Amino acids and small peptides are very efficiently reabsorbed at the level of the kidney proximal tubule by a series of specialized membrane proteins. In a first step, they are taken up into tubular epithelial cells via secondary active transporters localized at the luminal brush border membrane. Most of these amino acids as well as the amino acids generated by the intracellular hydrolysis of the di-/tripeptides are then transported out of the tubular cell across the basolateral membrane into the interstitial space. This second transport step is mediated by another set of transporters that function as facilitated diffusion pathways and obligatory exchangers. Besides reabsorbing amino acids, proximal tubule cells also contribute to body amino acid metabolism, for instance by producing L-arginine, L-tyrosine and L-serine or by metabolizing L-glutamine to produce ammonia for the urinary excretion of protons. Mutations of genes encoding luminal or basolateral amino acids transporters of proximal tubule cells can cause aminoacidurias and lead in some cases, for instance of cystinuria or lysinuric protein intolerance (LPI), to severe diseases.
Keywords
amino acid transport, proximal tubule, amino acid metabolism, cystinuria, hartnup disorder, lysinuric protein intolerance (LPI), iminoglycinuria, dicarboxilic aminoaciduria, tmem27, ace2
Filtration and Reabsorption of Amino Acids and Oligopeptides
Reabsorption of amino acids is a fundamental and evolutionarily well-conserved mechanism that involves active transcellular transport by specialized transporter proteins localizing to both luminal and basolateral tubular epithelial cell membranes. Approximately 50 g (450 mmol) of free amino acids are filtered daily into the primary urine by human kidney glomeruli. By reabsorbing these amino acids, kidney tubules prevent their loss in the urine. In addition, amino acid metabolism by kidney tubules plays an important role in whole body amino acid homeostasis. Di- and tripeptides are also efficiently reabsorbed from the primary urine with uptake, to a large extent, followed by intracellular hydrolysis to free amino acids and subsequent basolateral export.
Depending on the amino acid, plasma concentration of free amino acids and consequently in the ultrafiltrate varies from low µmol/l up to nearly 1 mmol/l ( Table 71.1 ). For most amino acids reabsorption is ~99.5% efficient and therefore the fractional excretion is less than 0.5% of filtered load. In humans, serine, glycine, histidine, and taurine are notable exceptions for which a higher fraction is lost in the urine ( Table 71.1 ). For several amino acids, a variable fractional excretion is observed, for example, tending to increase when the filtered load increases. The filtered load also depends on the glomerular fluid filtration rate (GFR), which itself is influenced by factors like circadian rhythm, orthostasis, and extracellular volume. Interestingly, by a mechanism not yet understood, renal plasma flow (RPF) and GFR are increased by a high protein diet or infusion of free amino acids. The involvement of glucagon, NO or tubuloglomerular feedback have been suggested as possible mechanisms. As discussed in later sections, differences in the fractional excretion observed for individual amino acids may result from the various selective transporters’ (relative) expression levels, kinetics, ion dependence, and axial localization along the nephron.
Amino acid | Plasma concentration µM (range) 1 | Urine concentration (mmol/mol creatinine, 24 hours) 2 | Fractional excretion (%) 3 |
---|---|---|---|
Alanine | 146–494 | 9–98 | 0.44 |
Arginine | 28–108 | 0–8 | 0.77 |
Aspartic acid | 2–9 | 5–50 | 1.96 + |
Asparagine | 26–92 | 10–65 | 1.54 $ |
Citrulline * | 10–58 | 1–22 | |
Cystine | 24–54 | 2–12 | |
Glutamic acid | 6–62 | 0–21 | 0.68 |
Glutamine | 340–798 | 11–42 | 0.71 |
Glycine | 100–384 | 17–146 | 2.4 |
Histidine | 68–108 | 49–413 | 6.52 |
Isoleucine | 39–90 | 30–186 | 0.51 |
Leucine | 98–205 | 1–9 | 0.26 |
Lysine | 119–243 | 2–16 | 0.92 |
Methionine | 13–37 | 2–53 | 0.66 |
Ornithine * | 36–135 | 1–5 | 0.32 $ |
Phenylalanine | 42–74 | 1–5 | 0.69 |
Proline | 97–297 | 3–13 | 0.047 $ |
Serine | 78–166 | 0–9 | 2.19 |
Taurine * | 18–95 | 18–89 | 6.55 + |
Threonine | 92–197 | 13–587 | 1.04 |
Tryptophan | 25–65 | 6–74 | |
Tyrosine | 26–78 | 3–14 | 1.38 |
Valine | 172–335 | 3–36 | 0.2 |
1 Shih V. Amino acid analysis. In: Blau N DM, Blaskovics ME, ed. Physician’s guide to the laboratory diagnosis of metabolic disease. London: Chapman Hall Medical, 1996:13–30.
