Glucose is the major fuel source to the body’s tissue and its plasma concentration is maintained within narrow limits, 4–10 mM, except in diabetes where it may rise to over 16 mM. In this chapter we review the role of the kidneys in glucose homeostasis. In particular, we discuss the molecular and genetic evidence for the importance of SGLTs and GLUTs in the proximal tubule in the complete glucose absorption from the glomerular filtrate. This leads into a review of the recent developments on a new therapy for diabetes based on SGLT2 inhibitors.
Keywords
structure and function of SGLTs, glucosuria, diabetes: SGLT2 Inhibitors
Overview of Glucose Homeostasis
Glucose is the major fuel source to the body’s tissues, and it comes in various forms in the diet: simple sugar, disaccharides, polysaccharides, and starch. It is utilized in both oxidative and non-oxidative metabolic pathways, it provides a substrate for triglyceride synthesis in adipose tissue, and it is enzymatically polymerized into glycogen for storage in muscle and the liver. Glucose is synthesized endogenously in the liver and kidneys by gluconeogenesis. Its plasma concentration is maintained within narrow limits, 4–10 mM (70–110 mg/dL), with many hormonal influences. Pathological alterations in glucose homeostasis are most often identified with diabetes.
Glucose Transporters in glucose Homeostasis
The transport of glucose across biological membranes is a critical step regulating its concentration in plasma. There are two major classes of proteins responsible for glucose transport.
Facilitated GLU cose T ransporters (GLUTs) bind to glucose and translocate it across membranes in an energy-independent fashion: they require a glucose concentration gradient to drive net transport; hence they facilitate the diffusion of glucose. The GLUTs belong to the SLC2A gene family, part of the larger Membrane Facilitators Superfamily (MFS). There are currently 14 identified GLUT genes within the human body, for example, the protein coded by the SLC2A2 gene , GLUT2, allows glucose to be reabsorbed in the kidney by facilitating diffusion across basolateral membranes, and mutations in this gene are responsible for the Fanconi-Bickel Syndrome (FBS, OMIM 227810).
S odium-dependent GL ucose T ransporters (SGLTs) bind Na + ions and glucose, transporting them across the membrane in an energy-dependent fashion: the Na + electrochemical potential gradient, maintained by the Na + /K + ATPase, drives the transport of glucose into cells. This allows non-metabolized glucose analogs to accumulate in tissues in excess of the plasma concentration and so they are crucial for the reabsorption of glucose in the kidneys and the intestinal absorption of dietary glucose. SGLTs belong to the SLC5A gene family, part of the Sodium Solute Symporter (SSS) superfamily of proteins. Although the amino acid sequences are quite different among members of the SSS family, the atomic structures reveal common features that suggest similar transport mechanisms.
As with the GLUTs, SGLT genes are expressed throughout the body. However, mRNA expression profiles offer only hints of physiological function, and establishing functional roles for SGLTs in the body’s tissues has proven to be non-trivial.
Cellular and Molecular Physiology of SGLTs
SGLT1 was the first identified and is the most extensively studied Na + /D-glucose co-transporter. It was cloned in 1987 by expression cloning, and it is responsible for the intestinal absorption of D-glucose and D-galactose from the diet. Through a combination of biophysics, immunohistochemistry, molecular genetics, and freeze fracture electron microscopy, it was established that either defective translation, post- translational trafficking, or function of SGLT1 leads to glucose-galactose malabsorption (GGM, OMIM # 182380; online, Mendelian Inheritance in Man, http://www-ncbi-nlm-nih-gov.easyaccess1.lib.cuhk.edu.hk/OMIM ). GGM is an extremely rare, autosomal recessive disease, in which patients experience life-threatening osmotic diarrhea starting in the first few days of life. Failure to absorb sugars broken down from the lactose in mother’s milk or infant formula leads to profuse, watery diarrhea that abates only when sources of glucose or galactose are eliminated from the diet. As long as GGM is identified and appropriate dietary modifications are made, patients lead relatively normal and healthy lives. Its expression cloning and detailed characterization, in addition to the molecular details of GGM, firmly established hSGLT1 as crucial to glucose and galactose absorption in the gut. On the other hand patients with GGM have only a mild renal glycosuria (see below).
