Bengt Rippe
Peritoneal Dialysis
Principles, Techniques, and Adequacy
Peritoneal dialysis (PD) is presently used by approximately 180,000 end-stage renal disease (ESRD) patients worldwide, representing approximately 7% of the total dialysis population.1 In PD the peritoneal cavity, which is the largest serosal space in the body, is used as a container for 2 to 2.5 L of sterile, usually glucose-containing dialysis fluid, which is exchanged four to five times daily via a permanently indwelling catheter. The dialysis fluid is provided in plastic bags. The peritoneal membrane, via the peritoneal capillaries, acts as an endogenous dialyzing membrane. Across this membrane waste products diffuse to the dialysate, and excess body fluid is removed by osmosis induced by the glucose or another osmotic agent in the dialysis fluid, usually denoted ultrafiltration (UF). PD is usually provided 24 h/day and 7 days/wk in the form of continuous ambulatory peritoneal dialysis (CAPD). Approximately one third of the patients in most centers are on automated peritoneal dialysis (APD; sometimes also referred to as continuous cycling peritoneal dialysis [CCPD]), in which nightly exchanges are delivered via an automatic PD-cycler. The use of PD as a modality for ESRD treatment varies widely among countries, mostly because of nonmedical factors such as the reimbursement policy. One study reported that 81% of all dialysis patients in Hong Kong were treated with CAPD or APD in 2006, followed by Mexico (71%) and New Zealand (39%), whereas in the United States, PD was used by only 7.5%, and in Germany by 4.8%.
Advantages and Limitations of Peritoneal Dialysis
If patients or their care givers are competent to undertake PD, the only absolute contraindications are large diaphragmatic defects, excessive peritoneal adhesions, surgically uncorrectable abdominal hernias, or acute ischemic or infectious bowel disease. These and other relative contraindications are discussed further in Chapter 97. PD is best used for patients with some residual renal function, although anuric patients may also do very well. Most patients who start PD will eventually, after several years, transfer to other modalities of renal replacement therapy (RRT), such as hemodialysis (HD), if adequacy cannot be maintained or as a result of other complications, such as recurrent peritonitis or exit site or catheter problems. Only rarely do HD patients transfer to PD, most commonly because of failure to maintain adequate vascular access for HD.
Peritoneal dialysis offers a number of advantages over HD, at least during the first 2 or 3 years of treatment. First, PD represents a slow, continuous, physiologic mode of removal of small solutes and of excess body water, associated with relatively stable blood chemistry and body hydration status. Second, there is no need for vascular access. The absence of vascular access and the absence of the blood-membrane contact of HD make catabolic stimuli less prominent in PD than in HD. Furthermore, residual renal function is somewhat better preserved in PD patients than in HD patients. Because of its continuous nature, PD provides a standardized weekly Kt/V similar to that of thrice-weekly HD despite less efficient small-solute clearance.
Peritoneal dialysis is a home-based therapy, and most patients are trained to do the bag exchanges themselves. In general, home dialysis patients have a better quality of life than those on other types of dialysis. The number of hospital visits is reduced, and the ability to travel is increased. There is also some evidence that PD patients may be better candidates for transplantation than HD patients; several studies have shown a lower incidence and severity of delayed graft function in PD patients after transplantation.2 In children, PD (usually APD) is the preferred dialysis modality because it is noninvasive and socially acceptable, reducing hospital visits and allowing the child to attend school. Advocates of PD often recommend that RRT should ideally begin with PD according to the patient’s choice and then proceed, as required when residual renal function declines, to HD or transplantation. PD should thus be regarded as part of an integrated RRT program, together with HD and transplantation.3 Factors influencing the choice of dialysis modality between PD and HD as well as global variations in the use of PD are discussed further in Chapter 90.