2 Venta R. Year-long validation study and reference values for urinary amino acids using a reversed-phase HPLC method. Clin Chem 2001; 47:575–83.
3 Tietze I.N, Sorensen S.S, Eiskjaer H, Thomsen K, Pedersen E.B. Tubular handling of amino acids after intravenous infusion of amino acids in healthy humans Nephrol Dial Transplant 1992;7:493–500.
+ Silbernagl S. Renal handling of amino acids and oligopeptides Contrib Nephrol 1981;24:18–29.
$ Liu W, Lopez JM, VanderJagt DJ, Glew RH, Fry DE, Schermer C, et al. Evaluation of aminoaciduria in severely traumatized patients. Clin Chim Acta 2002; 316:123–8.
Evidence that amino acid reabsorption along the nephron is axially heterogeneous was first derived from clinical observations. Specifically, lesions of the early proximal kidney tubule were observed in Fanconi syndrome, a condition characterized by glucosuria, phosphaturia, and aminoaciduria. Since glucose and phosphate were already known to be reabsorbed in proximal tubules, it was inferred that this nephron segment is also the main site of amino acid reabsorption. The hallmark experiments confirming the role of proximal tubules were carried out using micropuncture and microinjection. Quantification revealed approximately 80% of filtered amino acids are reabsorbed in the first half of the proximal convoluted tubule (S1 segment) whereas less than 1% remains in the tubular fluid at the end of the straight portion (S3 segment) of the proximal tubule.
Concerning the later tubular segments, it was demonstrated by free flow micropuncture that no additional active amino acid transport takes place along the loop of Henle. Furthermore, it was shown by microinjection and microinfusion experiments that with the exception of taurine, amino acids delivered into the early loops of superficial distal nephrons are recovered quantitatively from the final urine. Taken together, these experiments indicated that there is no significant amino acid reabsorption beyond the proximal tubule. One caveat is that a quantitative difference exists between the amino acid fractional delivery values for superficial nephrons reported above and the urinary amino acid fractional excretion. This difference was suggested to be due to the differential absorptive capacity of superficial and deep nephrons.
From “Transport Systems” to Transporters
The transport of free amino acids and small peptides was intensively studied during the second half of the last century thanks to the availability of radiolabeled amino acids and sensitive analytical quantification techniques such as paper chromatography and liquid chromatography. Erythrocyte and cell culture models, as well as tissue and organ experimental systems, in particular small intestine and kidney, were used. From the latter epithelial organs, brush border membrane vesicles were produced that allowed measurement of luminal transports in the presence of defined solutes at their trans-side, i.e., inside vesicles. Prior to the molecular identification of transport proteins, the so-called “transport systems” were functionally defined by amino acid selectivity, stereospecificity, ion-(sodium, chloride, potassium), or proton dependence, thermodynamic properties (equilibrative or concentrative) and transport type (co-transport=symport (S), exchange=antiport (A) or facilitated diffusion=uniport (U). These “transport systems” were named according to functional properties with letters corresponding to the main/first described amino acid substrate, e.g., system A (L-alanine), N (L-asparagine), L (L-leucine), and ASC (L-alanine, L-serine and L-cysteine). In general, capital letters denoted sodium-dependent (except systems L and T) and lowercase sodium-independent systems (e.g., b 0,+ ). The transport of basic or acidic amino acids was identified by a plus or a minus symbol (e.g., system y + L and X − AG ) and the letter B used for systems with a broad amino acid selectivity (for example B 0 , B 0,+ ).
