This review will trace the general mechanisms of renal cell injury before focusing on selected specific nephrotoxins that demonstrate the key principles of injury. Potentially, all of the cellular events involved in injury leave molecular footprints, which can be used as biomarkers in urine or plasma. Event specific biomarkers may allow mapping the time course of injury and the recruitment of secondary injury pathways. We will review the development and use of biomarkers in the detection of nephrotoxic injury. The selected nephrotoxins represent those agents where the key pathways of injury have been extensively investigated.
Keywords
nephrotoxicity, acute kidney injury, cellular injury, apoptosis, biomarkers, cyclosporine, aminoglycosides, cisplatin, acetaminophen, lithium
Introduction
Nephrotoxins produce acute or chronic kidney failure or functional injury, usually as a result of specific injury to tubular epithelial cells. While these toxins usually produce localized and specific injury to target cells in the kidney by specific mechanisms, many of the final common pathways of cytotoxic injury are shared with ischemia-reperfusion injury. As the latter have been studied more extensively, these are included as part of this review. The common cellular pathways involved lead to cellular adaptation, sublethal or lethal injury, and pathways for regeneration and repair. These events are not confined to the target cells but also involve other cells in the kidney, which may not necessarily reveal apparent injury but which invoke a stress response and/or inflammatory and vascular responses. The role of direct and indirect injury in mediating the phenotypic response to injury is illustrated schematically in Figure 85.1 . The parallel response in the cells that are not directly targeted, such as cytokine or growth factor release or release of inflammatory mediators and recruitment of further inflammation together with the response of target cells, are factors that determine the final outcome of toxic injury. The accompanying vascular response may well be pivotal. Thus, the pathophysiology of acute kidney injury includes a complex interplay among specific cellular injury, inflammation, and altered renal hemodynamics. This review will trace the general mechanisms of renal cell injury before focusing on selected specific nephrotoxins that demonstrate these principles of injury. Potentially, all of the cellular events involved in injury leave molecular footprints, which can be used as biomarkers in urine or plasma, Although such biomarkers have been used largely for diagnostic and predicitive purposes, it is through these biomarkers that we may be able to map such events non-invasively. Event specific biomarkers may allow mapping the time course of injury and the recruitment of secondary injury pathways. We will review the development and use of biomarkers in the detection of nephrotoxic injury.

Localization of Injury
Cellular Susceptibility: General Principles
Renal susceptibility to toxic injury emanates from a combination of nonspecific and specific factors. The major nonspecific factor is high blood flow with the kidney receiving 20–25% of the cardiac output, thus ensuring high delivery rates of circulating toxins. The general principles governing the susceptibility of a particular nephron segment to nephrotoxic or ischemic injury include segmental transport functions, metabolic activity, metabolic reserve, filtered load, and local blood and substrate supply including oxygen. The concentration of a drug or its metabolites will contribute to toxicity, and this will be modified by local tubular reabsorption, secretion, and metabolism, as well as by the local reabsorption of water. Intracellular activating mechanisms and organelle distribution will contribute further to toxicity. Because of the heterogeneity of these factors along the nephron, it is not surprising that the pattern of injury differs among nephrotoxins. Toxin delivery to a target site is a sine qua non for toxicity. Protein binding of the potential nephrotoxin will dictate whether the molecule is freely filtered at the glomerulus, dictating early exposure to the luminal aspect of proximal tubular cells or, if highly protein bound, to basolateral transport systems anywhere along the nephron. Thus, there is good correlation between the morphological and functional attributes of each nephron segment and the potential development of nephrotoxicity, especially in the proximal tubule where the greater proportion of specific drug toxicity occurs. Survival after toxicant exposure is also affected by the cycle status of the cell and the extent to which activation of pro- versus anti-apoptotic pathways and pro- versus anti-inflammatory pathways occurs; these factors collectively represent the “stress response” of the cells and are affected by the metabolic state of the cell. The anti-apoptotic and -inflammatory factors may be considered as a type of functional cytoprotective reserve (akin to antioxidant status in the face of oxidant stress), which might account for the differential susceptibility of particular tubular cells.