There are four other members of the SLC5A gene family that are capable of transporting glucose, SGLT2, SGLT4, SGLT5 and SGLT6. Human SGLT3, which is expressed in gut neuronal cells, depolarizes the cell upon exposure to glucose, is believed to be a glucose sensor and not a transporter. hSGLT6 is a Na + /myo-inositol co-transporter which also is a low affinity glucose transporter and it is now referred to as hSMIT2. hSGLT2 (SLC5A2) is abundantly expressed in the kidney but mRNA is also found in liver, heart, brain and muscle. This is believed to be the transporter responsible for the bulk of glucose reabsorption in kidney and mutations in the gene are associated with renal glucosuria (see below). In addition to D-mannose, hSGLT4 has also been shown to transport D-glucose, fructose, and 1,5-anhydro-D-glucitol. Its mRNA has been detected in small intestine, kidney, liver, lung and brain, where it may be important in maintaining stores of mannose for protein glycosylation. SGLT5, exclusively expressed in the human kidney cortex, is a mannose and fructose sodium cotransporter with a higher affinity and turnover than for glucose. Its cellular location and function within the cortex is as yet unknown.
SGLTs and Renal Glucose Reabsorption
180 g/day of D-glucose is filtered across the glomerulus into the kidney in humans, yet less than <1% of this filtered load ends up in the urine in healthy persons. What is responsible for the efficient reabsorption of glucose, and where is this accomplished within the kidney?
In what has been described as one of the most important contributions to renal physiology, Wearn and Richards developed the free-flow micropuncture technique in 1924. In their first study on frog nephrons, they detected plasma levels of sodium and glucose in the “glomerular urine” but not in the “bladder urine,” proving “beyond doubt that the reabsorption of these substances must take place in the renal tubules.” By introducing oil blocks and perfusing pipettes in subsequent work, specific tubular regions were quantitatively perturbed and analyzed. With such a novel approach, they showed that nearly all glucose was reabsorbed within the proximal tubule, and this could be blocked by phlorizin.
Micropuncture and single nephron analyses were subsequently adapted for the study of mammalian kidneys, demonstrating that, in rats, glucose reabsorption occurred in the proximal tubule with sensitivity to phlorizin. It was previously established that intravenous administration of phlorizin inhibited renal glucose reabsorption in humans by up to 100%.
In 1970, using sensitive fluorometric-enzymatic methods for measuring D-glucose and inulin, as well as Lissamine green for more precisely locating nephron segment puncture sites and tubular transit times, detailed studies of glucose reabsorption could be performed in rat nephrons. These free-flow micropuncture studies demonstrated that ≈98% of D-glucose reabsorption occurred within the PT, and most reabsorption occurred within the first 3 mm from the glomerulus ( Figure 70.1 ); no measurable net glucose reabsorption occurred distal to the PT. A follow-up study showed that glucose competed with [ 3 H]-phlorizin for the same (and at that time) as-yet-unknown “binding site” in isolated brush borders of rat kidney, with reversibility, stereospecificity (D-glucose over L-glucose), and Na + -dependence. Renal D-glucose reabsorption was attributed to active Na + /sugar co-transport, similar to and consistent with Crane’s hypothesis that Na + -dependent transport drives intestinal absorption of D-glucose and D-galactose, which he articulated in a groundbreaking 1960 presentation in Prague.

Barfuss and Schafer expanded on these early studies by making quantitative estimates of D-glucose absorption in isolated, perfused segments of early and late PT from rabbit nephrons. They found that in early segments, maximal [ 14 C]-D-glucose absorption (J max ) was high, with a relatively low affinity for glucose (K m ≈ 2 mM). In comparison, later segments showed a 10-fold reduction in J max , with higher affinity for glucose (K m ≈ 0.4 mM). From this, they concluded there were at least two distinct systems of glucose reabsorption in the proximal tubule. Detailed studies using PT brush border membrane vesicles from human and rabbit kidneys showed more evidence of two transport systems: an early, low affinity (K m glucose=6 mM) transporter with 1:1 Na + :D-glucose stoichiometry and a later, high affinity (K m glucose=0.3 mM) transporter with 2:1 stoichiometry.