A new era started with the molecular identification of transporters, which was made possible by the complementary techniques of DNA cloning and cellular over-expression. Currently, about 50 amino acid and peptide transporters have been molecularly described. Researchers identifying new amino acid transporters have generally named them according to the “rules” previously used to describe transport systems on occasion resulting in multiple names for the same transporter. The human genome organization (HUGO) proposed a new classification scheme for the genes of solute carriers (SLC) based on sequence homology. This system is now in use for other vertebrate species as well. The SLC gene nomenclature is increasingly used also to name the encoded transport proteins ( Table 71.2 ). The molecular identification of transporters has allowed their detailed functional characterization using cellular overexpression systems, in particular Xenopus laevis oocytes. Additionally, the availability of cDNA allowed measurement of mRNA expression in tissues, initially by Northern blot analysis, and later by real time quantitative RT-PCR (qPCR) and microarray chips. Furthermore, specific antibodies could be generated and used to localize transport proteins at the tissue, cellular and subcellular levels. Noteworthy, the molecular identification of transporters has resulted in the identification of genetic defects underlying several hereditary amino acid transport-related diseases, in particular causing aminoacidurias (discussed in later sections).
Protein name 2 | Slc name 3 | System | Main Substrates | Ion dependency | Transport type | Kidney tubule localization |
---|---|---|---|---|---|---|
APICAL SURFACE LOCALIZATION | ||||||
B 0 AT1 | Slc6a19 | B | Neutral AA | Na + | S | S1 >S2 |
B 0 AT3 | Slc6a18 | Neutral AA | Na + , Cl – | S | S2, S3 | |
b 0,+ -rBAT | 4 Slc7a9-Slc3a1 | b 0,+ | R,K,ornithine, cystine | – | A | S1 >S2 (rBAT also S3) |
PAT2 | Slc36a2 | IMINO | P,G,A, MeAIB | H + | S | S1 >S2 |
SIT1 | Slc6a20 | P, MeAIB | Na + , Cl – | S | S1-3 | |
EAAC1/EAAT3 | Slc1a1 | X – AG | L or D-D, L-E | Na + , H + -S; K + -A | S/A | S2, S3 >S1 |
PEPT1 | Slc15a1 | peptide | di- tri-peptides | H + | S | S1 >S2 |
PEPT2 | Slc15a2 | S3 | ||||
Taut1 | Slc6a6 | taurine, hypotaurine, and β-alanine | Na + , Cl – | S | Collecting duct | |
BASOLATERAL SURFACE LOCALIZATION | ||||||
LAT2-4F2 | Slc7a8-Slc3a2 | L | Neutral AA, but not P, BCH | – | A | S1 >S2 |
LAT4 | Slc43a2 | L,I,M,F,BCH | – | U | (4F2hc also S3) | |
y+LAT1-4F2 | Slc7a6-Slc3a2 | y + L | K,R,Q,H,M,L | Na + for neutral AA | A | S1 >S2 |
TAT1 | Slc16a10 | T | F,Y,W | – | U | S1 >S2 |
Snat3 / SN1 | Slc38a3 | N | Q,N,H | Na + -S; H + -A | S/A | S3, (S2) |
AGT1 / XAT2 | Slc7a13-Slc3a2 | x – | L-D, L-E | – | A? | S3, distal convoluted tubule |
UNKOWN SUBCELLULAR LOCALIZATION | ||||||
Asc1-4F2 | Slc7a10 | asc | G,A,S,C,T | – | A | Loop of Henle, distal and collecting duct |
Asc2 | Slc7a12& | S,G,A,T,L,F,Y | – | Collecting ducts |
1 , Camargo SM, Bockenhauer D, Kleta R. Aminoacidurias: Clinical and molecular aspects. Kidney Int. Apr 2008;73(8):918-925; Verrey F, Closs EI, Wagner CA, Palacin M, Endou H, Kanai Y. CATs and HATs: the SLC7 family of amino acid transporters. Pflugers Arch. Feb 2004;447(5):532–542.
2 The most common names are indicated.
3 Slc names correspond to the gene symbols given by the HUGO gene nomenclature committee.
4 Members of the heterodimeric amino acid transporter (HAT) family are associated to glycoproteins (heavy chains) rBAT (Slc3a1), 4F2hc (Slc3a2) or unknown (&); AA, amino acids (indicated in single letter code); S, symport=co-transport with ion; A, antiport=obligatory exchange against AA or ion; U, uniport=facilitated diffusion pathway. S1-S3, segments of the proximal kidney tubule.
