171: pp. 1630-1635
- [282]. Leemans J.C., Stokman G., Claessen N., Rouschop K.M., Teske G.J., Kirschning C.J., et al: Renal-associated TLR2 mediates ischemia/reperfusion injury in the kidney. J Clin Invest 2005; 115: pp. 2894-2903
- [283]. Kim B.S., Lim S.W., Li C., Kim J.S., Sun B.K., Ahn K.O., et al: Ischemia-reperfusion injury activates innate immunity in rat kidneys. Transplantation 2005; 79: pp. 1370-1377
- [284]. Wolfs T.G., Buurman W.A., van Schadewijk A., de Vries B., Daemen M.A., Hiemstra P.S., et al: In vivo expression of Toll-like receptor 2 and 4 by renal epithelial cells: IFN-gamma and TNF-alpha mediated up-regulation during inflammation. J Immunol 2002; 168: pp. 1286-1293
- [285]. Zhang J.X., Wu H.S., Wang H., Zhang J.H., Wang Y., and Zheng Q.C.: Protection against hepatic ischemia/reperfusion injury via downregulation of toll-like receptor 2 expression by inhibition of Kupffer cell function. World J Gastroenterol 2005; 11: pp. 4423-4426
- [286]. Shishido T., Nozaki N., Yamaguchi S., Shibata Y., Nitobe J., Miyamoto T., et al: Toll-like receptor-2 modulates ventricular remodeling after myocardial infarction. Circulation 2003; 108: pp. 2905-2910
- [287]. Wendt T., Tanji N., Guo J., Hudson B.I., Bierhaus A., Ramasamy R., et al: Glucose, glycation, and RAGE: implications for amplification of cellular dysfunction in diabetic nephropathy. J Am Soc Nephrol 2003; 14: pp. 1383-1395
- [288]. Nawroth P., Bierhaus A., Marrero M., Yamamoto H., and Stern D.M.: Atherosclerosis and restenosis: is there a role for RAGE? Curr Diab Rep 2005; 5: pp. 11-16
- [289]. Jensen L.J., Ostergaard J., and Flyvbjerg A.: AGE-RAGE and AGE Cross-link interaction: important players in the pathogenesis of diabetic kidney disease. Horm Metab Res 2005; 37: pp. 26-34
- [290]. Bohlender J.M., Franke S., Stein G., and Wolf G.: Advanced glycation end products and the kidney. AJP – Renal Physiol 2005; 289: pp. F645-F659
- [291]. Rabadi M.M., Kuo M.C., Ghaly T., Rabadi S.M., Weber M., Goligorsky M.S., et al: Interaction between uric acid and HMGB1 translocation and release from endothelial cells. Am J Physiol Renal Physiol 2011; undefined:
- [292]. Iyer S.S., Pulskens W.P., Sadler J.J., Butter L.M., Teske G.J., Ulland T.K., et al: Necrotic cells trigger a sterile inflammatory response through the Nlrp3 inflammasome. Proc Natl Acad Sci U S A 2009; 106: pp. 20388-20393
- [293]. Ricklin D., Hajishengallis G., Yang K., and Lambris J.D.: Complement: a key system for immune surveillance and homeostasis. Nat Immunol 2010; 11: pp. 785-797
- [294]. Thurman J.M., and Renner B.: Dynamic control of the complement system by modulated expression of regulatory proteins. Lab Invest 2011; 91: pp. 4-11
