Hyperkalemia is a common electrolyte disorder that may cause life threatening cardiac arrhythmia. The trans-cellular distribution of K + limits the acute rise in P K . Regulation of K + excretion by the kidney maintains overall K + balance. To shift K + into cells requires an increase in cell interior negative voltage, this is achieved by activating the electrogenic Na-K-ATPase pump. Insulin causes the translocation of Na-K-ATPase pump units into the cell membrane. A higher concentration of H + in the ICF compartment and insulin activate NHE-1 and increase the electroneutral entry of Na + into cells. ß- 2 adrenergic agonists increase the affinity of Na-K-ATPase for Na + .
Control of K + secretion occurs primarily in the late cortical distal nephron (CCD). Two factors influence the rate of excretion of K + ; the flow rate in the terminal CCD and the net secretion of K + by principal cells in the CCD. Secretion of K + requires electrogenic Na + , i.e., reabsorption more Na + than Cl − in CCD. Aldosterone actions lead to an increase in the number of open ENaC units in luminal membrane of principal cells in CCD. A complex network of with-no-lysine kinases (WNKs) via effects on Na/Cl cotransporter (NCC) in DCT and ROMK normally function as a switch to change the aldosterone response of the kidney to either conserve sodium or excrete potassium, depending on whether the release of aldosterone is induced by a change in dietary Na + or dietary K + intake. An increased delivery of <SPAN role=presentation tabIndex=0 id=MathJax-Element-1-Frame class=MathJax style="POSITION: relative" data-mathml='HCO3−’>HCO−3HCO−3
HCO3−
HCO−3
HCO 3 −
and/or a rise in the pH of luminal fluid in CCD may provide a signal to augment the secretion of K + . In the presence of vasopressin action, the number of osmoles in its luminal fluid determines the flow rate in the terminal CCD. Urea recycling aids the excretion of K + ; this process is especially important in subjects with disorders or those who are taking drugs that lead to a less lumen-negative voltage in the CCD.
It is imperative to recognize when hyperkalemia represents a medical emergency because therapy must take precedence over diagnosis. Treatment of emergency hyperkalemia is to antagonize the cardiac effects of hyperkalemia with the infusion of Ca 2+ , to induce a shift of K + into the ICF, and to remove K + from the body. In patients with life-threatening hyperkalemia, there is no role for the use of cation exchange resins. To induce an appreciable K + loss via the GI tract over a relatively short period of time, the goal of therapy should be to induce diarrhea.
If hyperkalemia developed over a short period of time or in a setting of a low K + intake, its cause is a shift of K + out of cells or pesudohyperkalemia. To assess the renal response in a patient with hyperkalemia, we use the ratio of the concentrations of K + and creatinine in a spot urine sample (U K /U Creatinine ). A lower than expected U K /U Cr in a patient with hyperkalemia is most commonly due to a less negative voltage in the lumen of the CCD due to a lower rate of electrogenic reabsorption of Na + . This could be due to disorders that lead to a diminished rate of reabsorption of Na + via ENaC, or disorders that lead to reabsorption of Na + and of Cl − in CCD at near equal rates. The basis of a lower rate of reabsorption of Na + includes low P Aldosterone levels, blockade of the aldosterone receptor in principal cells, molecular defects that diminish the number of ENaC units in the luminal membrane of the CCD, the presence of cationic compounds in the lumen of the CCD that block ENaC. The site of the lesion that leads to reabsorption of Na + and Cl − at near-equal rates in the CCD might be in the early DCT where there is enhanced electroneutral reabsorption of Na + and Cl − via NCC. The defect might be also due to enhanced electroneutral reabsorption of NaCl in CCD; a “chloride shunt disorder.”
In patients with chronic hyperkalemia, decreasing K + intake and the use of diuretics to increase flow in the terminal CCD seems to be an effective strategy. Patients who have disorders and/or are taking drugs that significantly affect the ability to generate trans-epithelial lumen negative voltage in their CCD, are dependent on maintaining a high flow rate in their terminal CCD to maintain K + balance without a need for a higher P K . If the patient were given a diuretic and became intravascular volume-depleted, the increase in NaCl reabsorption in the proximal convoluted tubule will decrease the number of osmoles of Na + and Cl − in the lumen of the terminal CCD. In this regard, restricting protein intake may decrease the amount of urea recycling and hence the volume of fluid that exits the terminal CCD. The role of induction of bicarbonaturia with the use of acetazolamide needs to be examined.
Keywords
Hyperkalemia, K + shift, K + secretion, causes of hyperkalemia, emergency hyperkalemia, treatment of hyperkalemia, aldosterone , WNK kinases, Familial Hypertension with Hyperkalemia
Introduction
Hyperkalemia is a common electrolyte disorder that may have detrimental effects, the most serious of which is a cardiac arrhythmia. There is large variability among patients in the absolute concentration of potassium (K + ) ions in plasma (P K ), which leads to electrocardiographic changes and cardiac toxicity of hyperkalemia. From a diagnosis of the cause of hyperkalemia perspective, the basis of hyperkalemia will be a shift of K + out of cells if the time course for its development is short and/or if there is little K + intake. On the other hand, chronic hyperkalemia implies that there is a defect in the regulation of the excretion of K + by the kidney.
Basic Concept for the Movement of K + Ions Across Cell Membranes
K + is the principal cation in cells. The movement of K + ions across cell membranes has two requirements; first, there must be a driving force; second there must be an open K + channel in a cell membrane.
Driving Force
K + are kept inside the cell by an electrical force; the cell interior has a negative voltage. It follows that a more negative cell voltage is required to shift K + into cells. The negative voltage in cells is generated by the activity of the electrogenic cation pump, the Na + , K + ATPase (Na-K-ATPase). This pump exports three sodium (Na + ) ions while importing only two K + ions; hence there is a net export of {1/3} of a positive charge per Na + that exits from cells via the Na-K-ATPase ( Figure 51.1 ). Because, intracellular anions are largely macromolecular phosphates such as RNA, DNA, and phospholipids, which remain inside the cell, a negative intracellular voltage is generated when Na + exits the cell via the electrogenic Na-K-ATPase.

There are three ways to acutely increase ion pumping by the Na-K-ATPase: First, a rise in the concentration of its rate-limiting substrate—intracellular Na + ; second, an increase in the affinity for Na + or in the V max of the existing Na-K-ATPase units in cell membranes; third, an increase in the number of active Na-K-ATPase pump units in the cell membrane by recruitment of new units. A chronic increase in Na-K-ATPase pump activity requires the synthesis of new pump units as occurs with exercise training.