More recently, to address questions about the functional role of amino acid transporters in a physiological context, knockout mice (conventional, tissue-specific and/or conditional) and transgenic mice and rats have been generated. Urine analysis, determination of amino acid fractional excretion, microperfusion, isolated tubule perfusion and other techniques are therefore once again valuable tools for phenotyping genetically-modified animals to increase understanding of the physiological roles of amino acid transporters. In addition, the structure-function relationship of amino acid transporters can be probed using truncated and chimeric constructs, cysteine scanning and so on. Specifically questions could be addressed concerning accessibility of residues, substrate and ion binding sites, allosteric binding domains, tertiary and quaternary structure, and protein interactions. Currently, using bacterial homologues of mammalian transporters, the three dimensional structures of GltPh (PDB 1XFH), a homologue of sodium-dependent glutamate transporters (SLC1 family), and of LeuT Aa (PDB 2A65), a homologue of B 0 AT1/SLC6A19 (SLC6 family), and PEPT(So) (PDB 2XUT), a homolog of the mammalian peptide transporters (SLC15 family) have been solved adding a new level to our understanding of the structure-function relationships of these molecular machines.
Functional Schema of Amino Acid Reabsorption by Proximal Tubule Epithelial Cells
The reabsorption of amino acids by kidney proximal tubules is a transcellular process involving passage across two membranes, namely from the tubular lumen into an epithelial cell, and from the cytoplasm to the extracellular space, for diffusion back into the circulation.
A summary of the current knowledge concerning the arrangement of transporters involved in transepithelial amino acid reabsorption by kidney proximal tubule cells is proposed in the model shown in Figure 71.1 (modified from Verrey et al., 2009). The luminal influx of amino acids is mediated by a set of secondary active transporters driven by the co-transport of Na + or H + and by one heteromeric amino acid transporter that functions as an antiporter, (b 0),+ AT-rBAT (SLC7A9-SLC3A1).

Transporter selectivity for amino acids overlaps to some extent though generally with different affinities for common substrates. For example, neutral amino acids, which represent>80% of the free plasma amino acids, are all transported with different apparent affinities and transport rates by the luminal transporter B 0 AT1 (SLC6A19) ( Fig. 71.1 ). Some amino acids are also transported by the heterodimeric amino acid transporter, b 0,+ -rBAT (SLC7A9–SLC3A1). However, the main function of this exchanger is the import of cationic amino acids and cystine. Furthermore, b 0,+ -rBAT cannot substitute for B 0 AT1 since as an obligatory amino acid exchanger (antiporter) it does not contribute to the overall net amino acid import (see sections on specific transporters below). The imino and neutral amino acid transporters, SIT1, PAT1 and PAT2 also display overlapping substrate selectivity with B 0 AT1. SIT1 (SLC6A20) is an SLC6 family member expressed in the proximal tubule, which in addition to mediating a very efficient L-proline transport, transports other neutral amino acids. PAT2 (SLC36A2) and PAT1 (SLC36A1), SLC36 family proton co-transporter(s), also transport L-proline and small neutral amino acids. Estimation of the relative contribution of each transporter to the actual transport of a given amino acid is very difficult, since this depends not only on the apparent affinity (K 0.5 ) and V max (expression level and transport rate) of each involved transporter and on the amino acid concentration (for the given amino acid), but also on the presence of competing amino acids and compounds. An additional complication is the role of co-transported and/or exchanged substrates, which depending on the transporter are ions, protons, or amino acids. The concentration of co-substrate(s) on both sides of the membrane determines availability and driving force, and thus determines their impact on the amino acid transport rate. Furthermore, in the case of transports driven by an electrical gradient, membrane potential plays an important role.
The majority of the transporters involved in amino acid reabsorption in the kidney are localized along the first two (S1 and S2) proximal tubule segments where most of the amino acid reabsorption occurs. However, some high affinity luminal co-transporters are localized predominantly in the S3 proximal tubule segment, downstream of lower affinity transporters. This is the case for the high affinity Na + -Glucose co-transporter SGLT1 (Slc5a1), which is expressed downstream of low affinity SGLT2 (Slc5a2). Additionally, the high affinity oligopetide transporter PEPT2 (Slc15a2) and the high affinity neutral amino acid transporter B 0 AT3 (SLCA18) are expressed downstream of PEPT1 (Slc15a1) and B 0 AT1 (Slc6a19), respectively. This sequential distribution of low and high affinity transporters along the proximal tubule is suggested to optimize recovery from the ultrafiltrate by permitting absorption of residual solutes and of solutes that have diffused back into the tubular fluid via the relatively leaky paracellular pathway. The various secondary active amino acid transporters expressed in the luminal plasma membrane are all described below in short sections.