- [295]. Bao L., Wang Y., Chang A., Minto A.W., Zhou J., Kang H., et al: Unrestricted C3 activation occurs in Crry-deficient kidneys and rapidly leads to chronic renal failure. J Am Soc Nephrol 2007; 18: pp. 811-822
- [296]. Lockshin R.A., and Zakeri Z.: Caspase-independent cell death? Oncogene 2004; 23: pp. 2766-2773
- [297]. Edinger A.L., and Thompson C.B.: Death by design: apoptosis, necrosis and autophagy. Curr OpinCell Biol 2004; 16: pp. 663-669
- [298]. Vercammen D., Brouckaert G., Denecker G., Van de C.M., Declercq W., Fiers W., et al: Dual signaling of the Fas receptor: initiation of both apoptotic and necrotic cell death pathways. J Exp Med 1998; 188: pp. 919-930
- [299]. Leist M., and Jaattela M.: Four deaths and a funeral: from caspases to alternative mechanisms. Nat Rev Mol Cell Biol 2001; 2: pp. 589-598
- [300]. Proskuryakov S.Y., Konoplyannikov A.G., and Gabai V.L.: Necrosis: a specific form of programmed cell death? Exp Cell Res 2003; 283: pp. 1-16
- [301]. Kitanaka C., and Kuchino Y.: Caspase-independent programmed cell death with necrotic morphology. Cell Death Differ 1999; 6: pp. 508-515
- [302]. Devalaraja-Narashimha K., Singaravelu K., and Padanilam B.J.: Poly(ADP-ribose) polymerase-mediated cell injury in acute renal failure. Pharmacol Res 2005; 52: pp. 44-59
- [303]. Ame J.C., Spenlehauer C., and de Murcia G.: The PARP superfamily. Bioessays 2004; 26: pp. 882-893
- [304]. Boulares A.H., Zoltoski A.J., Sherif Z.A., Jolly P., Massaro D., and Smulson M.E.: Gene knockout or pharmacological inhibition of poly(ADP-ribose) polymerase-1 prevents lung inflammation in a murine model of asthma. Am J Respir Cell Mol Biol 2003; 28: pp. 322-329
- [305]. Martin D.R., Lewington A.J., Hammerman MR, and Padanilam B.J.: Inhibition of poly(ADP-ribose) polymerase attenuates ischemic renal injury in rats. Am J Physiol Regul Integr Comp Physiol 2000; 279: pp. R1834-R1840
- [306]. Chatterjee P.K., Chatterjee B.E., Pedersen H., Sivarajah A., McDonald M.C., Mota-Filipe H., et al: 5-Aminoisoquinolinone reduces renal injury and dysfunction caused by experimental ischemia/reperfusion. Kidney Int 2004; 65: pp. 499-509
- [307]. Patel N.S., Cortes U., Di Poala R., Mazzon E., Mota-Filipe H., Cuzzocrea S., et al: Mice lacking the 110-kD isoform of poly(ADP-Ribose) glycohydrolase are protected against renal ischemia/reperfusion injury. J Am Soc Nephrol 2005; 16: pp. 712-719
- [308]. Zheng J., Devalaraja-Narashimha K., Singaravelu K., and Padanilam B.J.: Poly(ADP-ribose) polymerase-1 gene ablation protects mice from ischemic renal injury. AJP – Renal Physiol 2005; 288: pp. F387-F398