The driving force for the secretion of K + by principal cells in the late distal convoluted tubule, the connecting segments and the cortical collecting duct (the abbreviation CCD is used in this Chapter to indicate all these nephron segments) is a lumen-negative voltage. This lumen-negative voltage is generated by the electrogenic reabsorption of Na + (reabsorption of more Na + ions than of their accompanying anion, chloride (Cl − ) ions).
Ion Channels for K +
There are several different types of channels that permit the diffusion of K + through cell membranes. Some of these channels are regulated by voltage, others by ligands such as calcium ions (Ca 2+ ), and yet others by metabolites such as adenosine di-phosphate (ADP)—the latter are called K ATP channels. Because K + ions do not reach diffusion equilibrium, control is exerted not only by modulating the number but also the conductance and gating of these channels.
The major K + channel that adjusts the intracellular voltage is the K + ATP channel. It is a misnomer to call these channels K + ATP as the concentration of ATP in cells does not change sufficiently to provide a signal for regulating these channels. The signal that regulates these channels may be the result of a change in the concentration of a related nucleotide, ADP. When the concentration of ADP rises, K + ATP channels will open; conversely, when the concentration of ADP falls, K + ATP channels will close. This regulation of K + ATP channels adjusts the negative voltage in cells, which in turn regulates other ion channels. The best example is the voltage-gated Ca 2+ channel, which is opened by a less negative voltage in cells. Ionized Ca 2+ is a major signal for regulation of a number of intracellular processes.
Example : Regulation of the K + ATP channel by ADP plays a critical role in the release of insulin. When the concentration of glucose in plasma (P Glucose ) is high, a metabolic signal is generated in ß-cells of the pancreas to cause the release of insulin ( Figure 51.2 ). When the rate of oxidation of glucose rises in ß-cells, the concentration of ADP in these cells falls. This lower concentration of ADP causes the K + ATP channels to close and thus the ICF compartment becomes less negative because fewer K + ions (positive charges) exit ß-cells. This less negative voltage in ß-cells increases the conductance of voltage-gated Ca 2+ channels and hence the intracellular concentration of ionized Ca 2+ rises—this provides the signal to release insulin from ß-cells ( Figure 51.2 ).

A similar logic can be used to deduce how the blood flow to exercising muscle may be controlled using intracellular Ca 2+ as the signal ( Figure 51.3 ). During a sprint, L-lactic acid is released from skeletal muscle cells. When lactic acid enters vascular smooth muscle cells, H + and/or lactate anions lead to the opening of the K + ATP channels. As a result, K + exit and the voltage in these cells become more negative. This causes closure of voltage-gated Ca 2+ channels and less entry of Ca 2+ into cells and hence vasodilatation.

Several types of K + channels including renal outer medullary K + channels, ROMK (K ir 1.1) (which have similar biophysical prosperities and regulatory mechanism as that of the small conductance K + channels identified in mouse and rat CCD), a Ca 2+ activated big-conductance K + channel (BK) and the double-pore K + channel, are expressed in the apical membrane of principal cells in CCD. ROMK are the K + channels most important for the secretion of K + in CCD. Big K + conductance “BK” or maxi-K + channels are activated by a rise in intracellular Ca 2+ and are thought to play an important role in flow dependent K + secretion. Although these channels likely mediate K + secretion in patients with Bartter’s syndrome that is due to a loss of function mutation in ROMK, their role in physiological regulation of renal excretion of K + is not clear. BK channel activity has been detected in both principal cells and intercalated cells in CCD. It is difficult to explain the contribution of BK located in intercalated cells to K + secretion as these cells have low Na-K-ATPase pump content. BK channel activity is increased in the CCD from rats on a high K + diet. Furthermore, in BK-α subunit knocked mice, increase in flow failed to stimulate K + secretion in distal nephron. Notwithstanding, deletion of BK-α subunit does not affect net K + excretion in mice fed a high K + diet. It is important to recall that the role of these channels was examined in laboratory rodents which consume 10-fold more K + per kg body weight and close to three-fold more Na + per kg body weight compared to human subjects consuming a typical Western diet. Therefore, it is possible that the BK channel may provide a way for a “speedy” K + excretion if there is a large intake of K + and if flow through CCD were augmented. Although, the same limitations imposed by the magnitude of the lumen negative voltage on the rate of secretion of K + by these channels will apply, it is possible that “conductance” through ROMK may be rate limiting when there is a need for a high rate of excretion of K + over a short period of time.
Regulation of K + Homeostasis
Regulation of K + homeostasis has two important aspects:
1. Control of the trans-cellular distribution of K + , which is vital for survival because it limits acute changes in the P K .
2. The regulation of K + excretion by the kidney, which maintains overall K + balance; nevertheless, this is a much slower process.
Acute Control of the Plasma K +
Distribution of K + between the ECF and ICF Compartments
The Na-K-ATPase
The activity of the Na-K-ATPase is higher when the concentration of its substrate, Na + in cells rises. The impact of this increase in the net exit of Na + on the trans-membrane voltage, however, depends on whether the Na + entry step into cells is electroneutral or electrogenic.
Electroneutral Entry of Na + into Cells : This occurs when Na + ions enter cells in exchange for hydrogen ions (H + ) via the Na + /H + exchanger-1 (NHE-1) ( Figure 51.4 , left side). The NHE-1 is normally inactive in cell membranes, as can be deduced from the fact that the concentrations of its substrates (Na + in the extracellular fluid (ECF) and H + in the intracellular fluid (ICF) compartment) are considerably higher than that of its products (Na + in the ICF compartment and H + in the ECF compartment) in steady state. The two major activators of NHE-1 are a higher concentration of H + in the ICF compartment near NHE -1 and insulin. Monocarboxylic acids (e.g., L-lactic acid) enter cells on the monocarboxylic acid cotransporter (M-CT) ( Figure 51.4 ). If the M-CT is located in close proximity to NHE in a cell membrane, the release of H + from these acids may cause a high concentration of H + in the local area of NHE-1, which activates NHE-1 and hence increases the electroneutral entry of Na + into cells ( Figure 51.4 , left side).

HCO3−
HCO−3
HCO 3 −
will be exported and Cl − enter cells. The intracellular negative voltage will drive the exit of Cl − ; from cells through their specific ion channel. This latter step will lead to the export of negative voltage from cells and the subsequent exit of K + .