Principles of Peritoneal Dialysis
Three-Pore Model
The major principles governing solute and fluid transport across the peritoneal membrane are diffusion, driven by concentration gradients, and convection (filtration or UF), driven by osmotic or hydrostatic pressure gradients. The barrier separating the plasma in the peritoneal capillaries from the fluid in the peritoneal cavity is represented by the capillary wall and the interstitium. The interstitium can be regarded as a barrier coupled in series with that of the capillary wall; the mesothelium lining the peritoneal cavity is of much less significance as a transport hindrance. For the transport of fluid (UF) and of large solutes, the capillary wall is by far the dominating transport barrier. However, for small-solute diffusion the interstitium accounts for approximately one third of the transport (diffusion) resistance. The permeability of the capillary wall can be described by a three-pore model of membrane transport (Fig. 96-1).4,5 In the capillary wall the major route for small-solute and fluid exchange between the plasma and the peritoneal cavity is the space between individual endothelial cells, the so-called interendothelial clefts. The functional radius of the permeable pathways in these clefts, denoted small pores, is 40 to 50 Å, slightly larger than the radius of albumin (36 Å). The size of these pores markedly impedes the transit of albumin and completely prevents the passage of larger molecules (e.g., immunoglobulins and α2-macroglobulin). However, larger proteins can transit via very rare large pores (radius approximately 250 Å) in capillaries and postcapillary venules. The large pores constitute only 0.01% of the total number of capillary pores, and the transport across them occurs by hydrostatic pressure–driven unidirectional filtration from the plasma to the peritoneal cavity. In addition, the capillary wall has a high permeability to osmotic water transport via aquaporin-1 (AQP-1) channels in the endothelial cell membranes (Fig. 96-2).6
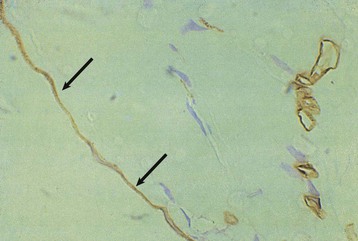
Fluid Kinetics
Under normal (non-PD) conditions most transport occurs via the small pores. Only 2% of peritoneal water transport occurs via AQP-1. In PD, fluid removal is markedly enhanced by infusion of a hyperosmolar dialysate into the peritoneal cavity. The type of osmotic agent used markedly affects the mechanism of osmosis. Glucose will induce fluid flow through both AQP-1 (approximately 45%) and small pores (approximately 55%), whereas large molecules, such as polyglucose (icodextrin) will remove fluid mainly via small pores (approximately 90%). Thus glucose osmosis will result in a rapid dilution of the peritoneal dialysate, as reflected by a fall in sodium concentration (sodium sieving) during the first 2 hours of the dialysate dwell, caused by relatively large transport via the water-only AQP-1 channels; this tends to correct later as diffusion across the small pores eventually increases the sodium concentration to that in the plasma.
Glucose, the commonly used osmotic agent, is usually available at three concentrations: 1.36%, 2.27%, and 3.86%. Figure 96-3, A, demonstrates the intraperitoneal fluid kinetics, computer simulated using the three-pore model, over 12 hours of dwell time with these three solutions. Glucose is an intermediate-size osmolyte with a low osmotic efficiency (osmotic reflection coefficient [σ] = 0.03) across small pores, whereas glucose is 100% efficient as an osmotic agent across AQP-1 (σ = 1). For that reason, glucose will markedly (30-fold) boost the transport of fluid through AQPs and thus redistribute fluid transport away from the small pores toward AQP-1, resulting in significant sodium sieving. For example, for 3.86% glucose in the PD solution, the dialysate Na+ concentration will drop from 132 mmol/l to 123 mmol/l in 60 to 100 min, which later increases toward serum Na+ concentration (Fig. 96-3, B). On the other hand, icodextrin, with an average molecular weight of 17 kd, has a high osmotic efficiency (σ approximately 0.5) across small pores and in relative terms is rather inefficient across AQPs. Hence, during icodextrin-induced osmosis only a very minor fraction of the UF will occur through AQP-1, producing insignificant sodium sieving (see Fig. 96-7, B).