- [309]. Chatterjee P.K., Zacharowski K., Cuzzocrea S., Otto M., and Thiemermann C.: Inhibitors of poly (ADP-ribose) synthetase reduce renal ischemia-reperfusion injury in the anesthetized rat in vivo. FASEB J 2000; 14: pp. 641-651
- [310]. Ha H.C., and Snyder S.H.: Poly(ADP-ribose) polymerase is a mediator of necrotic cell death by ATP depletion. Proc Natl Acad Sci USA 1999; 96: pp. 13978-13982
- [311]. Alano C.C., Ying W., and Swanson R.A.: Poly(ADP-ribose) polymerase-1-mediated cell death in astrocytes requires NAD+ depletion and mitochondrial permeability transition. J Biol Chem 2004; 279: pp. 18895-18902
- [312]. Szabo C., and Dawson V.L.: Role of poly(ADP-ribose) synthetase in inflammation and ischaemia-reperfusion. Trends Pharmacol Sci 1998; 19: pp. 287-298
- [313]. Los M., Mozoluk M., Ferrari D., Stepczynska A., Stroh C., Renz A., et al: Activation and caspase-mediated inhibition of PARP: a molecular switch between fibroblast necrosis and apoptosis in death receptor signaling. Mol Biol Cell 2002; 13: pp. 978-988
- [314]. Petrilli V., Herceg Z., Hassa P.O., Patel N.S., Di Paola R., Cortes U., et al: Noncleavable poly(ADP-ribose) polymerase-1 regulates the inflammation response in mice. J Clin Invest 2004; 114: pp. 1072-1081
- [315]. Baines C.P., Kaiser R.A., Purcell N.H., Blair N.S., Osinska H., Hambleton M.A., et al: Loss of cyclophilin D reveals a critical role for mitochondrial permeability transition in cell death. Nature 2005; 434: pp. 658-662
- [316]. Nakagawa T., Shimizu S., Watanabe T., Yamaguchi O., Otsu K., Yamagata H., et al: Cyclophilin D-dependent mitochondrial permeability transition regulates some necrotic but not apoptotic cell death. Nature 2005; 434: pp. 652-658
- [317]. Schneider M.D.: Cyclophilin D: knocking on death’s door. Sci STKE 2005; 2005: pp. e26
- [318]. Schinzel A.C., Takeuchi O., Huang Z., Fisher J.K., Zhou Z., Rubens J., et al: Cyclophilin D is a component of mitochondrial permeability transition and mediates neuronal cell death after focal cerebral ischemia. Proc Natl Acad Sci USA 2005; 102: pp. 12005-12010
- [319]. Kim J.S., Ohshima S., Pediaditakis P., and Lemasters J.J.: Nitric oxide: a signaling molecule against mitochondrial permeability transition- and pH-dependent cell death after reperfusion. Free Radic Biol Med 2004; 37: pp. 1943-1950
- [320]. Yoshimoto T., and Siesjo B.K.: Posttreatment with the immunosuppressant cyclosporin A in transient focal ischemia. Brain Res 1999; 839: pp. 283-291
- [321]. Kim J.S., He L., and Lemasters J.J.: Mitochondrial permeability transition: a common pathway to necrosis and apoptosis. Biochem Biophys Res Commun 2003; 304: pp. 463-470