Renal Blood Flow and Cell Susceptibility
Heterogeneity of renal blood flow (RBF) defines the vascular limits of substrate and toxin delivery. The outer cortex receives 80% of the total RBF, the inner cortex and outer medulla only 15%, the inner medulla 5%, and the papillary region 2%. Renal oxygenation is modified by diffusional shunting of oxygen from arterial to venous vessels running in close approximation. This is most apparent in the vasa recta, so that oxygen tension also falls off sharply at the corticomedullary junction, thereby producing the characteristic low oxygen tensions found in the renal medulla averaging approximately 10 mm Hg. However, preglomerular shunting of oxygen also contributes and reduces cortical oxygenation to below renal vein values. This arrangement is essential for maintenance of the medullary interstitial osmotic gradient generated by the countercurrent multiplier system that allows modulation of water reabsorption for the generation of concentrated or dilute urine. In a teleological sense, the arrangement also provides a selection advantage by creating a zone of borderline oxygenation in the kidney that may be ideal for sensing hypoxia by erythropoietin-producing interstitial cells in cortex. The disadvantage is that any significant reduction in RBF—for example, in shock—can easily lead to hypoxic damage, at least in outer medullary tissues, including “medullary rays” situated in the cortex and containing the S3 proximal tubule and TAL segments arising from glomeruli in the outer and mid-cortical layers. Although both proximal tubular S3 cells and medullary thick ascending limb cells are exposed to a similar risk of hypoxia, only proximal tubular cells undergo early cell death following equivalent hypoxic insults in vivo . This suggests that there are differences in susceptibility to injury between these segments, which may reflect differences in segmental cytoprotective mechanisms as discussed below.
Regulation of regional blood flow may be critical to localization of hypoxic and toxic injury. Autoregulation of cortical blood flow is highly efficient, whereas the extent of autoregulation of medullary blood flow remains controversial. Some studies indicate that medullary blood flow is not efficiently autoregulated, especially during volume expansion. Other studies show efficient autoregulation of medullary blood flow under other conditions. Such disparate observations may arise from different methods of estimating medullary blood flow. Modulation of cortical and medullary blood flow may be critical to the actions of several nephrotoxins, such as iodinated contrast and nonsteroidal anti-inflammatory drugs. Heterogeneity of nitric oxide synthase (NOS) distribution within the kidney influences these regional differences in renal perfusion, and contributes to cytotoxic injury. All three isoforms of NOS are present in the kidney. NO from eNOS is involved in regulation and maintenance of blood flow, and NO synthesized by nNOS in the macula densa is important in the modulation of tubuloglomerular feedback (TGF) and regulation of glomerular filtration rate (GFR). iNOS is present in the tubules of normal rat kidney, predominantly in the medullary thick ascending limb, but its involvement in regulation of kidney function under normal physiological conditions is unclear. Quantitative studies of NOS activity and NO production suggest that the renal medulla is the principal site for basal NO synthesis in the kidney, suggesting an important role of NO in regulation of medullary circulation.
There is increasing interest in the role of the endothelium in ischemia-reperfusion injury, which may provide some insights into the effects of nephrotoxic injury on the renal vasculature. Endothelial dysfunction and vasoconstrictor hypersensitivity are well-recognized sequelae of ischemic acute renal failure (ARF). After ischemia-reperfusion injury loss of GFR arises from a combination of a reduced transcapillary hydraulic pressure gradient (ΔP) during delayed graft function and back leak of the glomerular filtrate presumed secondary to both epithelial injury and tubular obstruction. The decrease in ΔP may arise from two mechanisms. The first is a reduction in perfusion pressure secondary to an increase in afferent arteriolar tone as a result of some combination of vasoconstrictor hypersensitivity, endothelial dysfunction impairing the generation of vasodilators, and increased TGF. The afferent arteriole provides the highest contribution to renal vascular resistance and is the key modulatory site for renal autoregulation. The second mechanism is a rise in intratubular pressure arising from actual or functional tubular obstruction. Recent studies confirming the continuing presence of TGF activity after ischemia-reperfusion injury support the postulated contribution from enhanced TGF secondary to reduced sodium and water reabsorption. Structural obstruction of the nephron is recognized in ischemic ARF, and functional (high-flow) obstruction causing intratubular pressure increases sufficient to stop filtration have been observed in toxic ARF after p-aminophenol. Endothelial cell dehiscence and microvascular obstruction may also contribute a significant component of this reduction in renal blood flow, as recent studies have observed not only endothelial cell dehiscence, microvascular aggregation, and obstruction, but even reversal of flow. These observations coupled with evidence of endothelial ICAM1 adhesion molecule upregulation and leukocyte adhesion in the vasa recta explain the outer medullary vascular congestion observed consistently after ischemic injury, usually described as the “no-reflow” phenomenon.