The basolateral transport of amino acids is not as well understood as the luminal amino acid influx. This is due to several reasons, a trivial one being that measuring efflux is technically more demanding than measuring influx. The central issue is that the role of basolateral amino acid transport is more complex than that of luminal amino acid uptake. Whereas apical transport aims at clearing the filtered amino acids from the urinary fluid, basolateral transport in addition to transporting the cellular amino acid content into the extracelluar space, also maintains a physiological intracellular amino acid concentration. This is a task that basolateral amino acid transporters of tubular cells have in common with those of non-polarized cells. Indeed, intracellular amino acids are required to fulfil cellular housekeeping tasks and as substrates for amino acid metabolism. Therefore, a relatively stable amino acid gradient from the tubular cells towards the extracellular fluid has to be maintained.
Specifically, some amino acids display similar concentrations within and outside of the cells, while others display an asymmetric distribution. This is particularly evident for anionic amino acids that, despite carrying a charge that should drive efflux, are present at higher concentrations in the cytosol than in the extracellular space. Likewise, in some cells cationic AAs, whose charge should drive influx, are none-the-less not more concentrated in cytosol than in plasma. Two transporters in amino acid transporting epithelial cells that play important roles in the control of various neutral and cationic amino acid intracellular concentrations are the heterodimeric exchangers (antiporters) LAT2-4F2hc (SLC7A8-SLC3A2, neutral amino acid antiporter) and y + LAT1-4F2hc (SLC7A7-SLC3A2, antiporter exchanging intracellular cationic amino acids for extracellular neutral amino acids and Na + ). As obligatory exchangers (antiporters), these transporters can only mediate net vectorial transport of those amino acid substrates that are exchanged for co-substrates recycled back through the membrane by unidirectional amino acid efflux pathway(s). To date, two facilitated diffusion pathway transporters (uniporters), which mediate the directional transport of substrates along their concentration gradient, are proposed to fulfil this function in the basolateral membrane of kidney proximal tubule cells. Specifically, we have shown that the aromatic amino acid uniporter TAT1 (Slc16a10) is present in the proximal kidney tubule basolateral membranes. Using the Xenopus laevis oocyte expression system we demonstrated TAT1 is capable of recycling amino acids to drive the efflux of other substrates by the antiporter LAT2-4F2hc. However, the TAT1 knockout mouse does not show the dramatic changes in urinary amino acid concentrations expected if TAT1 alone recycles amino acids activating efflux via the exchangers LAT2-4F2hc and y + LAT1-4F2hc (unpublished data). Another candidate for the recycling function is LAT4 (SLC43A2), which we have shown also cooperates with LAT2 in the Xenopus laevis oocyte expression system. More work is needed to understand the controlled efflux of amino acids through the basolateral membrane of transporting epithelia.
Finally, it can be hypothesized that given the narrow substrate selectivity of the uniporters known to date, some players that drive net transport of neutral, anionic, and imino acids at the basolateral membrane are not, as yet, molecularly identified.
Transporters Involved in the Luminal Uptake of Amino Acids and Oligopeptides
Neutral Amino Acids
B 0 AT1 (SLC6A19) is the major neutral amino acid transporter. It is a symporter that co-transports Na + and a broad range of neutral amino acids (all proteogenic ones) with low affinity (millimolar range). Unlike many other members of the SLC6 family, B 0 AT1 is not Cl − dependent, and does not transport biogenic amines nor related osmolites. B 0 AT1 mRNA was found to be highly expressed in kidney proximal tubule and small intestine. In both mice and humans B 0 AT1 is expressed in the brush border membrane of early proximal kidney tubules (S1). Another interesting feature of B 0 AT1 is its interaction with two tissue specific accessory proteins. In kidney, B 0 AT1 interacts with the accessory protein, collectrin ( Tmem27 ), and in intestine with the angiotensin converting enzyme 2 ( Ace2 ). Tmem27 is a type I transmembrane protein that appears to interact non-covalently with B 0 AT1. The collectrin (Tmem27 or coll ) knockout mouse lacks renal B 0 AT1 although intestinal expression is normal. Furthermore, tmem27 knockout animals display a massive neutral aminoaciduria without glucosuria or phosphaturia pointing to Tmem27 being the specific obligatory subunit of B 0 AT1 in the kidney. In the intestine, where Tmem27 is not expressed, B 0 AT1 interacts with Ace2. The Ace2 knockout mouse, which has normal kidney expression of B 0 AT1, lacks intestinal B 0 AT1 expression and displays impaired intestinal neutral amino acid transport. The B 0 AT1 knockout mouse, which does not express B 0 AT1 in either kidney or intestine, has a reduced bodyweight. Consistent with B 0 AT1 being the major Na + -dependent neutral amino acid transporter of small intestine and kidney proximal tubule luminal membranes, brush-border vesicles prepared from both tissue sources lack Na + -dependent neutral amino acid uptake. Furthermore, they do not show any compensatory increase in peptide transport and have normal expression of both accessory proteins, namely Tmem27 and Ace2.