After the two renal sodium glucose cotransporters were characterized, attention turned to their molecular identification In 1992, low-stringency hybridization screening of human kidney cDNA libraries, using rabbit and human SGLT1 sequences as probes, led to the identification of a clone bearing 59% DNA sequence identity to hSGLT1. When cRNA of the clone was injected into Xenopus oocytes, [ 14 C]-α-methyl-D-glucopyranoside (αMDG, a SGLT-specific glucose analog) accumulation was 2- to 3-fold higher than that observed in water-injected oocytes. αMDG transport was Na + -dependent and phlorizin-sensitive, sugar affinity was low compared to hSGLT1, D-galactose was not a substrate, and Na + :glucose stoichiometry was 1:1. In the same study, Na + /glucose co-transport was also reported to be electrogenic and voltage-dependent, although the magnitude of maximal currents observed under voltage-clamp were <1% of those seen in hSGLT1-injected oocytes; but they were 2-fold larger than currents from control (H 2 O-injected) oocytes. From this, Kanai and colleagues concluded it was not possible to claim hSGLT2 is a high capacity transporter per se , but rather its high level of mRNA in early PT segments—measured by in situ hybridization—might correspond to abundant protein expression at the luminal membrane; and hSGLT2 proteins as an ensemble might operate with a high capacity for glucose reabsorption. They further suggested that hSGLT1’s 2:1 Na + :glucose coupling, which endows it with greater accumulative power than a transporter with 1:1 stoichiometry, along with its high affinity for glucose, allows it to salvage the remaining tubular glucose in the late PT.
Subsequent studies of rat SGLT2 demonstrated substrate specificity (i.e., lack of D-galactose transport) and an additional estimate of 1:1 Na + :glucose coupling. In situ hybridization and northern blot analyses revealed SGLT2 message predominantly in outer kidney cortex while SGLT1 was found within inner cortical and outer medullary regions. Based on: (1) previous work showing the bulk of glucose reabsorption occurs in the early PT, (2) fluxes in isolated tubules showing evidence of two transport systems, and (3) spatial differences in SGLT1 and 2 mRNA expression, it was concluded that SGLT2 is the major Na + /D-glucose co-transporter in the kidney.
Recently our biophysical characterization of cloned human SGLT1 and SGLT2 expressed in HEK293 cells confirmed and extended the previous oocyte studies: the apparent glucose affinities (K 0.5 ) were much more similar than expected, 1.8 and 4.9 mM for SGLT1 and SGLT2; Galactose was a poor substrate for SGLT2 with a K 0.5 greater than 100 mM; and phlorizin was a more potent blocker of SGLT2 than SGLT1 with a Ki of 11 vs 140 nanomolar. The higher affinity of SGLT2 for phlorizin was mostly due to a slower off rate.
There are several reports on SGLT protein localization within the rat kidney. In the first, SGLT1 and GLUT1 antibodies were tested in various sections—inner medulla, outer medulla-inner segment, outer medulla-outer segment, and cortex. SGLT1 immunoblotting revealed signal in cortical and outer medullary regions, whereas GLUT1 signal was observed in inner and outer medulla but not cortex. Immuno-fluorescent, -peroxidase, and -gold staining identified SGLT1 signal in all segments—S1, 2, and 3—of PT on apical membrane surfaces, whereas GLUT1 reactivity was observed on basolateral membrane surfaces of S3 PT as well as more inner (outer and inner medullary) regions. A second study examined SGLT1 and GLUT2 distribution; in cortex, SGLT1 (on apical membranes) and GLUT2 (on basolateral membranes) showed co-reactivity in 40% of tubules. Moreover, SGLT1 signal was found in the majority of cortical PTs, based on co-staining with the PT marker, anti-γ-GTP. In a third study SGLT1 was localized on the brush border membrane of the proximal tubule with increasing intensity from the S1 to the S3 segments. There was substantial intracellular staining in the cells of the S1 segment and, surprisingly, there was SGLT-related staining in the apical domains of the macula densa and adjacent cortical parts of the thick ascending limb of Henle. Apart from the fact that different SGLT1 antibodies were used in these studies there is no simple explanation for these subtle differences in SGLT1 distribution. So far there is only one paper reporting on the immunolocation of SGLT2 in the rodent kidney. Here there was specific SGLT2 antibody staining of apical membranes of the early proximal convoluted tubule of the mouse and this was not observed in the SGLT2 −/− mice.