Iodinated contrast agents may be injurious through modulation of regional blood flow. One of the two principal explanations for genesis of acute renal failure by contrast is induction of vasoconstriction, either directly by high osmolar-contrast agents or by release of endothelin or adenosine or both. Medullary blood flow may be critical, since the potential for regional hypoxia described above predisposes to ischemic injury when both nitric oxide and vasodilator prostaglandin synthesis are blocked. Iodinated contrast reduces NO synthesis in primary cultures of renal artery smooth muscle cells. Injection of radiocontrast results in an immediate decrease in renal blood flow that is counteracted by an increase in renal prostaglandin formation. When prostaglandin synthesis is inhibited by cyclooxygenase inhibitors, prolonged endothelin-mediated renal vasoconstriction is observed. Endothelin antagonists have not been successful in preventing contrast nephropathy. However, the only clinical trial using endothelin antagonists utilized a nonselective endothelin antagonist that promoted both an increase in circulating endothelin of longer duration than the duration of antagonism and antagonism of ET-B receptors, which would have suppressed NO production. Subsequent experimental studies have demonstrated protection by ET-A selective antagonists, but it is uncertain whether this protection was hemodynamically mediated.
Modulation of NO also contributes to calcineurin-inhibitor and cisplatin toxicity. Acute dose-dependent vasoconstriction of the renal microcirculation by cyclosporine and tacrolimus is NOS dependent and reversed by supplementation with l-arginine. Chronic cyclosporine involves downregulation of eNOS, which can be reversed by administration of l-arginine or pravastatin. Inhibition of NOS (by 2-amino-4-methylpyridine aggravates experimental cisplatin-induced nephrotoxicity with exaggeration of both histological and metabolic features of cisplatin toxicity including reduction in glutathione -peroxidase activity and elevation of platinum accumulation, but prevents the typical reduction in GSH and increase in malondialdehyde.
Both vasodilator and vasoconstrictor prostaglandins generated by COX from arachidonic acid are critical in the regulation of vascular tone and sodium and water homeostasis in the kidney. Endogenous prostaglandins have been found to modulate the regulatory status of the perfused kidney and there is growing evidence to indicate that COX-2 is involved in the modulation of afferent arteriolar autoregulatory responses. The interplay between endogenous vasodilators and vasoconstrictors—for instance, prostaglandins and endothelin—and the increase in vasoconstrictor production when vasodilators are stimulated or vice versa, highlights the important homeostatic effects of local autoregulation. This is critical in determining regional blood flow and toxicity, but can make interpretation difficult.
Cellular Targets
Cellular Targets: General
Significant exposure of the kidney to toxicants typically results in ARF. The primary target for most nephrotoxin and ischemic injury are epithelial cells of the proximal tubule. Other renal target cells include endothelial cells—for example, in response to Shiga toxin-2 or ischemia. Endothelial cells can also be modified by cytotoxins to sensitize them to other forms of injury. For example, incubation of endothelial cells with calcineurin inhibitors has been shown to increase subsequent adhesion by dendritic cells. Vascular smooth muscle cells can be indirect or direct targets. For instance, impaired vasorelaxation through impaired NO formation characterizes cyclosporine, contrast, and myoglobin toxicity as described. Severe ischemia may injure smooth muscle cells directly. Podocyte injury is critical in the development of several forms of glomerular disease, and is induced experimentally by the nephrotoxin puromycin. Tubular interstitial cells may be targeted by drugs with resultant tubulointerstitial nephritis, glomerular cells to cause proteinuria, and distal tubular cells to cause functional injury manifested as inhibition of transport (e.g., lithium and demeclocycline cause diabetes insipidus, and cotrimoxazole causes hyperkalemia) or structural damage (e.g., with fibrosis induced by lithium or other drugs). Secondary parenchymal injury may result from activation of inflammatory chemokines resulting in an inflammatory response with leukocyte activation and infiltration. This is a critical component of ischemia-reperfusion injury, but has been inadequately explored in nephrotoxic injury.