In addition to the size of the osmotic agent, the degree of sodium sieving is dependent on the presence and quantity of AQP-1, and also on the total rate of net UF (which is mainly determined by the glucose concentration), and the diffusion capacity of Na+. A high rate of small-solute transport, and thereby of sodium diffusion, in so-called fast transporters will manifest as rapid equilibration of small solutes (creatinine and glucose) in the peritoneal equilibration test (PET; see later) and will also reduce sodium sieving.
In the absence of an osmotic agent in the PD fluid, the dialysate would be reabsorbed into the plasma within a few hours, mainly driven by the difference in colloid osmotic pressure between plasma and the peritoneum. This absorption will to a major extent occur via small pores, whereas approximately 30% of the peritoneal fluid will be removed by lymphatic absorption. The partial fluid flows in the peritoneal membrane modeled across different fluid conductive pathways in the three-pore model (for 3.86% glucose) are shown in Figure 96-4. It is the presence of relatively high concentrations of glucose in the peritoneal fluid that prevents the reabsorption of fluid into the plasma during the first few hours of the dwell.
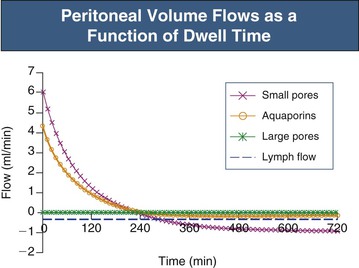
Patients who have a high rate of sodium diffusion (fast transporters) also have rapid transport of glucose out of the peritoneal cavity. Therefore the glucose gradient favoring UF dissipates more rapidly, and it is harder to achieve effective fluid removal compared with “slow transporters.” In fast transporters the maximum UF volume is reduced and UF occurs earlier. There is usually also a more rapid reabsorption of fluid in the late phase of the dwell. The issue of fluid loss from the peritoneum is, however, very controversial, because some authors claim that the peritoneal fluid loss occurring in the late phase of the PD dwell is dominated by lymphatic absorption.7
Effective Peritoneal (Vascular) Surface Area
The functional surface area of the peritoneum reflects the effective surface area of the peritoneal capillaries.8 The transport of small solutes, such as urea, creatinine and glucose, is partly limited by the degree of perfusion of these capillaries—the effective peritoneal membrane blood flow. Furthermore, as mentioned earlier, some of the diffusion resistance for the smallest solutes (urea and creatinine) is located in the interstitium. The number of effectively perfused capillaries is increased by arteriolar vasodilation and reduced by vasoconstriction. These alterations often occur without large changes in the fluid permeability (hydraulic conductance [LpS]) of the peritoneum. Thus, during vasodilation or vasoconstriction there is usually a dissociation between changes in the permeability–surface area product (PS) for small solutes and in the LpS of the membrane. Vasodilation, with recruitment of capillary surface area, occurs early in the dwell when glucose is used as the osmotic agent, causing early, transient increases in PS.9
Peritonitis is also associated with marked vasodilation, again leading to increases in small-solute PS, in the absence of large changes in LpS, during the first 60 to 100 minutes of the dwell. However, in some patients with peritonitis, an increase in LpS will result in relative increased fluid transport across the small pores. Furthermore, there is usually an opening of large pores in the capillaries (and postcapillary venules), resulting in enhanced leakage of macromolecules (e.g., albumin and immunoglobulins) from plasma to peritoneum. Peritonitis may thus result in relative difficulty in removing fluid (because of rapid dissipation of intraperitoneal glucose), a reduced sodium sieving (because of the reduced UF and increased Na+ diffusion), and a markedly increased leakage of proteins to the dialysate.