Overall, we may conclude that there are two SGLT genes expressed in the kidney, SGLT2 in the brush border membrane of the early proximal tubule and SGLT1 in the brush border membrane of the late proximal tubule. Furthermore the localization of SGLTs to the apical membrane and GLUTs to the basolateral membrane provides plausible cellular mechanisms for glucose reabsorption in kidney ( Figure 70.2 ). However, for human kidney there still are no immunocytochemical studies of SGLT2 and SGLT1 distribution and no physiological studies on isolated tubules.

While the atomic structure of human SGLTs is not yet known, the structure of a bacterial homolog vSGLT with 32% amino acids identity to hSGLT1 has been solved and refined to 2.7 Å ( Figure 70.3 ). As expected from biochemical studies the structure contains 14 transmembrane helices, and we also find one galactose molecule bound to the center of the protein occluded from the aqueous solutions on each side of the membrane. The most notable features of the structure are: i) the inverted repeat (TM1–5 and TM6–10) forming the core of the protein. While these repeats can be superimposed there is no amino acid homology between them; ii) there are two discontinuous membrane helices, TM1 and TM6, at the core of the sugar binding sites; and iii) hydrophobic gates occlude the sugar from the external solutions, external gates, M73, Y87 and F424 and the internal gate Y263. The pyranose sugar ring is stacked against the aromatic ring of the inner gate. Molecular dynamic studies show that after sodium release into the cytoplasm the tyrosine side chain rotates to a different position to allow the release of sugar to the cytoplasm.

The high amino acid identity between vSGLT and hSGLT1, including the conservation of the sugar and sodium coordinating residues and the gates, has facilitated the generation and testing of a hSGLT homology model. Mutation of the glucose binding residues produces profound reductions in the apparent sugar affinity (K 0.5 ), e.g. the mutation E102C increases the glucose K 0.5 from 0.5 to >100 mM. Evidence was also obtained for the opening of the external gates by external sodium, i.e., external methanethiosulfonate reagents and dyes only reached cysteine mutants in the sugar binding site when sodium was present in the external medium, and this access was blocked by sugar and phlorizin. This provides direct evidence that sodium/glucose cotransport is controlled by the coordinated opening and closing of the external and internal gates. Much structural work remains to be done to fully understand the atomic mechanism of transport.
Genetic Ablation of SGLTs
Studies of transgenic mice deficient in SGLT2 (SGLT2 −/− ) and SGLT1 (SGLT1 −/− ) have shed new light on the relative roles of SGLT2 and 1 in renal glucose reabsorption in vivo . In each of the three SGLT2 −/− studies, absolute urinary glucose excretion was 60–70% of the filtered load in knock-out (SGLT2 -/- ) mice, compared with 0% in WT mice. This implies that SGLT2 is of major importance in renal glucose reabsorption, but in the absence of functional SGLT2, there is still significant renal glucose reabsorption.
In the first study, detailed micropuncture experiments of cortical (i.e., superficial ) nephrons were performed. Early proximal convoluted tubular collections demonstrated 80% reabsorption of the filtered glucose load in WT mice, compared with 0% in SGLT2 -/- mice. In later PT collections, the fractional reabsorption was 90% in wild type mice and 20% in knock-outs. It was also found that the filtered glucose load was proportional to the rate of excretion in knock-out mice, but independent of the load in WT. A major role for SGLT2 in glucose reabsorption was clearly established by this work; yet there was a significant discrepancy between superficial cortical fluid collections, which suggested SGLT2 is responsible for ≥80% of glucose reabsorption, and whole kidney glucose excretion measurements suggesting SGLT2 was responsible for 60% of glucose reabsorption. The results of this study make clear the importance of considering nephron heterogeneity in defining the role of Na + /D-glucose co-transporters in renal glucose reabsorption.