Proximal Tubular Characteristics
Most nephrotoxic and ischemic injury involves proximal tubular cells. Proximal tubule dysfunction is primarily responsible for activation of several of the main pathophysiological mechanisms, which leads to loss of GFR in acute renal failure as discussed earlier. The S3 segment of the proximal tubule and the medullary thick ascending limb (MTAL) of the loop of Henle traverse the outer stripe of the outer medulla and medullary rays in close proximity. These are regions of the kidney that are marginally oxygenated because of diffusional shunting of oxygen, and where blood flow is reduced in the setting of ischemic and some toxic injuries. Although both nephron segments have high transport activity and concomitant high metabolic activity, only proximal tubular cells are susceptible to injury causing cell death in most animal models. This greater susceptibility probably arises because proximal tubular cells rely principally on fatty acid oxidation for energy, and have low glycolytic capacity compared with MTAL cells under conditions of oxygen deprivation. Thus, the higher glycolytic capacity of MTAL cells allows continued generation of ATP in the setting of rapid ATP depletion resulting from impaired oxidative phosphorylation. There may also be greater cytoprotective reserve in MTAL cells in contrast to proximal tubule cells against early apoptotic injury through early and differential upregulation of anti-apoptotic Bcl-2 family members, and of the protective mitogen-activated kinases (MAP kinase) pathway regulated by extracellular signal kinase (ERK), as will be discussed further below. However, while ATP availability may make distal nephron cells less vulnerable to immediate cell death by necrosis, MTAL cells do undergo sublethal changes and produce various chemokines, cytokines, and growth factors that may have autocrine and/or paracrine effects on the injury and regeneration process of the kidney post-ischemia; MTAL cells may also undergo apoptotic cell death pathways under severe stress conditions.
Transport-Based Selectivity
In the S1 and S2 segments, the proximal tubular cells have well-developed brush borders, a well-developed phagolysosomal system, a large endocytic apparatus with numerous apical vesicles, and mitochondria associated with the basolateral membrane. Functionally, this region is associated with bulk isosmotic solute and fluid reabsorption coupled to the gradient generated by active sodium transport across the basolateral membrane by sodium ATPase and the tubular uptake and metabolism of protein by the endocytic and phagolysosomal systems. The final (S3) segment of the proximal tubule is located in the outer stripe of the outer medulla. The cells are characterized by less well-developed phagolysosomal and endocytic systems, but contain a much greater proportion of smooth endoplasmic reticulum (ER) and peroxisomes. S3 segment cells are the predominant location of the renal mixed-function oxidases, which contribute to both metabolic activation and toxicity of some compounds.
The greater diversity of transport functions in proximal tubule contributes to localization of injury because of preferential accumulation of many toxicants in this segment. For example, halogenated hydrocarbons are common environmental chemicals present in solvents and pesticides that produce toxicity and ARF after enzymatic conversion to reactive intermediates and cysteine S-conjugates, which are particularly injurious to the S3 segment of proximal tubule, since the S3 segment has the highest γ-glutamyl transpeptidase and cysteinylglycinase transport activity. Similarly, nephrotoxic heavy metals—including mercury, cadmium, gold, lead, nickel, and chromium—induce injury targeted to the proximal tubule as a result of active transport systems. For example, mercuric chloride conjugates with glutathione and cysteine in the liver and plasma, but accumulates in and is toxic to the S3 segment where the Hg-GSH conjugates are converted into Hg-cysteine conjugates by γ-glutamyl transpeptidase, and then transported intracellularly by the Na-dependent L-alanine, L-serine, and L-cysteine (ASC) systems, and the Na-independent L-system.
The relationship between transport proteins and protection is complex, as illustrated by cadmium-induced injury. Cadmium, a ubiquitous environmental toxin, is absorbed by inhalation or ingestion, and accumulates to produce proximal tubular injury. Cadmium induces the cysteinyl-rich metal-binding protein, metallothionein (MT), an important protective mechanism against many heavy metals. However, MT contributes to cadmium injury since MT-knockout mice are protected against Cd toxicity. Paradoxically, the MT complex facilitates uptake and accumulation by proximal tubular cells, with free cadmium and Cd-MT targeting different subsegments (S3 and S1/S2 segments, respectively) of the proximal tubule in vivo . Cd-MT complexes formed in the liver are freely filtered and taken up into proximal tubular cells by endocytosis. After endocytosed Cd-MT is degraded by lysosomes, free cadmium is released intracellularly and binds to subcellular targets to produce toxic injury characterized by proteinuria, phosphaturia, glucosuria, aminoaciduria, and polyuria. Some protection against cadmium is afforded by pretreatment with cyproterone acetate, which appears to increase transport out of the proximal tubular cells by upregulation of a ZnT-1 transporter gene that encodes for a membrane protein associated with zinc efflux.