The contact area between the dialysate and the peritoneal tissue varies as a result of posture and fill volume. Adult patients usually tolerate 2 to 2.5 liters of instilled volume. An intraperitoneal hydrostatic pressure (IPP) of less than 18 cm H2O (supine position) is usually tolerated.10 At higher pressures (>18 cm H2O) the patient usually feels some discomfort. At intraperitoneal volumes of less than 2 liters there is a reduction in small-solute PS, whereas PS is only moderately increased at high fill volumes. Overall, an increased fill volume implies a more efficient exchange with regard to both small-solute exchange and UF, the latter being much more pronounced for hypertonic solutions.11 For a long time it was thought that increased fill volumes would directly affect peritoneal fluid reabsorption by the hydrostatic pressure effect (increases in IPP). However, because 80% of any increase in IPP is transmitted via vein compression back to the capillaries, the actual changes in the transcapillary hydrostatic pressure gradient, which governs UF, will be rather small.12 Thus the impact of IPP on fluid absorption is moderate.13
Peritoneal Access
The key to successful chronic PD is a safe and permanent access to the peritoneal cavity (Fig. 96-5). Despite improvements in catheter survival over the past few years, catheter-related complications still occur, causing significant morbidity and sometimes forcing the removal of the catheter. Catheter-related problems are a cause of permanent transfer to HD in up to 20% of all patients. Most catheters are derived from that originally devised by Tenckhoff and Schechter.14 The Tenckhoff catheter is a Silastic tube with side holes along its intraperitoneal portion. There are usually one or two Dacron cuffs, allowing tissue ingrowth, which secures the catheter in place and prevents pericatheter leakage and infection. The Tenckhoff catheter is straight, having one cuff lying on the peritoneum with the catheter tip pointing in the caudal direction; the outer cuff is close to the skin exit. Several centimeters of the catheter is thus located transcutaneously. Intraperitoneal and transcutaneous catheter modifications continue to appear, indicating that no single design is perfect (Fig. 96-6). Although a number of studies report less frequent catheter drainage failures with use of the arcuate “swan neck” catheter (see Fig. 96-6) compared with straight catheters, there is no hard evidence that any of the modified catheters on the market are actually superior to the original (one- or two-cuff) Tenckhoff catheter.15
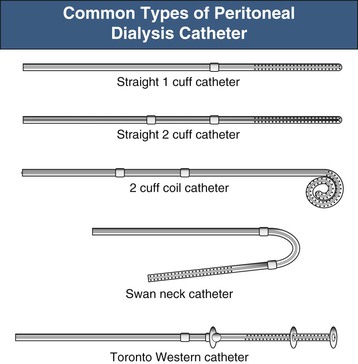
Ideally, catheter insertion should be undertaken under operating room sterile conditions by an experienced operator, either a surgeon or a nephrologist trained in interventional nephrology techniques. Presurgical assessment for the presence of herniation or any weakness of the abdominal wall is essential. If present, it may be possible to correct these at the time of catheter insertion. Before the operation, eradication of nasal carriage of Staphylococcus aureus with locally applied antibacterials (such as mupirocin) significantly reduces exit site infection rates. A single preoperative intravenous dose of a first- or second-generation cephalosporin is also recommended. To avoid development of vancomycin-resistant enterococcus, vancomycin should not be used as a prophylactic agent. Several placement techniques have been described and practiced: surgical mini-laparotomy and dissection, blind placement using the Tenckhoff trocar, blind placement using a guidewire (Seldinger technique), mini-trocar peritoneoscopy placement, and laparoscopy. These techniques are discussed further in Chapter 92.