Electrogenic entry of Na + into Cells : The Na + channel in cell membranes is normally gated by voltage. When open, one cationic charge enters the cell per Na + transported. Since only 1/3 of a charge exits per Na + ion that is exported out of the cell via the Na-K-ATPase ( Figure 51.1 ) ; the net effect would be to diminish the degree of intracellular negative voltage and this leads to net exit of K + out of cells. This explains a rise in the P K during intense exercise. It could explain the development of hyperkalemia in patients with hyperkalemic periodic paralysis.
Hormones that Affect the Distribution of K +
Catecholamines : FXYD1 (phospholemman) interacts with the catalytic α subunit of the Na-K-ATPase and modulates its activity. Un-phosphorylated FXYD1 binds to the α subunit and this inhibits the pump activity by decreasing its affinity for Na + and/or its V max . ß- 2 adrenergic agonists activate adenalyate cyclase and this leads to stimulation of the conversion of ATP to cAMP. This second messenger, in turn, activates protein kinase-A, which induces phosphorylation of the FXYD1; this disrupts its interaction with α subunit of the Na-K-ATPase and results in an increase in the affinity of the Na-K-ATPase for intracellular Na + . The increase in export of pre-existing intracellular Na + ( Figure 51.1 ) increases the negative voltage in cells and causes a shift of K + into cells .
An acute shift of K + into cells and hypokalemia is observed in conditions associated with a surge of catecholamines (e.g., patients with a subarachnoid hemorrhage, myocardial ischemia, and/or an extreme degree of anxiety). ß 2 -agonists may be used to induce a shift of K + into cells in patients with hyperkalemia in an emergency setting. Non-specific ß-blockers are being used for therapy of the subtype of hypokalemic periodic paralysis associated with hyperthyroidism.
Insulin : Insulin increases the activity of the Na-K-ATPase and its expression in the plasma membrane through a phosphoinositide 3-kinase, atypical protein kinase C (aPKC), and extracellular signal regulated kinases 1 and 2 (ERK1/2). ERK1/2 kinase phosphorylation of the Na-K-ATPase α subunit promotes translocation of Na-K-ATPase from an intracellular pool to the cell membrane. aPKC phosphorylation of FXYD1 leads to an increase in the V max of the Na-K ATPase. Insulin may also have an effect in augmenting the electroneutral entry of Na + into cells via NHE-1. This, in conjunction with increasing the number and activity of the Na-K-ATPase on the cell surface, causes the voltage in cells to become more negative ( Figure 51.4 , left side). Notwithstanding the glucose transporter, GLUT4 and Na-K-ATPase do not co-localize to the same skeletal muscle intracellular vesicles and hence, the effect of insulin to shift K + into cells is separate from its effect on glucose entry into these cells.
Insulin has been utilized clinically in the treatment of patients with an emergency due to the adverse cardiac effects of hyperkalemia. In contrast, in patients with diabetic ketoacidosis, a lack of action of insulin results in a shift of K + out of cells and the development of hyperkalemia despite a total body deficit of K + .
Acid–Base Influences
When an acid is added to the body, most of the H + are buffered in the ICF compartment. Monocarboxylic acids (e.g., L-lactic acid) enter cells on the M-CT, and, as mentioned above, release of their H + near NHE-1 may activate NHE-1 and increase the electroneutral entry of Na + into cells and hence, in the presence of insulin, may cause a shift of K + into cells.
A shift K + out of cells may occur in patients with metabolic acidosis due to acids that are not transported on MC-T (e.g., metabolic acidosis due to a gain of HCl acid owing to loss of NaHCO 3 in a patient with diarrhea, ingestion of citric acid ). A possible mechanism to explain how this may occur is illustrated in ( Figure 51.4 , right side ) . Activation of the Cl − /bicarbonate ( <SPAN role=presentation tabIndex=0 id=MathJax-Element-3-Frame class=MathJax style="POSITION: relative" data-mathml='HCO3−’>HCO−3HCO−3
HCO3−
HCO−3
HCO 3 −
) anion exchanger (AE) will cause <SPAN role=presentation tabIndex=0 id=MathJax-Element-4-Frame class=MathJax style="POSITION: relative" data-mathml='HCO3−’>HCO−3HCO−3
HCO3−
HCO−3
HCO 3 −
to exit and Cl − to enter cells. Since cells have Cl − channels in their membrane, the rise in the concentration of Cl − in the ICF in conjunction with the usual negative voltage forces Cl − to exit. As this exit of Cl − is electrogenic, it causes a less negative voltage in cells and K + will exit from cells.
Several clinical implications follow from this analysis:
- 1.
If hyperkalemia is present in a patient with metabolic acidosis due to accumulation of monocarboxylic organic acid, other causes for hyperkalemia than the acidemia should be sought (e.g., lack of insulin in patients with diabetic ketoacidosis, tissue injury, and a lack of ATP to drive the Na-K-ATPase in patients with L-lactic acidosis due to hypoxia ).
- 2.
Metabolic acidosis due to inorganic acids (addition of HCl) causes a shift of K + out of cells. Patients with chronic hyperchloremic metabolic acidosis (e.g., patients with chronic diarrhea or those with renal tubular acidosis (RTA)) however, usually have a low P K because of excessive loss of K + in the diarrhea fluid in the former or the urine in the later.
Respiratory acid–base disorders, on the other hand, cause only small changes in the P K as there is little movement of Na + or Cl − across cell membranes in these disorders.
Tissue Catabolism
Hyperkalemia may be seen in patients with crush injury or those with tumor-lysis syndrome. In these patients, factors that compromise the ability of the kidney to excrete K + are usually present as well. Patients with diabetic ketoacidosis, have a total body K + depletion but hyperkalemia is commonly present due to a shift of K + from cells secondary to a lack of insulin. The corollary is that during therapy, complete replacement of the deficit of K + must await the provision of cellular constituents (phosphate, amino acids, Mg 2+ , etc.) and the presence of anabolic signals.
Long-Term Regulation of K + Homeostasis
Control of the renal excretion of K + maintains overall daily K + balance. Although the usual intake of K + in adults eating a typical western diet is close to 1 mmol of K + /kg body weight, the rate of excretion of K + can match an intake of more than 200 mmol/day with only a minor rise in the P K .
Control of K + secretion occurs primarily in the CCD. Two factors influence the rate of excretion of K + in CCD; the flow rate in the terminal CCD, and the net secretion of K + by principal cells in the CCD which raises the luminal concentration of K + ([K + ] CCD ).
K + Secretion
The secretory process for K + by principal cells in the CCD, has two elements. First, a trans-epithelial lumen negative voltage must be generated by electrogenic reabsorption of Na + via epithelial Na + channels (ENaC) ( Figure 51.5 ). Second, open ROMK channels must be present in the luminal membrane of principal cells.