Thus, transport systems, including endocytosis, are important in localizing toxicity. Gentamicin localizes to proximal tubule because of selective uptake. Megalin (also known as LRP-2), is a giant multiligand endocytic receptor, abundantly expressed in the apical membrane of proximal tubular epithelial cells, and is known to mediate uptake of β2-microglobulin. Megalin-knockout mice accumulate very little gentamicin compared with wildtype mice, suggesting that megalin-mediated endocytosis is the main mechanism of gentamicin accumulation in proximal tubules. Even more compelling evidence indicates that megalin mediates MT–heavy metal complexes including MT-Cd. Surface plasmon resonance and flow cytometry studies using overlapping MT peptides and recombinant MT fragments identified the hinge region of MT as a critical site for megalin binding, and suggest that disruption of the hinge motif (SCKKSCC) can inhibit proximal tubular MT uptake and eliminate much of the renal accumulation and toxicity of heavy metals such as cadmium, gold, copper, and also of cisplatinum, which is also transported bound to metallothionin.
Distal tubular cells are also targeted through selective transport mechanisms. Lithium toxicity localizes to the distal nephron because lithium ions enter the cells via the amiloride-sensitive Na channel to cause nephrogenic diabetes insipidus by markedly downregulating expressions of AQP2 and Na,K-ATPase. Through understanding this transport selective toxicity, it was shown that polyuria can be ameliorated by amiloride in patients receiving long-term lithium therapy. Similarly, the side effect of closure of luminal apical sodium channels in distal tubular cells—for example, by the antibiotics trimethoprim and pentamidine or by the diuretics triamterine and amiloride—is functional dose-dependent hyperkalemia, as blockade of Na transport reduces the transepithelial voltage and inhibits potassium secretion.
Cell Injury Pathways
Sublethal Injury
Nephrotoxic or ischemic injury not severe enough to cause cell death can nevertheless produce sublethal injury with significant pathophysiological consequences. Some nephrotoxins produce changes in solute reabsorption as highlighted above for lithium and trimethoprim. Similarly, cadmium and maleic acid toxicity can produce proximal renal tubular acidosis with aminoaciduria, and glycosuria, while amphotericin B can induce a distal renal tubular acidosis with hypomagnesemia and hypokalemia. The factors defining whether sublethal injury produces a higher risk of further injury leading ultimately to cell death or is protective through pre-conditioning (e.g., by upregulation of heme-oxygenase-1) remain of considerable interest in ischemia-reperfusion injury.
Sublethal injury associated with ATP depletion in proximal tubular cells results in impairment of epithelial permeability, loss of functional integrity of tight junctions (zona occludens), loss of cell polarity, and altered localization of cell adhesion molecules and polarized membrane proteins. Proximal tubular cell injury after acute chemical or ischemic insult is characterized by ATP depletion, mitochondrial and peroxisome dysfunction, impaired solute and ion transport, loss of cell polarity, and cytoskeletal dysfunction. Oxidative damage and ionic and pH changes also contribute to cell injury, but ischemic and chemically induced ATP depletion have been more extensively studied than other mediators of injury. The cellular and molecular lesions induced by ATP depletion are duration dependent and have been reviewed recently. The lesions include misfolding and aggregation of membrane and secreted proteins; disruption of the actin-based cytoskeleton; disturbances in apical–basolateral protein polarization; mislocalization and degradation of protein components of the intercellular junctions; upregulation of many genes, including molecular chaperones, growth factors and their receptors, and regenerative proteins; perturbation of integrin-mediated cell adhesion; and induction of cell death by apoptosis or necrosis.
Many of these changes occur very rapidly after the onset of ATP depletion, particularly changes involving the cytoskeleton. The apical microvillar region of proximal tubular cells is particularly sensitive because of the rapid rate of actin polymerization, so that severe but repairable damage occurs within five minutes of ischemia. These changes are not confined to tubular epithelial cells. Rather, renal ischemia rapidly produces characteristic and duration-dependent effects on the actin cytoskeleton of numerous renal cell types, including endothelial and smooth muscle cells, suggesting that the alterations are independent of cell type. Microvascular injury resulting from changes in the endothelial cell actin cytoskeleton—including cell swelling, altered cell–cell attachment and reduced endothelial cell–basement membrane attachment—may play a central role in reduced blood flow to affected microvascular beds secondary to altered vascular reactivity, in increased leukocyte adherence and extravasation, in altered coagulation after endothelial dysfunction, and in increased interstitial edema secondary to increased endothelial permeability. Injury to the vascular endothelium may also contribute to progressive loss of the microvasculature and perhaps via chronic hypoxia through glomerulosclerosis and tubulointerstitial fibrosis to progressive renal disease. Chronic hypoxia induced in part by angiotensin II–mediated vasoconstriction may similarly contribute to chronic cyclosporine nephrotoxicity.