Techniques of Peritoneal Dialysis
In CAPD, 2 to 2.5 liters of dialysis fluid is instilled into the peritoneal cavity four or five times daily. In 4 to 5 hours there is 95% equilibration of urea and approximately 65% equilibration of creatinine, whereas the glucose gradient has dissipated to approximately 40% of the initial value. For glucose as an osmotic agent, 4 to 5 hours is a suitable dwell time. For night dwell exchanges, longer dwell times can be accepted (8 to 10 hours), although this increases the quantity of glucose and therefore calorie absorption. Furthermore, there is room for individual exchange schedules that can be adjusted to suit individual patient convenience. Dwell times shorter than 4 to 5 hours can be performed with use of a machine (cycler). This technique can be used to maintain adequate dialysis when more frequent lower-volume exchanges are needed—for example, to minimize leakage in conjunction with catheter insertion, hernia repair, or abdominal operations. Rapid exchanges may also be required during treatment for peritonitis, or in patients with fluid overload when the patient’s hydration status needs to be corrected rapidly.
Today, double-bag systems (so-called Y systems) are in general use according to the principle “flush before fill.” The double-bag system contains the unused dialysis fluid connected to an empty sterile drain bag via a Y-set tubing system. After the patient has connected the system and flushed the connection (for 2 to 3 seconds), a frangible (breakable) pin to the drain bag is opened, and the peritoneal cavity is drained over 10 to 15 minutes to fill the drain bag. Then this bag is clamped and the fresh bag opened, so as to fill the peritoneal cavity over another 10 to 15 minutes. The time for exchange (instillation and drainage), if the catheter is in good order, should not exceed a total of 30 minutes. Usually the first 1.6 to 1.8 liters will drain rapidly (at ≥200 ml/min), whereas the last 200 to 300 ml will drain much more slowly. The breakpoint between the rapid and the slow phase may vary markedly from individual to individual.
Automated peritoneal dialysis is usually performed with use of a cycler overnight (8 to 10 hours), during which large volumes (10 to 20 liters) can be exchanged. During the daytime the APD patient usually has a so-called wet day—that is, a long dwell, usually with icodextrin as the osmotic agent in the dialysis fluid. Some patients with nightly APD perform one daily exchange so that there are two long (6- to 8-hour) daily dwells. Most cyclers can be programmed to vary inflow volume, inflow time, dwell time, and drain time. Cyclers usually warm the fluid before inflow, and they also monitor outflow volume and the excess drainage (UF volume). Current APD machines have alarms for inflow failure, overheating, and poor drainage. Some cyclers interrupt drainage at the breakpoint between the fast and slow phases to make the exchange more efficient. Another way to accelerate exchanges is to allow a considerable sump volume in the peritoneal cavity by not letting all the fluid drain; subsequent inflow volumes are proportionally reduced, and after a number of cycles complete drainage occurs. This technique is called tidal peritoneal dialysis (TPD).
The exchange volume should be adjusted according to the patient’s size. Adult patients weighing less than 60 kg should start with 1.5-liter bags. The average patient (60 to 80 kg) should receive 2-liter exchanges, and for patients weighing more than 80 kg, 2.5 liters should be used. If pressure monitoring systems are available, the IPP may inform the choice of exchange volume. In the supine position most patients have an IPP of 12 cm H2O.