HCO3−
HCO−3
HCO 3 −
or an alkaline luminal pH) is shown to the right of the dashed vertical line.
Aldosterone actions lead to an increase in the number of open ENaC units in the luminal membrane of principal cells in CCD ( Figure 51.5 ). The steps involved include binding of aldosterone to its receptor in the cytoplasm of principal cells, entry of this hormone-receptor complex into the nucleus, and then the synthesis of new proteins including the serum and glucocorticoid regulated kinase-1 (SGK-1). SGK-1 increases the expression of ENaC in the apical membrane of principal cells. The mechanism seems to be related to the effect of SGK-1 to phosphorylate and inactivate the ubiquitin ligase Nedd-4-2. Nedd-4-2 ubiquinates ENaC subunits, leading to their removal from the cell membrane and degradation in proteasomes. Therefore, inhibition of the Nedd-4-2 induced endocytosis of ENaC leads to increased expression of ENaC in the luminal membrane, which increases the transport of Na + . Another mechanism by which aldosterone activates ENaC involves proteolytic cleavage of the channel by serine proteases. Aldosterone induces production of “channel activating proteases” (CAP 1–3). These proteases activate ENaC by increasing their open-probability, rather than by increasing their expression in the luminal membrane.
The driving force for Na + reabsorption in the CCD is a higher concentration of Na + in the lumen of CCD than that in principal cells (~10–15 mmol/L) and a negative voltage inside cells, owing to the actions of the Na-K-ATPase at the basolateral membrane of these cells. The concentration of Na + in luminal fluid is limited by the presence of urea osmoles and that the osmolality of luminal fluid in CCD will be equal to plasma osmolality in the presence of vasopressin. The rate of electrogenic Na + reabsorption is limited by constraints on the magnitude of luminal negative voltage that can be generated in CCD because of limitations on transport of Cl − . At a trans-epithelial lumen negative voltage of −61 mV and a plasma Cl − of 100 mmol/L, the minimum luminal Cl − concentration would be 10 mmol/L. Therefore, under most circumstances, the concentration of Na + in luminal fluid is sufficient for the activity of ENaC. Variations in the luminal concentration of Na + in the CCD do not regulate the net secretion of K + .
The reabsorption of Na + in the CCD can be electroneutral, if the rate of reabsorption of its major accompanying anion Cl − matches its rate of reabsorption, or electrogenic, if more Na + than Cl − are reabsorbed in CCD ( Figure 51.5 ). The pathway(s) for the reabsorption of Cl − in the CCD is (are) not well defined, but it is thought that paracellular pathways play an important role. Recently an electroneutral, thiazide-sensitive and amiloride-resistant Na + -Cl − transport was identified in the intercalated cells of the cortical collecting duct in mice. This seems to be mediated by the parallel activity of the Na + -dependent <SPAN role=presentation tabIndex=0 id=MathJax-Element-6-Frame class=MathJax style="POSITION: relative" data-mathml='Cl−/HCO3−’>Cl−/HCO−3Cl−/HCO−3
Cl−/HCO3−
Cl−/HCO−3
Cl − / HCO 3 −
exchanger (NDCBE), which promotes the electroneutral exchange of one intracellular Cl − with one Na + , two <SPAN role=presentation tabIndex=0 id=MathJax-Element-7-Frame class=MathJax style="POSITION: relative" data-mathml='HCO3−’>HCO−3HCO−3
HCO3−
HCO−3
HCO 3 −
and the Na + independent <SPAN role=presentation tabIndex=0 id=MathJax-Element-8-Frame class=MathJax style="POSITION: relative" data-mathml='Cl−/HCO3−’>Cl−/HCO−3Cl−/HCO−3
Cl−/HCO3−
Cl−/HCO−3
Cl − / HCO 3 −
exchanger (pendrin). This transport mechanism is apparently responsible for as much as 50% of Na + -Cl − transport in CCD of the mouse while mineralocorticoids act. To play a role in modulating the rate of K + secretion, this mechanism of electroneutral NaCl reabsorption needs to be expressed in the late DCT and the connecting tubule.
An increase in the concentration of <SPAN role=presentation tabIndex=0 id=MathJax-Element-9-Frame class=MathJax style="POSITION: relative" data-mathml='HCO3−’>HCO−3HCO−3
HCO3−
HCO−3
HCO 3 −
and/or an alkaline luminal fluid pH seem to increase the amount of K + secreted in the CCD. It was suggested that this may be due to a decrease in the apparent permeability of Cl − and/or an increase in ROMK open probability in this nephron segment. It is also possible that the increase in luminal <SPAN role=presentation tabIndex=0 id=MathJax-Element-10-Frame class=MathJax style="POSITION: relative" data-mathml='HCO3−’>HCO−3HCO−3
HCO3−
HCO−3
HCO 3 −
concentration in CCD, inhibits the <SPAN role=presentation tabIndex=0 id=MathJax-Element-11-Frame class=MathJax style="POSITION: relative" data-mathml='Cl−/HCO3−’>Cl−/HCO−3Cl−/HCO−3
Cl−/HCO3−
Cl−/HCO−3
Cl − / HCO 3 −
exchanger (pendrin), and hence the NDCBE and the electroneutral NaCl reabsorption, perhaps leading to a higher rate of electrogenic reabsorption of Na + .
If the rate of reabsorption of Na + in CCD is not appreciably higher than the rate of reabsorption of Cl − , a large lumen-negative voltage cannot develop. The hyperkalemia in patients with the syndrome of “Familial Hyperkalemia with Hypertension” (also known as type II pseudohypoaldosteronism or Gordon’s syndrome) may be an example for this pathophysiology. Two factors are important to achieve this near-equal rate of ion transport in the CCD. First, low delivery of Na + and Cl − to the CCD occurs because the reabsorption of Na + and Cl − is augmented in the distal convoluted tubule because of increased activity of Na + -Cl − cotransporter (NCC). Second, effective arterial blood volume expansion suppresses the release of aldosterone; this leads to fewer numbers of open ENaC in the luminal membrane of principal cells in CCD. Hence the rate of reabsorption of Cl − in the CCD may match that of Na + .