Cell Death: Apoptosis and Necrosis
The responses of renal tubular cells to severe injury include cell death, de-differentiation of viable cells, proliferation, and re-differentiation and restitution of normal epithelium. Cell death pathways are initiated by the same stressors, that initiate adaptive responses, including toxins and ischemia. Where these stressors are of sufficient initial magnitude or there is failure of the adaptive response mechanisms to rescue the cell, cell death is initiated by either apoptosis or necrosis. These responses are intimately related to cell cycle events leading to recovery or cell death ( Figure 85.2 ). Heterogeneity of response in the kidney can result in concurrent apoptosis and necrosis within the same nephron segments following a single injurious influence. The distinction between these modes of death is important in defining therapeutic strategies. A clear distinction between modes of death follows the seminal study by Kerr, Wyllie, and Currie in which they coined the term “apoptosis,” and distinguished necrosis and apoptosis using morphological criteria. In necrosis, there is swelling of cell organelles including the mitochondrial matrix, chromatin flocculation, loss of plasma membrane integrity, and rupture of the cell contents into the extracellular space, which invokes an inflammatory response. The mitochondria undergo inner membrane swelling, cristeolysis, and disintegration. Polyribosomes disintegrate and disperse throughout the cytoplasm, and the cisterns of rough ER and Golgi apparatus are dilated and fragmented. In apoptosis, the nuclei undergo chromatin condensation, pyknosis, and karyorrhexis, with the chromatin forming crescents along the periphery of the nuclear membrane. The cells then shrink, lose microvillae and cell junctions, and fragment into a series of plasma membrane–bound condensed apoptotic bodies containing small masses of condensed cytoplasm, chromatin, and occasional organelles. Phosphatidylserine residues, normally located on the inner cell membrane surface, are exteriorized prior to cell fragmentation; these signal adjacent viable cells to phagocytose the apoptotic bodies without inducing an inflammatory response.

To emphasize the distinctions between the catastrophic nature of necrosis in contrast to the orderly, genetically driven process of apoptosis, these are sometimes referred to respectively as “accidental cell death” and “programmed cell death.” Despite these distinctions, there is considerable overlap between the cellular pathways involved in apoptosis and necrosis. For example, if a fragmented cell is not phagocytosed—for instance, in in vitro culture systems where macrophages are absent, and in vivo where phagocytosis is impaired, as in autoimmune disorders —the apoptotic bodies eventually swell and lyse, a process known as secondary necrosis. Consequently, the same insult may produce apoptosis or necrosis depending on severity. Studies in animal models which demonstrated that apoptosis is a “normal” feature of ischemia-reperfusion injury in vivo , have been confirmed in clinical biopsies of transplanted cadaveric kidneys, which are recognized as the best-defined model of human ischemia-reperfusion injury. These studies have stimulated interest in downregulating apoptosis as a means of ameliorating or preventing acute renal injury, a proposal that has now proven successful in experimental ischemia-reperfusion injury with the use of caspase inhibitors and erythropoietin.
Adaptive and cell regulatory pathways are initiated simultaneously with cell death pathways at the time of initial cell injury ( Fig. 85.2 ). Thus, injury and recovery are postulated to be part of the same response and involve engagement of the cell cycle. Recovery after injury requires an orderly progression through the cell cycle (reviewed by Price et al., ) which is regulated inter alia by the phosphorylation of substrates by interacting proteins called cyclins and cyclin-dependent kinases (cdk). Transition through so-called regulatory checkpoints G1 (gap-1) and S (DNA synthesis) and G2 and M (mitosis) cannot occur before each phase is completed. Natural inhibitors of cdk’s such as p21, the 21-kd protein that inhibits cyclin-dependent kinase 2 (cdk2) activity are increased naturally during G1 to prevent further cycle progression or by the p53 transcription factor after DNA damage or by p53-independent mechanisms after acute renal injury. In p21(-/-) mice, both cisplatin and ischemic injury produce more rapid and severe acute renal failure with morphologic damage including necrosis and apoptosis throughout the renal cortex, whereas damage is restricted to the S3 segment of the proximal tubule in wildtype p21(+/+) mice. In association with the increase in cell death there is an increase in cell cycle activity in p21(-/-) mice. The corollary to these changes is that increases in p21 expression are associated with suppression of apoptosis after injury, and in preventing p53-induced apoptosis in a variety of cancer types, while reduction of p21 may induce apoptosis in such transformed cells. These findings emphasize the importance of coordinated cell cycle control to recovery from acute renal injury with the final outcome dependent on both the severity of injury and the net balance of the cellular responses.