A second study looked at mice deficient in SGLT2 and how they handled streptozotocin-induced diabetes. Interestingly, SGLT2 −/− mice showed dramatically increased mortality—70% compared with 10% in wild type—due to sepsis from pyelonephritis. From this work, new questions about the safety of hSGLT2 inhibitors have emerged, which warrant further study.
In a third study, the SGLT2 −/− strain used by Vallon et al. was crossed with db/db— diabetic, obese, hyperlipidemic—mice. The resulting double transgenics had better glycemic control in both the fed and fasting states, they were less obese, and they displayed improved pancreatic β-cell health—increased cell mass (60%) and reduced cell death—compared with db/db mice alone. Also, single transgenic (SGLT2 -/- ) mice fed a high fat diet were protected from developing hyperglycemia, hyperinsulinemia, and glucose intolerance, when compared with their wild-type littermates on the same diet.
Taken together, these studies suggest that SGLT2 plays a significant role in glucose reabsorption, and genetically ablating SGLT2 can result in dramatic improvement of hyperglycemia and associated sequelae in T2DM. Indeed these knock-out studies are consistent with using SGLT2 inhibitors as a viable insulin-independent approach to treating type 2 diabetes mellitus (see below). It is also clear that there are significant compensatory mechanisms of glucose reabsorption in the absence of SGLT2, capable of reabsorbing up to 40% of the filtered load. What are the molecular players behind such compensation? One possibility is that hSGLT1 serves a robust role in the absence of SGLT2.
In awake SGLT1 −/− mice the urinary glucose concentration was significantly increased (from ~0 to 20 mM) and the fractional reabsorption was reduced 3% in the absence of any apparent changes in SGLT2 mRNA or protein expression. Micropuncture experiments on the last surface loop of proximal convoluted tubules showed that there was an increase in both the delivery and concentration of glucose in SGLT1 -/- mice relative to wild-type mice: from 2 to 4 pmole/min and from 0.4 to 0.8 mM. These studies suggest that SGLT1 in the late proximal tubule does play a role in mopping up the last remaining glucose in the glomerular filtrate. As expected the most obvious phenotype of the SGLT1 -/- mice is glucose-galactose-malabsorption, but the surprise is that unlike human GGM this phenotype only develops after weaning.
Genetics of Renal Glycosuria
Familial renal glycosuria (FRG, OMIM # 233100 and 182381) is an autosomal recessive condition characterized by urinary glucose excretion with normal plasma glucose levels, oral glucose tolerance tests, and plasma insulin levels. Patients absorb glucose and galactose normally, and they have no known defects in glucose utilization in tissues. Long before any of the SGLT genes were identified, work was well underway to characterize FRG. In 1969, renal glucose titrations on two families with hereditary renal glycosuria showed that affected individuals typically had a lowered threshold (F mingG ) for glycosuria and reduced T max (characteristic of “type A FRG”), and in one instance an exaggerated splay (characteristic of “type B FRG”). Importantly, sugar fluxes in jejunal mucosa samples demonstrated no difference in hexose transport between FRG patients and normal controls, i.e. intestinal glucose absorption was unaffected in FRG. There were also hints that inheritance of glycosuria was autosomal recessive, conflicting with the dogma of the time that FRG was inherited in autosomal dominant fashion. Further pedigree and physiological analyses of three generations from a single family established autosomal recessive inheritance as well as an intermediate phenotype of mild glycosuria in heterozygote family members.
In more recent years, given the proposed role for hSGLT2 in renal glucose reabsorption, researchers analyzed the gene encoding hSGLT2 ( SLC5A2 ) and found it was associated with hereditary glycosuria. Further studies confirmed SLC5A2 ’s involvement in a single individual with glycosuria, and across multiple families, many with consanguineous unions. In patients with either homozygous or compound heterozygous SGLT2 mutations, glucose excretion ranged from 15 to 200 g/day; and, consistent with previous hypotheses, heterozygote family members frequently displayed glycosuria of ≤4 g/day. Follow-up studies of patients with combined severe glycosuria and aminoaciduria revealed a novel missense mutation in hSGLT2, and aminoaciduria has been described in another case report of a patient with FRG. The aminoaciduria was not immediately explained and was believed due to the severity of glycosuria rather than an intrinsic defect in amino acid reabsorption.