B 0 AT3 is structurally and functionally related to SLC6A19 (B 0 AT1). It is highly expressed in apical membranes of kidney proximal tubules with a complementary axial expression vs. B 0 AT1, namely with a higher expression in the later S2 and S3 segments. The SLC6A18 gene is localized on chromosome 5p15 in tandem with the SLC6A19 gene and the two protein products share ~50% identity. Functionally, B 0 AT3 transports a broad range of neutral amino acids in a Na + and Cl − dependent manner with a substrate specificity similar to B 0 AT1, but with higher affinities (micromolar range). As for B 0 AT1, B 0 AT3 requires the accessory protein Tmem27 for renal expression. B 0 AT3 appears to reabsorb residual tubular amino acids, i.e. amino acids not reabsorbed in early proximal kidney tubule segments by B 0 AT1 and/or which have leaked back into the lumen via a paracellular route. Although, in a slc6a18 knockout mouse model an abnormal excretion of several neutral amino acids (especially glycine) was observed, this transporter appears not to play a functional role in humans since a large fraction of the population displays a nonsense single nucleotide polymorphism with no associated phenotype.
Imino and Small Neutral Amino Acids
PAT1 and PAT2 (SLC36A1 and 2) co-transport small neutral amino acids or imino acids with protons. Their functional description was rendered more complicated by the fact that the proton gradient across the luminal membrane of proximal tubule cells depends on the activity of the sodium–proton exchanger NHE3. Therefore, PAT-mediated transport was first erroneously considered to be Na + -dependent. Both PAT1 and PAT2 mRNAs were detected in kidneys but are mainly expressed in other tissues. PAT1 mRNA was found for instance in small intestine, brain, and colon, while PAT2 in lungs, heart, testis, muscles, and spleen. These two transporters have a similar substrate selectivity that includes L- and D-proline, hydroxyproline, glycine, sarcosine, L, D, and β-alanine, D-serine, D-cysteine, betaine, α-(methylamino)isobutyric acid, and GABA. Compared with PAT1, PAT2 prefers L- over D-alanine, does not distinguish between L- or D-serine and has a ten-fold lower affinity for GABA. The major functional difference between these two transporters is that PAT1 displays a low affinity for its substrates (millimolar range), whereas PAT2 is a high affinity transporter (micromolar range). The PAT2 protein was shown to localize at the apical membrane of the early proximal tubule portion in human kidney. Although, PAT1 protein has been localized to the brush border membranes of intestinal enterocytes, in mouse kidney proximal tubule cells only a subapical membrane expression has been shown. No report of a knockout mouse model for either PAT1 or PAT2 has been published.
SIT1 (SLC6A20) functions as a symporter, co-transporting with high affinity (micromolar range) L-proline, methylaminoisobutyrate (MeAIB), methyl-proline, or hydroxyproline with Na + and Cl − . Thus, together with PAT2 and possibly PAT1, SIT1 represents the so called “imino system.” Unlike PAT1 and -2, SIT1 does not transport glycine or β-alanine. The mRNA of SIT1 is highly expressed in small intestine, kidney, lungs, spleen, testis, and brain, and the protein has been shown to localize to the luminal brush border membrane of the entire proximal kidney tubule and of the small intestine. SIT1 has been suggested to interact with the accessory protein Tmem27, since Tmem27 knockout animals showed a much reduced expression of SIT1 in the kidney. Co-expression experiments in Xenopus oocytes show that SIT1 can functionally interact with the accessory proteins Tmem27 and Ace2. SIT1 was also shown to co-localize with Ace2 in human small intestine brush border membranes (Vuille-dit-Bille, Camargo and Verrey, unpublished data). As yet, no knockout mouse model for SIT1 ( slc6a20 ) has been reported.