ROMK channels are abundant and have a high open-probability on a K + -rich diet. Therefore ROMK channels do not seem to be rate-limiting for net secretion of K + unless the P K falls to the mid-3 mmol/L range in rats. Dietary K + intake is an important regulator of ROMK channels. Low K + intake decreases, whereas a high K + intake increases the number of ROMK channels in the luminal membrane of principal cells. The membrane trafficking of ROMK is modulated by phosphorylation of tyrosine in the channel protein, such that phosphorylation of tyrosine stimulates endocytosis and de-phosphorylation of tyrosine induces exocytosis. K + depletion increases the expression and activity of the protein tyrosine kinase and tyrosine phosphorylation of ROMK channels results in their endocytosis. Angiotensin II (ANG II ) inhibits ROMK activity in K + -restricted rats, but not in rats on a usual K + diet. The effect of ANG II may be mediated via activating NADPH oxidase II and the production of superoxide anions, which increases the expression of protein tyrosine kinase. The WNK ( With No Lysine ) kinases seem to play a role in modulating K + secretion. There are two isoforms of WNK1; a ubiquitous full-length WNK1 (WNK1-L) and a kidney-specific, short WNK1 lacking the kinase domain (WNK1-S). WNK1-L induces endocytosis of ROMK whereas WNK1-S inhibits this effect of WNK1-L. The ratio of WNK1-S to WNK1-L transcripts is reduced by K + restriction (more endocytosis of ROMK) and is increased by K + loading (less endocytosis of ROMK).
Flow Rate in the CCD
When vasopressin acts and aquaporin-2 water channels (AQP2) have been inserted in the luminal membrane of principal cells, the late distal nephron is permeable to water, the osmolality of fluid in the terminal CCD is equal to the plasma osmolality and hence is relatively fixed. Therefore, the flow rate in terminal CCD is determined by the number of osmoles in the luminal fluid.
The osmoles that are present in the lumen of the terminal portion of CCD are largely urea, NaCl, and K + with an accompanying anion. While there is little reabsorption or secretion of electrolytes in the nephron segments distal to terminal CCD, and hence the number of electrolyte osmoles in terminal CCD is close to their rate of excretion in the urine, this is not the case for urea. A quantitative analysis of the process of intra-renal urea recycling reveals that the amount of urea delivered to the early DCT is about two-fold larger than the quantity of urea that is excreted in the urine. In the following paragraphs, we shall illustrate that urea recycling aids the excretion of K + ; this process is especially important in subjects with disorders or those who are taking drugs that lead to a less lumen-negative voltage in the CCD.
Urea Recycling, Process and Quantitative Analysis
For simplicity, we shall begin with the absorption of urea in the inner medullary collecting duct (MCD) ( Figure 51.6 ). This absorption requires the presence of urea transporters in the luminal membrane of cells of the inner MCD (vasopressin phosphorylates and causes the insertion of urea transporters UT-A1 in the luminal membrane of cells in this nephron segment ), and a higher concentration of urea in the luminal fluid in the inner MCD than that in the interstitial fluid in the inner medulla. This later requirement is achieved because all the segments of the distal nephron that are upstream to the inner MCD, are likely to be impermeable to urea, but most of the water delivered to the early DCT is reabsorbed in the CCD and MCD owing to the insertion of AQP2 into the luminal membranes of principal cells. The concentration of urea rises to higher than 600 mmol/L in the luminal fluid that is delivered to the inner MCD ( Figure 51.6 ).

The bulk of the urea that is reabsorbed in the inner MCD leaves the inner medulla via the ascending vasa recta, because it has UT-A2 and, as a result, is permeable to urea. Once in the deep outer medulla, most urea will enter the luminal fluid of the descending thin limbs (DtL) of the loop of Henle of superficial nephrons that have their bends deep in the outer medulla, as they possess the UT-A2 and the concentration of urea is higher in the interstitial compartment than in the luminal fluid of the DtL, which allows for a high rate of delivery of urea to the early DCT.
To obtain a quantitative estimate of the amount of urea that is recycled, one needs an estimate of the amount of urea that is delivered to the early DCT and the amount of urea that is excreted in the urine over a given period of time. Since the amount of urea delivered to the early DCT cannot be measured in human subjects, it is essential to use data from experiments using the micropuncture technique in the early DCT in fed rats. In these studies, the amount of urea in the early DCT was 1.1 times the amount of urea that was filtered. Extrapolated to a human adult with a GFR of 180 L/day, and a plasma urea concentration of 5 mmol/L, a reasonable estimate of the daily delivery of urea to the early DCT is approximately 1000 mmol/day. Since subjects eating a typical Western diet excrete close to 400 mmol of urea per day, the amount of urea that recycles would be approximately 600 mmol per day.
Importance of Urea Recycling for the Excretion of K +
This process of urea recycling adds an extra 2 L to the flow rate in terminal CCD (600 mosmol divided by a luminal fluid osmolality that is equal to that in plasma or ~300 mOsm/L). A reasonable estimate of the number of liters of fluid that exit the terminal CCD per day is close to 5 L (1500 mosmol/day in the terminal CCD (1000 mosmol of urea/day and 500 mosmol/day of electrolytes) divided by 300 mosmol/L). This means, in quantitative terms that 40% of the flow rate in terminal CCD is the result of this process of recycling of urea.
Implications for the Patient with Hyperkalemia
Patients who develop chronic hyperkalemia have a renal defect that is characterized by an inability to generate a sufficiently large trans-epitheleal (TE) lumen-negative voltage in the CCD to secrete all their daily intake of K + while maintaining a normal P K . These patients, however, will achieve a steady state in which they will excrete all the K + they ingest and absorb that is not lost via non-renal routes. To understand how this steady state is achieved, we shall consider how a K + load of 70 mmol/day is handled in normal subjects and a patient with an electrical defect that leads to a diminished ability to raise the concentration of K + in the lumen of terminal CCD ([K + ] CCD ) ( Figure 51.7 ).

Normal subjects have a much larger capacity to secrete K + in their CCD compared to the usual daily intake of K + ; they excrete most of their daily intake over a relatively short period of time close to noon. This likely reflects an increase in the lumen negative voltage in CCD owing to a larger delivery of NaHCO 3 to the CCD at the time of the alkaline tide in urine pH. If a normal subject has a P K of 4 mmol/L and a TE voltage in the CCD of -61 mvolts, the [K + ] in luminal fluid in CCD would be 10 times the P K or 40 mmol/L. If this voltage could be maintained while 1-L of fluid exits the CCD, 40 mmol of the 70 mmol of K + would be excreted in this time period (approximately five hours). For the next 19-hours, if 4-L of fluid would exit the terminal CCD with a concentration of K + of only close to 8 mmol/L, the remaining 30 mmol of K + can be excreted to achieve K + balance.