Analyses of SLC5A2 mutations in families with FRG have revealed several noteworthy trends. First, most mutations identified in FRG are private: in 23 families with FRG, 21 unique mutations were observed; and in another large analysis, 17 families yielded 20 unique mutations. One mutation, a splice-site mutation (“IVS 7+5 g >a”), was observed in nine different families, with index cases showing either homozygosity or compound heterozygosity with this mutation; it was speculated that due to the high prevalence of this mutation, the region encoding the splice-site is a mutational “hot spot.” Second, many of the mutations producing pronounced glycosuria (>20 g/d) are predicted to result in either premature stop codons (by point or frame-shift mutations) or deletions/insertions resulting in truncated proteins. There is precedent for this in GGM, where mutations in SGLT1 resulted in proteins which were non-functional or failed to traffic to the plasma membrane.
There is significant heterogeneity in urinary glucose excretion among individuals with FRG. In patients homozygous or compound heterozygous for mutations in SLC5A2 , excretion ranged from 10 to 200 g/d; some of this may be due to the difference between mutations resulting in partially-functional hSGLT2 protein versus those which produce non-functional or non-trafficked protein. However, in at least one instance, two members of the same family with the same compound heterozygous genotype (R137H, Δ358-8) had remarkably different excretion profiles: 200 g/d for the first index case, and 80 g/day for the second. Such a finding hints at major differences in compensatory mechanisms of renal reabsorption in the setting of partially or completely impaired hSGLT2 function.
In general, FRG patients have no other phenotypic findings other than increased urinary glucose excretion: they do not suffer from hypoglycemia or any other impairment in glucose homeostasis, they do not have alterations in electrolyte excretion or significant changes in volume status, and long-term follow-up of one patient with severe glycosuria showed no alterations in renal function. That said, in one recent study, elevated urinary glucose excretion was linked to mild diuresis in two patients with severe glycosuria: plasma renin activity and serum aldosterone were elevated to 3–4-fold above normal, suggesting volume and/or electrolyte loss accompanies glycosuria. Among the group of mutation analyses and clinical case report data, FRG appears to be linked exclusively to mutations in hSGLT2 further cementing its significant role in glucose reabsorption in the kidney.
A second genetic disorder of renal glucose reabsorption is the rare Fanconi-Bickel Syndrome (FBS) (MIM 227810) linked to mutations in the facilitated glucose transporter 2 (GLUT2). A major part of renal glucose reabsorption—facilitated diffusion of glucose across the basolateral membrane—is impaired ( Figure 70.2 ). Interestingly, GLUT2 does not appear to be essential for intestinal glucose absorption in mice and in FBS patients (see ). FBS is described as a glycogen storage disease with hepatic and renal overload. Symptoms include hypoglycemia, hypergalactosemia, failure to thrive, and hypophosphatemic rickets. In addition, patients show generalized aminoaciduria and metabolic acidosis. With frequent carbohydrate feeds, phosphate and bicarbonate supplementation, patients lead relatively normal lives, although they are short in stature.