Cationic Amino Acids and L-Cystine
rBAT (SLC3A1) and b 0,+ AT (SLC7A9) form together a heterodimeric amino acid transporter (HAT). It is composed of the type II glycoprotein (heavy subunit) rBAT and the catalytic subunit (light subunit) b 0,+ AT, that are covalently linked by a disulfide bridge. The light chain, which contains 12 putative transmembrane domains, is the active subunit and confers substrate specificity. Both subunits are primarily expressed in kidney and intestine where they localize to the brush border membrane of proximal tubules or small intestine enterocytes. When over-expressed in an epithelial cell model (MDCK cells) rBAT and b 0,+ AT co-localize in the apical membrane, while in the absence of rBAT, b 0,+ AT is retained intracellularly, demonstrating rBAT is essential for cell surface expression of b 0,+ AT. The rBAT and b 0,+ AT heterodimer mediates the obligatory exchange of large neutral (notably cystine) against dibasic (arginine, lysine, ornithine) amino acids. Mouse models generated by the ablation of the heavy or light chains excrete higher levels of cystine and dibasic amino acids in the urine.
Anionic Amino Acids
EAAC1/EAAT3 (SLC1A1) functions as a high affinity symporter and antiporter. It co-transports L-glutamate, L- or D-aspartate with Na + and H + from the tubule lumen into the cell in exchange for K + that is transported to the outside. The transporter is highly expressed in the later portion of kidney proximal tubules (S2, S3 >S1) and in distal convoluted tubule, the brush border of ileal enterocytes, and in various subpopulations of neurons. EAAC1/EAAT3 is the only anionic amino acid transporter thus far detected in the apical membrane of proximal tubule cells. EAAT3 was shown to have an allosteric negative modulator, the glutamate transport associated protein (GTRAP3–18). GTRAP3–18, which interacts with the carboxy-terminal end of the transporter, is a membrane-associated protein expressed in the same tissues as EAAT3 that has been shown to retard the exit of EAAT3 from the endoplasmatic reticulum. The ablation of Slc1a1 in a mouse model induced a marked urinary excretion of L-glutamate and aspartate, which was not compensated for by other transporters.
Oligopeptides
PEPT1 and PEPT2 (SLC15A1 and 2) are members of the PEPtide Transporter family. These symporters use the proton gradient as the driving force to transport small peptides derived from protein degradation into the cells. PEPT1 and PEPT2 have a broad selectivity accepting all dipeptides and tripeptides composed of the 20 proteinogenic amino acids. The substrate affinity and localization of the two orthologs diverge; PEPT1 has a low affinity (millimolar range) and is expressed in small intestine, kidney, extra hepatic biliary duct and brain, while PEPT2 has a higher affinity (micromolar range) and is expressed in the kidneys, brain, lungs, eyes, and mammary glands. Both transporters are complementarily expressed on apical proximal tubule membranes. The low affinity PEPT1 is highly expressed in the early segments of the proximal tubule and the high affinity PEPT2 in the more distal portion of the proximal tubule (S3). Importantly, PEPTs transport a large number of other molecules, in particular drugs from several important classes such as β-lactamate antibiotics (cephalosporins and penicillins). The transport of sartans and ACE inhibitors is still debated.
Knockout mouse models for both transporters have been generated. Both are viable and have a mild phenotype. The clearance of the non-metabolized peptide glycylsarcosine was not different in a PEPT1 knockout mouse compared with wild type animals, while the intestinal absorption of this substrate was drastically reduced. In contrast, glycylsarcosine clearance was twofold higher in PEPT2 knockout mice than in wild type littermates suggesting that the high affinity PEPT2 is responsible for the majority of peptide reabsorption in the kidneys.