- [322]. Malhi H., Gores G.J., and Lemasters J.J.: Apoptosis and necrosis in the liver: a tale of two deaths? Hepatology 2006; 43: pp. S31-S44
- [323]. Halestrap A.: Biochemistry: a pore way to die. Nature 2005; 434: pp. 578-579
- [324]. Lieberthal W., Menza S.A., and Levine J.S.: Graded ATP depletion can cause necrosis or apoptosis of cultured mouse proximal tubular cells. Am J Physiol 1998; 274: pp. F315-F327
- [325]. Leist M., Single B., Naumann H., Fava E., Simon B., Kuhnle S., et al: Inhibition of mitochondrial ATP generation by nitric oxide switches apoptosis to necrosis. Exp Cell Res 1999; 249: pp. 396-403
- [326]. Lelli J.L., Becks L.L., Dabrowska M.I., and Hinshaw D.B.: ATP converts necrosis to apoptosis in oxidant-injured endothelial cells. Free Radic Biol Med 1998; 25: pp. 694-702
- [327]. Chan F.K., Shisler J., Bixby J.G., Felices M., Zheng L., Appel M., et al: A role for tumor necrosis factor receptor-2 and receptor-interacting protein in programmed necrosis and antiviral responses. J Biol Chem 2003; 278: pp. 51613-51621
- [328]. Mareninova O.A., Sung K.F., Hong P., Lugea A., Pandol S.J., Gukovsky I., et al: Cell death in pancreatitis: caspases protect from necrotizing pancreatitis. J Biol Chem 2006; 281: pp. 3370-3381
- [329]. Cauwels A., Janssen B., Waeytens A., Cuvelier C., and Brouckaert P.: Caspase inhibition causes hyperacute tumor necrosis factor-induced shock via oxidative stress and phospholipase A2. Nat Immunol 2003; 4: pp. 387-393
- [330]. Castaneda M.P., Swiatecka-Urban A., Mitsnefes M.M., Feuerstein D., Kaskel F.J., Tellis V., et al: Activation of mitochondrial apoptotic pathways in human renal allografts after ischemiareperfusion injury. Transplant 2003; 76: pp. 50-54
- [331]. Daemen M.A., de Vries B., and Buurman W.A.: Apoptosis and inflammation in renal reperfusion injury. Transplant 2002; 73: pp. 1693-1700
- [332]. Kelly K.J., Sandoval R.M., Dunn K.W., Molitoris B.A., and Dagher P.C.: A novel method to determine specificity and sensitivity of the TUNEL reaction in the quantitation of apoptosis. AJP – Cell Physiol 2003; 284: pp. C1309-C1318
- [333]. Basile D.P., Liapis H., and Hammerman M.R.: Expression of bcl-2 and bax in regenerating rat renal tubules following ischemic injury. Am J Physiol 1997; 272: pp. F640-F647
- [334]. Ueda N., Kaushal G.P., and Shah S.V.: Apoptotic mechanisms in acute renal failure. Am J Med 2000; 108: pp. 403-415
- [335]. Bonegio R., and Lieberthal W.: Role of apoptosis in the pathogenesis of acute renal failure. Curr Opin Nephrol Hypertens 2002; 11: pp. 301-308
- [336]. Lauber K., Blumenthal S.G., Waibel M., and Wesselborg S.: Clearance of apoptotic cells: getting rid of the corpses. Mol Cell 2004; 14: pp. 277-287