Let us now consider a patient who has a large defect that leads to a lumen negative TE voltage in the CCD of only −29.1 mvolts ( Table 51.1 and Figure 51.7 ). At this TE voltage, the K CCD /P K can rise to a value of only three. At a P K of 4.7 mmol/L, the [K + ] CCD will be close to 14 mmol/L; hence at the usual flow rate in terminal CCD of 5-L/day, this patient could excrete 70 mmol of K + daily and maintain a normal P K . The point to emphasize is that this precise balance is dependant on intra-renal urea recycling and thereby maintaining the daily flow rate in the terminal CCD of 5-L. Therefore, if this patient were to be put on a protein restricted diet (decrease the number of osmoles of urea in the terminal CCD), a smaller number of liters of fluid will exit the CCD per day and hyperkalemia may develop. To put this in a quantitative perspective, if the number of liters exiting the CCD per day were 4-L instead of 5-L, and if the K CCD /P K were three, while this patient continued to consume 70 mmol of K + /day, in steady state, the P K will need to rise to 5.8 mmol/L to maintain K + balance and only if this maximum rate of K + secretion is maintained throughout the 24 hours period. To further emphasize this point, consider a patient who may have markedly impaired urea recycling due to a disease that involves the inner medulla (perhaps an example may be a patient with sickle cell disease). This patient may have a flow rate in the terminal CCD that is only 3-L/day rather than 5-L/day. If such a patient has a disease that leads to a reduced ability to generate a high lumen negative TE voltage in the CCD, a more severe degree of hyperkalemia will develop unless K + intake is markedly reduced. In quantitative terms, if the TE voltage in the CCD is −29.1 mvolts, the [K] CCD will be three-fold higher than P K . Therefore, if the volume of filtrate that exits the terminal CCD is only 3-L/day, the P K would have to be close to 8 mmol/L to excrete 70 mmol of K + /day.
TE Voltage(mvolts) | [K] CCD /P K | P K (mmol/L) | [K] CCD (mmol/L) | Flow Rate CCD (L/day) | K Excretion (mmol/day) |
---|---|---|---|---|---|
−29.1 | 3 | 4.7 | 14.4 | 5 | 70 |
−29.1 | 3 | 5.8 | 18 | 4 | 70 |
−29.1 | 3 | 7.8 | 24 | 3 | 70 |
−18.4 | 2 | 7.2 | 10 | 5 | 70 |
Integration of the Response to Dietary K + Intake: A Paleolithic Perspective
Virtually all our major control mechanisms developed in Paleolithic times; the important ones provided an advantage for survival. There have been no pressures in modern times that have enough control strength to over-ride these control mechanisms. The diet of our most ancient ancestors consisted mainly of fruit and berries; a diet that provides sugar, K + and organic anions, but little NaCl. In addition, this intake of K + was episodic, but large at times. Therefore, there was a need to have a mechanism in place to rapidly shift K + into the liver before it reaches the heart to avoid the risk of cardiac arrhythmia. Furthermore, the renal response needed to be orchestrated to switch from a NaCl conservation mode to a K + excretion mode. It is important in this regard to note that aldosterone can be a NaCl retaining or a kaliuretic hormone, in what has been dubbed as the “aldosterone paradox”. In more detail, when aldosterone acts, it causes the insertion of a larger number of open ENaC units in the luminal membrane of principal cells in CCD, which permits both NaCl retention or K + secretion. Thus, there must be another signal to select one of these possible effects. Of note, the secretagogue for aldosterone is ANG II when its release is in response to a low effective arterial blood volume. On the other hand, a higher P K directly stimulates the release of aldosterone, however, ANG II in this setting, will be suppressed.
Shift of K + into Hepatocytes
While it is well known that there is a rise in the P L-lactate in portal venous blood after absorption of dietary glucose, the physiological importance of this high P L-lactate was not evident when viewed solely in metabolic terms. We suggested that a possible function of this high portal P L-lactate is to prevent hyperkalaemia in hepatic venous blood following the absorption of K + from the diet. The process begins by increasing the rate of aerobic glycolysis in enterocytes; as a result of performing more work by intestinal cells and thereby the generation of more ADP, the other substrate (beside glucose) for glycolysis. This extra metabolic work occurs because the sodium linked glucose transporter (SLGT) in this location is SLGT-1. Hence, when 1 mmol of glucose is absorbed, 2 mmol of Na + must be absorbed; therefore more ATP will be converted to ADP to absorb a given quantity of glucose. Should glycolysis occur at a faster rate than that of pyruvate oxidation, L-lactate will released into the portal vein. In the liver, the uptake of L-lactic acid on the M-CT could lead to a rise the concentration of H + in the sub-membrane region of hepatocytes and hence activation of NHE-1. This electroneutral entry of Na + into hepatocytes and its subsequent exit via Na + /K + -ATPase in an electrogenic fashion, will lead to a higher negative intracellular voltage and hence the retention of K + in hepatocytes. This mechanism requires the presence of insulin, which is released in response to the dietary sugar load with the ingestion of fruit and berries. Perhaps, because of its effect, to cause the translocation of more Na + /K + -ATPase units to the cell surface of hepatocytes, insulin is required for this effect of L-lactic acid to cause a shift of K + into hepatocytes.
Integration of the Renal Response to Dietary K + Intake
WNK Kinases
Available evidence indicates that this complex network of with-no-lysine kinases (WNKs); WNK4 and WNK1, via effects on NCC and ROMK normally function as a switch to change the aldosterone response of the kidney to either conserve Na + or excrete K + , depending on whether the release of aldosterone is induced by a change in dietary Na + or dietary K + intake.
WNK4 is thought to inhibit NCC activity by reducing its plasma membrane abundance, by diverting post-Golgi NCC to the lysosome for degradation. The expected kaliuresis owing to increased delivery of NaCl to CCD is prevented because WNK4 inhibits ROMK, an effect that involves clathrin-mediated endocytosis. SGK-1, when ANG II is suppressed as in conditions of high K + intake, phosphorylates WNK4 and reverses its inhibition of ROMK. ANG II has been shown to inhibit ROMK activity in K + -restricted rats, but not in rats on their usual dietary K + intake. The effect of ANG II may be mediated via activating NADPH oxidase II and the production of superoxide anions, which increases the expression of protein tyrosine kinase such as c-Src. Src family protein tyrosine kinase phosphorylates ROMK and results in their endocytosis. Therefore, ANG II release due to low Na + intake or a K + restricted diet inhibits ROMK directly via protein tyrosine kinase or indirectly via blocking SGK-1 mediated WNK4 phosphorylation, which restores WNK4 inhibition of ROMK.