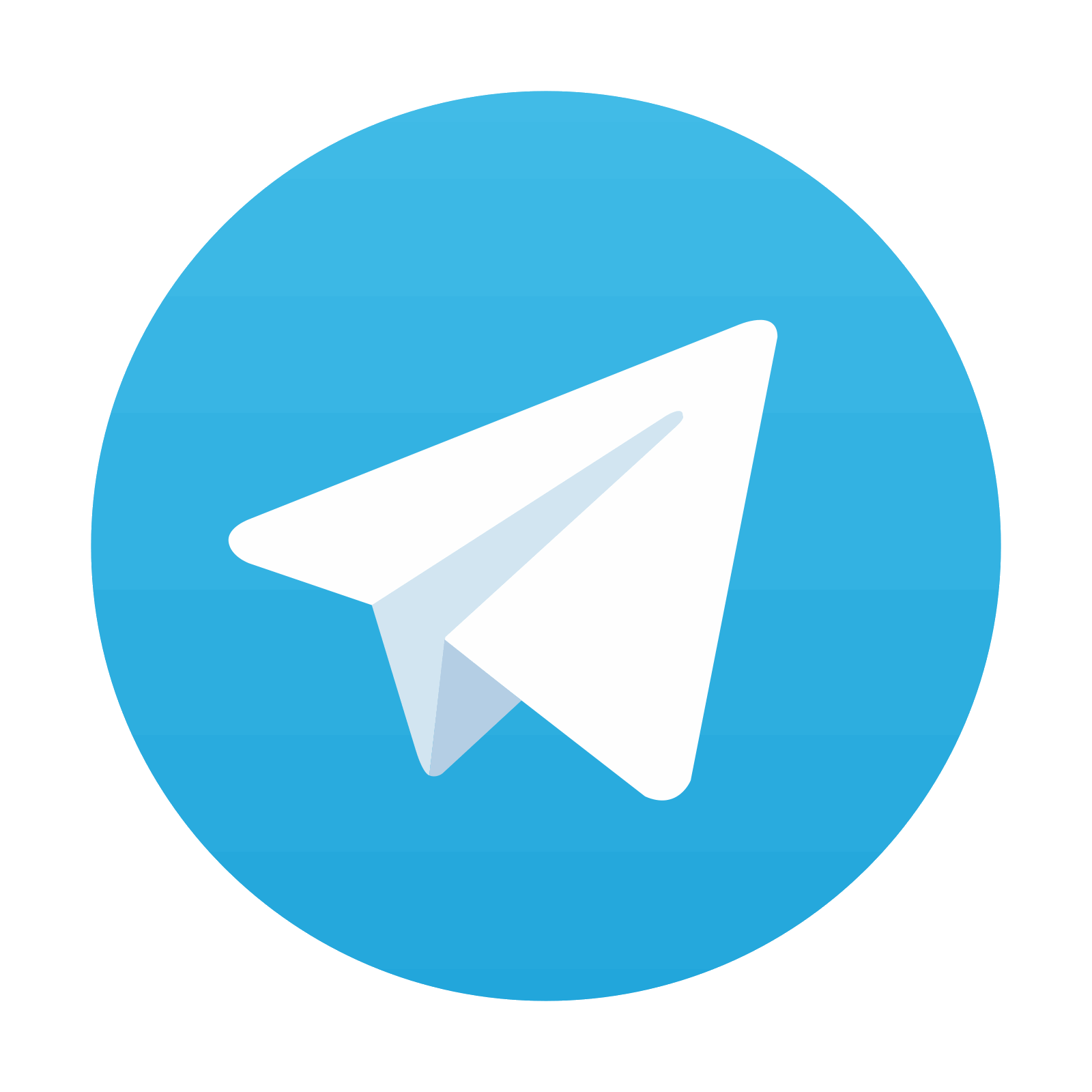
Stay updated, free articles. Join our Telegram channel
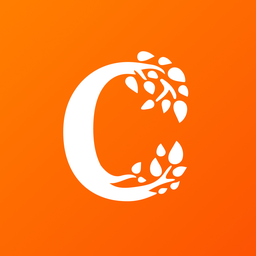
Full access? Get Clinical Tree
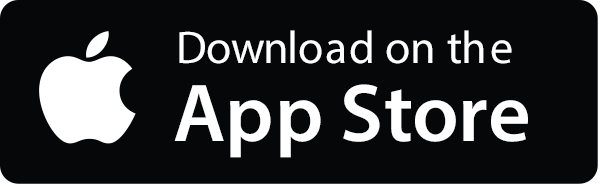
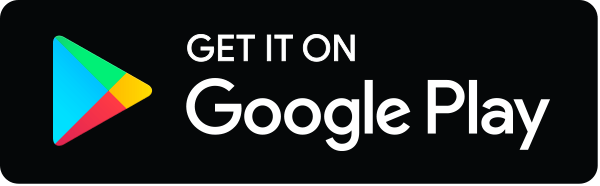