Lethal Injury: Apoptosis
Since apoptosis of renal tubular cells predictably accompanies necrosis and mechanisms of necrotic injury, such as ATP depletion and hypoxia, also induce apoptosis in cell culture models, inhibition of apoptosis may be therapeutic in ameliorating injury after ischemia-reperfusion injury including renal transplantation. Intensive investigation of the molecular pathways involved in apoptosis have demonstrated that aspects of necrosis are also actively mediated, and that cytotoxic injury also leads to apoptosis through similar or identical pathways affirming the concept of a continuum of cell injury and death.
Caspases are the molecular executioners of apoptosis. The molecular mechanisms of caspase-induced cell death were first elucidated in the nematode Caenorhabditis elegans . Caspases are a family of at least 14 highly specific cysteine proteases synthesized as proenzymes (procaspases). They all possess a catalytic cysteine in the active site, require a tetrapeptide recognition sequence with a C-terminus aspartate, cleave target proteins after an aspartate residue, and are hence stylized “c(asp)ases.” Procaspases are activated by proteolytic cleavage, usually at caspase substrate sites, which allows autolytic cleavage (e.g., adapter-facilitated self-cleavage by proximity) in a caspase cascade. Caspases are either pro-inflammatory (caspases 1, 4, 5, 11, 13) and involved in maturation of cytokines, such as both interleukin (IL)-1 and IL-18 in the case of caspase-1, or pro-apoptotic. The pro-apoptotic caspases can be divided into initiator caspases (caspases 2, 8, 9, 12) and effector caspases (caspases 3, 6, 7). Initiator caspases are activated by pro-apoptotic stimuli that induce adapter-facilitated self-cleavage. In turn, initiator caspases activate effector caspases that are responsible for cleavage of various physiological substrates including nuclear lamins, cytoskeletal proteins fodrin and gelsolin, actin-signaling protein, p21-activated kinase-2 (PAK2), and caspase-activated DNAase (CAD).
Target cleavage leads to the characteristic molecular and morphological patterns of apoptotic cell death. Lamins are intermediate filament proteins forming the nuclear lamina underlying the nuclear membrane and responsible for chromatin organization: caspase-induced cleavage leads to collapse and characteristic condensation of nuclear chromatin. Similarly, caspase-induced cleavage of fodrin and gelsolin leads to cell blebbing, while PAK2 cleavage leads to cytoplasmic and nuclear condensation, cellular detachment, and to phosphatidylserine externalization, which is critical for macrophage activation and phagocytosis. CAD activation, through proteolysis of ICAD (inhibitor of caspase-activated DNase), is one of the pathways of endonuclease activation. Endonuclease activation is the final common execution of both apoptotic and necrotic cell death pathways after ischemia-reperfusion injury and results in the generation of unrepairable strand breaks in double-stranded DNA. Random digestion of DNA in necrosis produces a smear pattern. Since internucleosomal DNA cleavage is nonrandom, producing DNA fragments that are multiples of ~180 base pairs and characteristic DNA laddering, these strand breaks have long been regarded as an indicator of apoptosis. However, other studies have shown that both apoptosis and necrosis can occur in the absence or in the presence of DNA fragmentation, so it is critical that apoptosis is defined by morphological as well as biochemical criteria. Caspase-independent (CAD-independent) DNA fragmentation occurs via endonuclease G, an endonuclease released from the mitochondrial intermembrane space and responsible mainly for single-strand fragmentation. Apoptosis-inducing factor also released from the mitochondrial intermembrane space and induces DNA fragmentation with high molecular weight fragments compared with the smaller fragments generated by caspase-induced deoxyribonuclease.