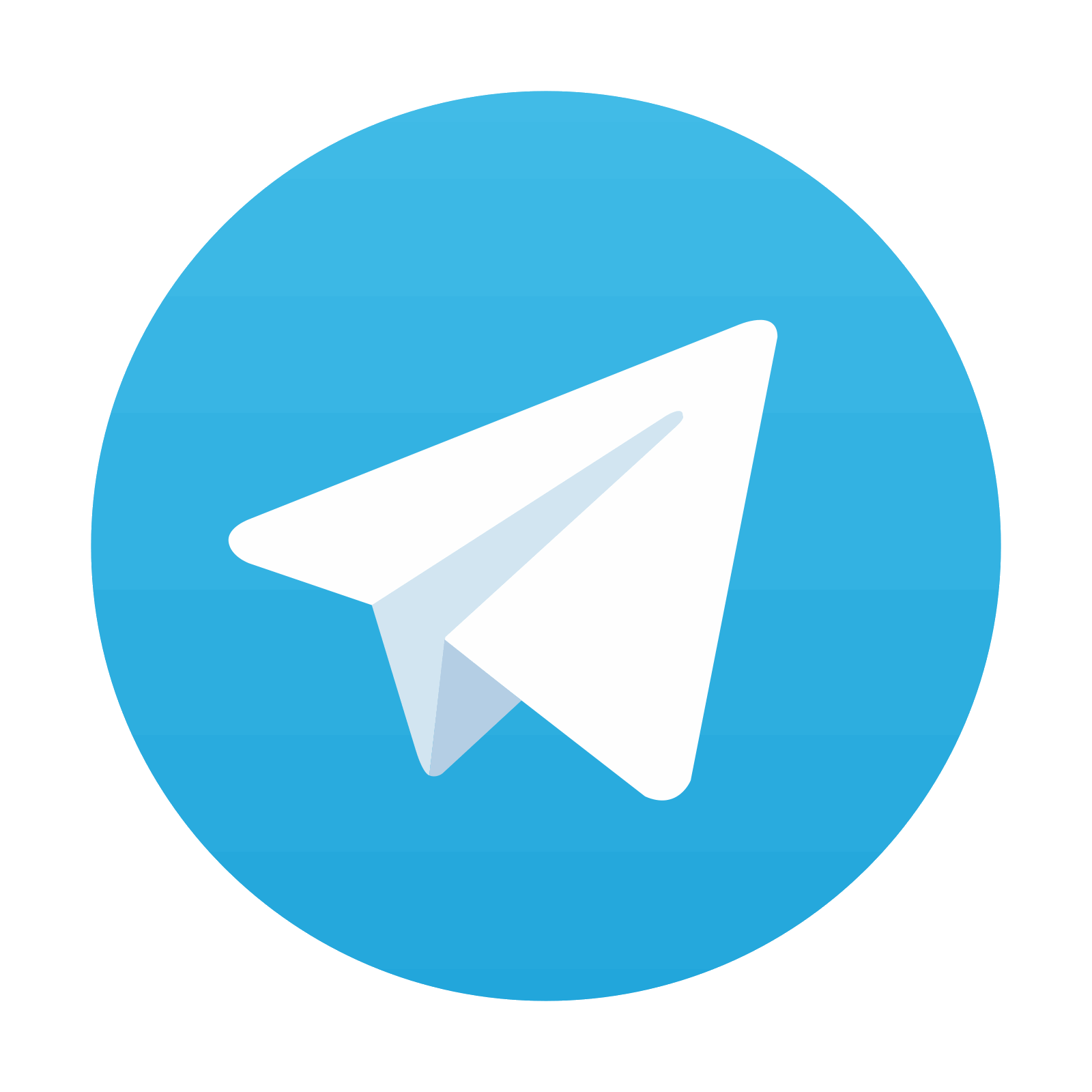
Stay updated, free articles. Join our Telegram channel
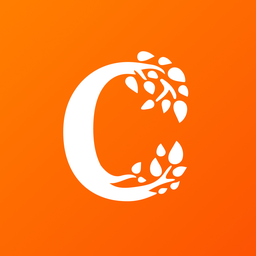
Full access? Get Clinical Tree
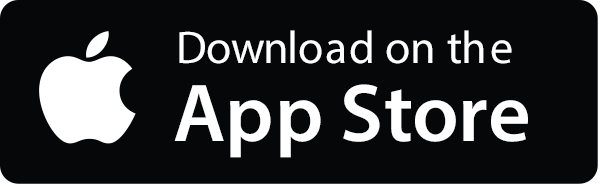
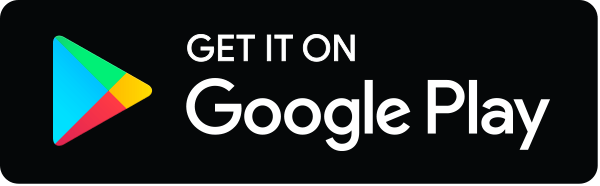