Peritoneal Dialysis Fluids
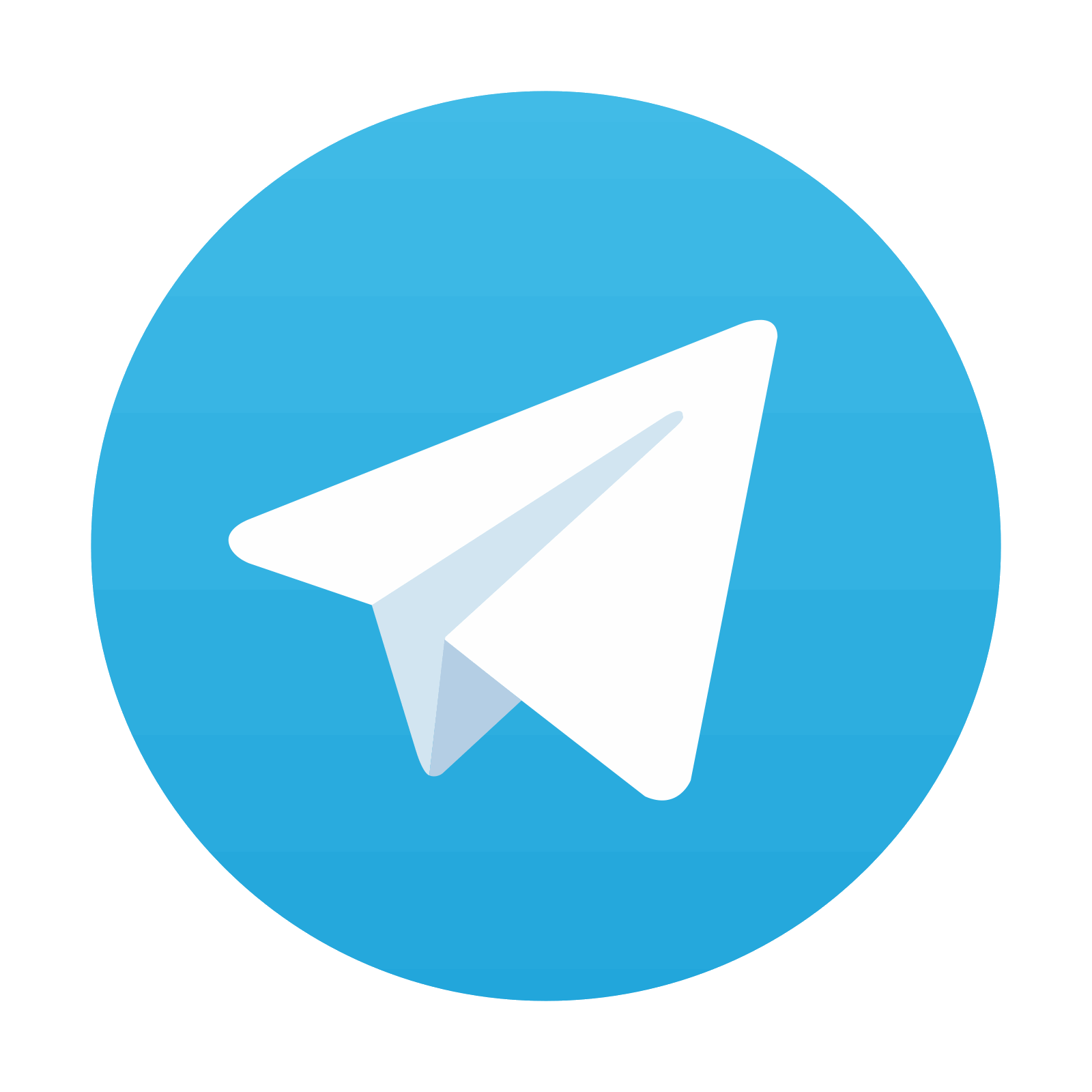
Stay updated, free articles. Join our Telegram channel
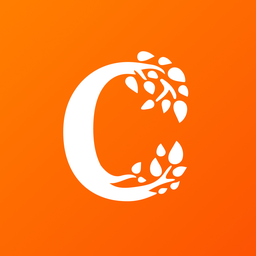
Full access? Get Clinical Tree
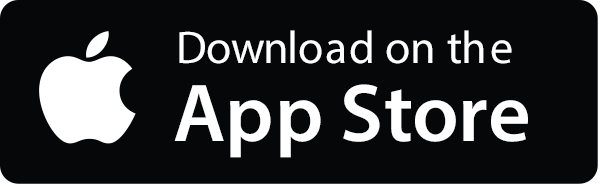
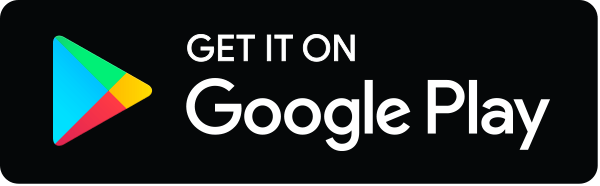