- [337]. Huynh M.L., Fadok V.A., and Henson P.M.: Phosphatidylserine-dependent ingestion of apoptotic cells promotes TGF-beta1 secretion and the resolution of inflammation. J Clin Invest 2002; 109: pp. 41-50
- [338]. Savill J., Dransfield I., Gregory C., and Haslett C.: A blast from the past: clearance of apoptotic cells regulates immune responses. Nat Rev Immunol 2002; 2: pp. 965-975
- [339]. Henson P.M., Bratton D.L., and Fadok V.A.: The phosphatidylserine receptor: a crucial molecular switch? Nat Rev Mol Cell Biol 2001; 2: pp. 627-633
- [340]. Savill J., Gregory C., and Haslett C.: Cell biology. Eat me or die. Science 2003; 302: pp. 1516-1517
- [341]. Li M.O., Sarkisian M.R., Mehal W.Z., Rakic P., and Flavell R.A.: Phosphatidylserine receptor is required for clearance of apoptotic cells. Science 2003; 302: pp. 1560-1563
- [342]. Gregory C.D., and Devitt A.: The macrophage and the apoptotic cell: an innate immune interaction viewed simplistically? Immunology 2004; 113: pp. 1-14
- [343]. Chang M.K., Binder C.J., Miller Y.I., Subbanagounder G., Silverman G.J., Berliner J.A., et al: Apoptotic cells with oxidation-specific epitopes are immunogenic and proinflammatory. J Exp Med 2004; 200: pp. 1359-1370
- [344]. Bratton D.L., and Henson P.M.: Autoimmunity and apoptosis: refusing to go quietly. Nat Med 2005; 11: pp. 26-27
- [345]. Poon I.K., Hulett M.D., and Parish C.R.: Molecular mechanisms of late apoptotic/necrotic cell clearance. Cell Death Differ 2010; 17: pp. 381-397
- [346]. Tabas I.: Macrophage death and defective inflammation resolution in atherosclerosis. Nat Rev Immunol 2010; 10: pp. 36-46
- [347]. Peter C., Wesselborg S., Herrmann M., and Lauber K.: Dangerous attraction: phagocyte recruitment and danger signals of apoptotic and necrotic cells. Apoptosis 2010; 15: pp. 1007-1028
- [348]. Matsuda A., Wu R., Jacob A., Komura H., Zhou M., Wang Z., et al: Protective effect of milk fat globule-epidermal growth factor-factor VIII after renal ischemia-reperfusion injury in mice. Crit Care Med 2011; 39: pp. 2039-2047
- [349]. Harrois A., and Duranteau J.: Acute kidney injury: clear the kidney of apoptotic debris! Crit Care Med 2011; 39: pp. 2180-2181
- [350]. Matsuda A., Jacob A., Wu R., Zhou M., Nicastro J.M., Coppa G.F., et al: Milk fat globule-EGF factor VIII in sepsis and ischemia-reperfusion injury. Mol Med 2011; 17: pp. 126-133
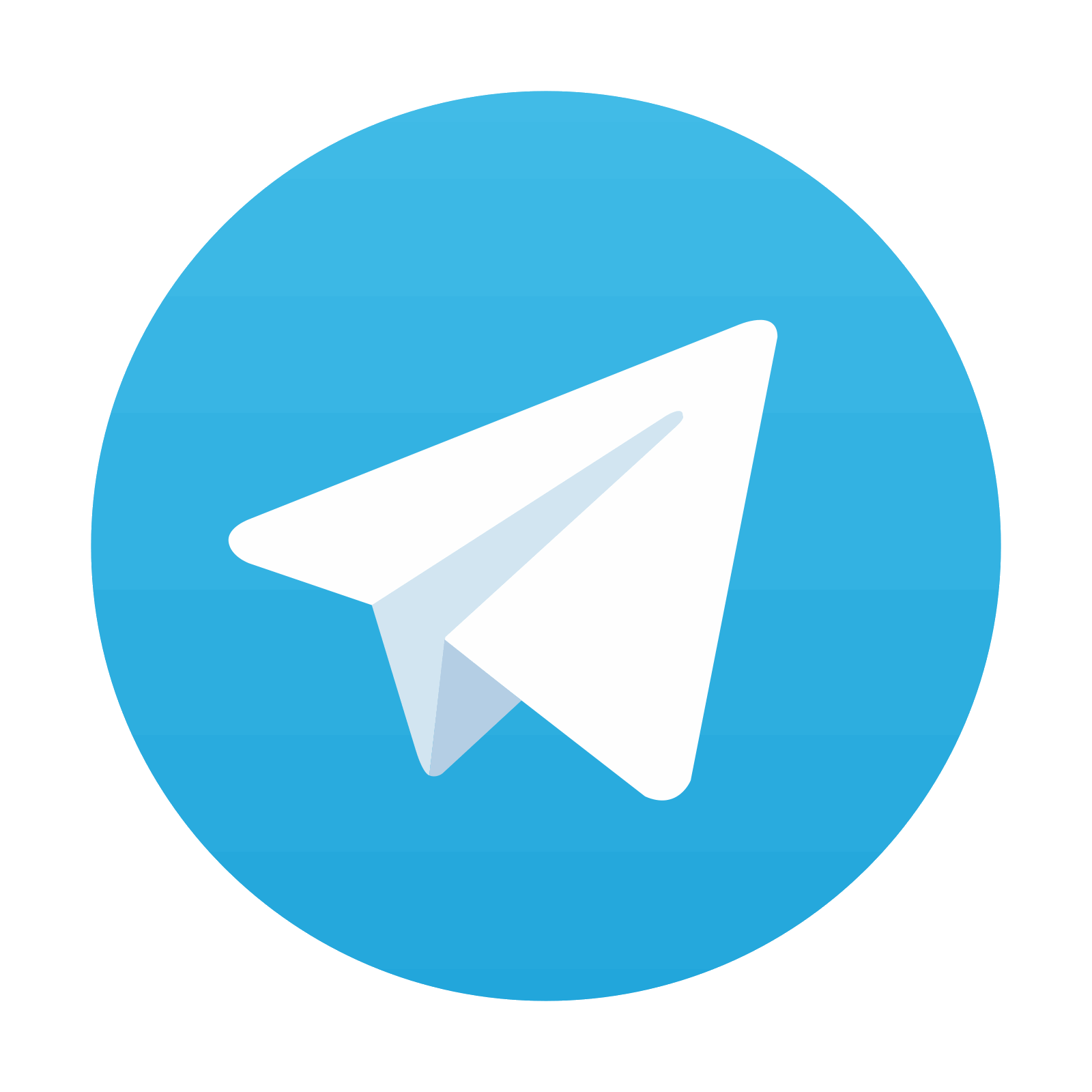
Stay updated, free articles. Join our Telegram channel
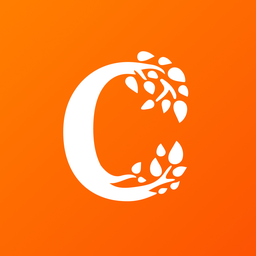
Full access? Get Clinical Tree
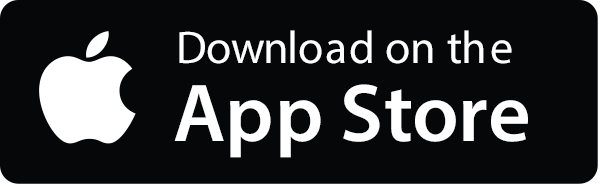
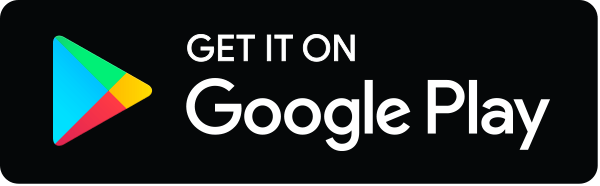