ANG II signaling through the ANG II receptor phosphorylates and converts WNK4 from an inhibiting mode to an NCC-activating kinase form. The activating form of WNK4 induces a phosphorylation cascade, whereby WNK4 phosphorylates the intermediary kinases, STE 20/SPS 1-related proline, alanine-rich kinase (SPAK) and perhaps also the oxidative stress responsive protein type 1 (ORS1). Phosphorylated-SPAK/ORS1 in turn phosphorylates and activates NCC. Since the release of aldosterone in states of hypovolemia (or low salt intake) is mediated by ANG II , but not when the stimulus of the release of aldosterone is due to hyperkalemia, this may provide a mechanism to signal the need of actions of aldosterone as a NaCl retaining hormone. In this setting, the effect of ANG II to inhibit ROMK prevents kaliuresis in response to the release of aldosterone and SGK-1. Although SGK-1 phosphorylates WNK4, this does not mimic the stimulatory effects of ANG II . The dual requirement for both SGK-1 and ANG II signaling to switch WNK4 from being an inhibitor to an activator of NCC would provide a mechanism to integrate the effects of high aldosterone and ANG II for appropriate activation of NCC in hypovolemia. It would also explain why high aldosterone and SGK-1 activation induced by a high-K diet does not stimulate NCC transport, in which case ANG II is not elevated.
In response to a large intake of K + , in the absence of ANG II , WNK4 will be in its NCC inhibiting mode and hence more NaCl will be delivered downstream. Aldosterone, acting via SGK-1, increases the number of open ENaC subunits in the luminal membrane of principal cells in CCD and hence the electrogenic reabsorption of Na + . Nevertheless, for this result in kaliuresis, ROMK channels in an open configuration must be present in the luminal membrane of principal cells. When ANG II is suppressed, SGK-1 mediated phosphorylation of WNK4 reverses WNK4 effect to inhibit ROMK.
As mentioned above, alternative promoter usage of the WNK1 gene produces a kidney-specific short form of WNK1, called WNK1-S, and a more ubiquitous long form, called WNK1-L. WNK1-L inhibits ROMK by inducing endocytosis of the channel protein; WNK1-S isoform inhibits this effect of WNK1-L. The relative abundance of the WNK1 isoforms is regulated by dietary K + . The ratio of WNK1-S to WNK1-L transcripts is reduced by K + restriction (greater endocytosis of ROMK) and increased by K + loading (reduced endocytosis of ROMK). WNK1-L up-regulates NCC by blocking the inhibitory form of WNK4 or directly via phosphorylation of SPAK/ORS1. WNK1-L has also been reported to activate SGK-1 through PI3 kinase. WNK1-S, on the other hand, antagonizes the effects of WNK1-L and therefore indirectly inhibits NCC.
Modulation by the Delivery of HCO 3 − to the CCD
An increased delivery of <SPAN role=presentation tabIndex=0 id=MathJax-Element-12-Frame class=MathJax style="POSITION: relative" data-mathml='HCO3−’>HCO−3HCO−3
HCO3−
HCO−3
HCO 3 −
and/or a rise in the pH of luminal fluid in CCD may provide a signal to augment the secretion of K + . The effect may be due to a decrease in the electroneutral component of Na + reabsorption in the CCD, which would increase the magnitude of the luminal negative voltage, if accompanied by an increase in electrogenic Na + reabsorption. Alternatively, or in addition, luminal alkalinization may increase the number of open ROMK in the luminal membrane of principal cells in the CCD.
In Paleolithic times, the major source of dietary K + was from fruit and berries. The anions ingested along with this K + were a family of organic anions that can be metabolized to neutral end products plus <SPAN role=presentation tabIndex=0 id=MathJax-Element-13-Frame class=MathJax style="POSITION: relative" data-mathml='HCO3−’>HCO−3HCO−3
HCO3−
HCO−3
HCO 3 −
. In addition, a higher P K is associated with alkalinization in the cells of the proximal convoluted tubule (PCT), which leads to inhibition of the reabsorption of <SPAN role=presentation tabIndex=0 id=MathJax-Element-14-Frame class=MathJax style="POSITION: relative" data-mathml='HCO3−’>HCO−3HCO−3
HCO3−
HCO−3
HCO 3 −
. The end result is the delivery of more <SPAN role=presentation tabIndex=0 id=MathJax-Element-15-Frame class=MathJax style="POSITION: relative" data-mathml='HCO3−’>HCO−3HCO−3
HCO3−
HCO−3
HCO 3 −
to the CCD, which promotes aldosterone to exert its kaliuretic effect. On the other hand, ANG II , which mediates the release of aldosterone in response to decreased effective circulating volume, is a potent activator of Na + /H + exchanger-3 (NHE-3) and the reabsorption of <SPAN role=presentation tabIndex=0 id=MathJax-Element-16-Frame class=MathJax style="POSITION: relative" data-mathml='HCO3−’>HCO−3HCO−3
HCO3−
HCO−3
HCO 3 −
in the cells of the PCT. Therefore, there will be diminished delivery of <SPAN role=presentation tabIndex=0 id=MathJax-Element-17-Frame class=MathJax style="POSITION: relative" data-mathml='HCO3−’>HCO−3HCO−3
HCO3−
HCO−3
HCO 3 −
to the CCD, which will allow for more electroneutral rather than electrogenic reabsorption of Na + in this nephron segment and hence more NaCl retention and less kaliuresis.
Medullary Recycling of K +
The Paleolithic diet provided little NaCl. Nevertheless, there is a need to deliver one mmol of Na + to the CCD to secrete one mmol of K + . Therefore, there must be a mechanism to increase the delivery of Na + to the CCD when there is a need to excrete a large K + load. Notwithstanding, it is also essential to minimize the excretion of Na + and Cl − to stay in salt balance.
To increase distal delivery of Na + , the kidney must make an investment. By this we mean that, the kidney must reabsorb some of the KHCO 3 delivered to the MCD to increase the medullary interstitial concentration of K + . While it may seem counter-productive to have active reabsorption of K + , when a high rate of excretion is needed, this process in fact increases the rate of excretion of K + by increasing the distal delivery of Na + . In more detail, a rise in the medullary interstitial concentration of K + will lead to depolarization of cells of the medullary thick ascending limb of the loop of Henle. This will diminish the exit of Cl − from these cells, as this is an electrogenic process, which carries a negative charge out of the cell. The subsequent increase in intracellular Cl − concentration inhibits the reabsorption on Na and Cl in the medullary thick ascending limb of the loop of Henle and hence increases their delivery to the distal nephron. This reabsorption of KHCO 3 could be achieved via the K + /H + -ATPase, which is likely to be present in the luminal membrane of cells in the MCD because of a prior state of depletion of K + before the large intake of K + occurred. As the rise in P K is sustained, there will be less K + /H + -ATPase units in the luminal membrane of MCD cells. Hence, the medullary interstitial concentration of K + will fall and a large natriuresis is avoided.
Intra-Renal Urea Recycling
The second source of dietary K + is from ingestion of animal organs (e.g., muscle and liver, which are rich in RNA). The principal anions that accompany this K + load are organic phosphates (which are ultimately metabolized to inorganic monovalent phosphate <SPAN role=presentation tabIndex=0 id=MathJax-Element-18-Frame class=MathJax style="POSITION: relative" data-mathml='(H2PO4−)’>(?2PO−4)(H2PO−4)
(H2PO4−)
(H2PO−4)
( H 2 PO 4 − )
) or sulfate anions. The key point here, is that unlike <SPAN role=presentation tabIndex=0 id=MathJax-Element-19-Frame class=MathJax style="POSITION: relative" data-mathml='HCO3−,H2PO4−’>HCO−3,?2PO−4HCO−3,H2PO−4
HCO3−,H2PO4−
HCO−3,H2PO−4
HCO 3 − , H 2 PO 4 −
and sulfate anions do not augment the secretion of K + unless the concentration of Cl − in luminal fluid in the CCD is very low. There is, however, a symbiotic relationship with another constituent in the diet, protein, because when its constituent amino acids are oxidized, urea is produced. The process of urea recycling aids the excretion of K + because it increases the number of liters of fluid that exit the terminal CCD. Owing to the process of urea recycling, as calculated above, the flow rate in terminal CCD is increased by about 2-L per day.
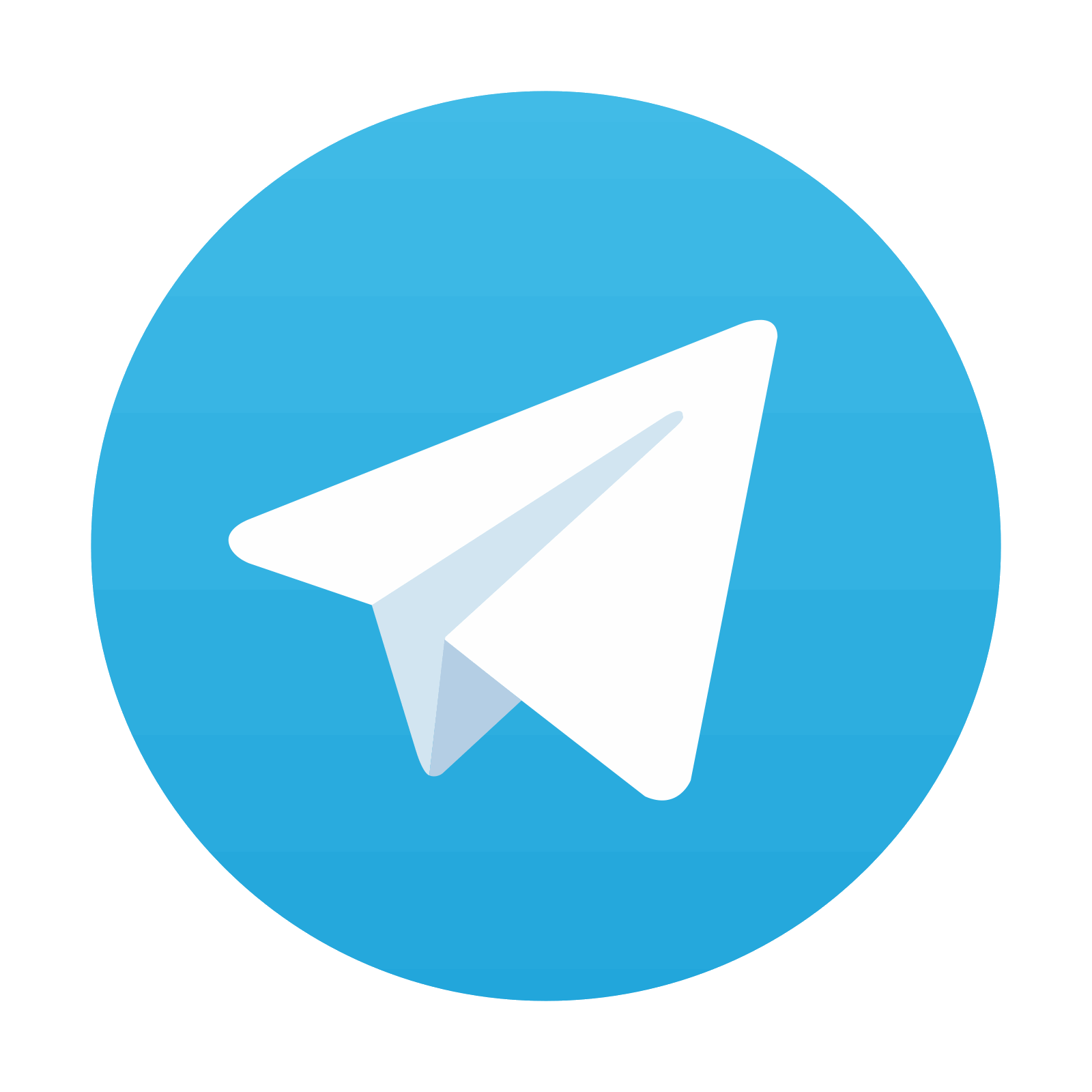
Stay updated, free articles. Join our Telegram channel
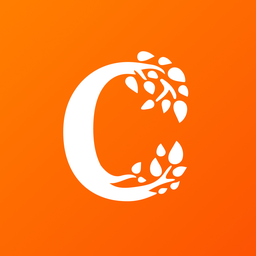
Full access? Get Clinical Tree
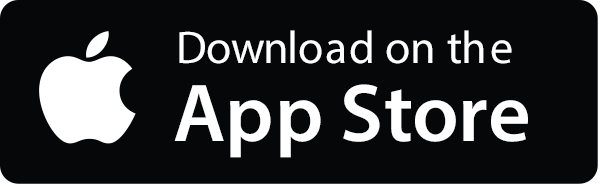
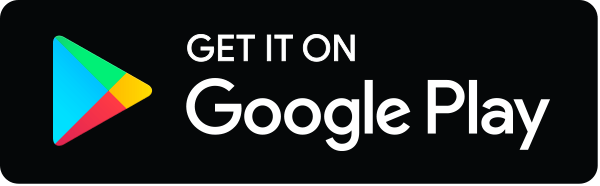