Caspases are activated by mitochondrial or ER-mediated intrinsic pathways or following activation of cell surface receptors, the extrinsic pathway. The extrinsic pathway is activated by triggering of cell death receptors, such as TNF and Fas, cell surface molecules that possess a common piece within their cytoplasmic tails called the death domain (DD). Binding of ligands such as FasL to these receptors (e.g., Fas) induces receptor trimerization and formation of a death receptor–ligand complex. Adapter molecules, such as Fas-associated death domain protein or TRADD in the case of TNF, possess both DD and the death sector domain (DED). The death receptor–ligand complex recruits FADD by binding to the DD. FADD in turn recruits procaspase-8 modules through DED interaction and forms the death-inducing signaling complex (DISC) that facilitates activation of caspase-8. Caspase-8 causes autocatalytic activation of further caspase-8 molecules through induced proximity. These translocate to the cytoplasm and directly activate downstream caspases, such as caspase-3, -6, and -7. Alternatively, caspase-8 catalyses the cleavage of cytoplasmic Bid, a death-promoting member of the Bcl-2 superfamily. Truncated Bid (tBid) translocates to the mitochondria, integrates into the outer membrane, and interacts with other Bcl-2 family members to promote release of cytochrome C and other pro-apoptotic factors from the mitochondria, thereby activating caspases of the mitochondrial-dependent pathway. Extrinsic pathway activation is regulated by Fas-associated death domain inhibitory proteins, like cytosolic FLIP (the FADD like IL-1β–converting enzyme inhibitory protein) and cowpox virus protein crm A, which inhibits caspase-8 and downstream caspases.
The mitochondria-dependent intrinsic pathway ( Figure 85.3 ) is responsible for most apoptosis, and leads to mitochondrial release of pro-apoptotic factors, including cytochrome C, AIF, and endonuclease G. Intrinsic pathway-activating signals include DNA damage, heat shock, radiation, oxidative stress, glucocorticoids, perturbations in redox balance, ceramide activation of tumor suppressor proteins such as p53, and oncogenes such as c-myc, growth factor withdrawal, and some nephrotoxins. During ischemia-reperfusion and after cisplatin, respiratory chain dysfunction impairs ATP generation. While severe ATP depletion causes necrosis, sustained milder degrees of ATP depletion can trigger apoptosis. One reason for the selective activation of apoptosis with milder degrees of ATP depletion appears to be the relatively greater reduction in the smaller intracellular pool of guanosine triphosphate (GTP), which is in tight equilibrium with ATP. While the GTP: GDP ratio regulates various signaling GTPases such as Ras and Rho, which are regulators of cell proliferation and apoptosis, GTP depletion also triggers an increase in p53, which translocates to the mitochondria and triggers mitochondria-dependent apoptosis. In addition to functional changes in mitochondrial energy generation, ischemia produces two structural abnormalities in mitochondria. First, it triggers collapse of the mitochondrial membrane potential (ΔΨμ) following opening of the mitochondrial transition pore in the inner mitochondrial membrane, producing high amplitude swelling and impairing ATP generation early in reflow. Second, it triggers leakage of cytochrome c leakage from the inner membrane space into the cytoplasm. Dislocation to the cytoplasm of cytochrome c, an electron shuttle protein, both impairs respiration and facilitates binding of deoxyadenosine triphosphate to the adapter protein apaf-1-cytochrome C-complex, inducing oligomerization to form the high-molecular-weight caspase-activating complex, the apoptosome. The apoptosome recruits procaspase-9, which autocatalytically activates and then proteolytically activates caspase-3, triggering execution of apoptosis.

The intrinsic mitochondrial signaling pathway is regulated by members of the Bcl-2 family. Mammals possess a large family of Bcl-2 proteins that includes pro-apoptotic as well as anti-apoptotic members. Anti-apoptotic members include the prototype Bcl-2, Bcl-xL, Bcl-w, and Mcl-1. Pro-apoptotic members include Bax, Bak, and Bok. A third class of molecules sharing homology with the Bcl-2 homology-3 (BH3) domain can activate pro-apoptotic members or inactivate antiapoptotic members and include Bin, Bid, Bad, and Bik. The ratio of anti- to pro-apoptotic molecules such as Bcl-2 to Bax is a measure of susceptibility to apoptosis via the intrinsic pathway, perhaps because pro- and anti-apoptotic members of the Bcl-2 family heterodimerize with each other. The precise mechanism by which mitochondrial intermembrane space proteins are released by the actions of Bax and Bak is uncertain. Translocation to the mitochondrion, oligomerization, and insertion of Bax into the outer mitochondrial membrane to form pores or channels, which allow release of intermembrane space proteins, has been proposed. Alternatively, Bax may mediate opening of the mitochondrial permeability transition pore, which then somehow modulates permeability of the outer mitochondrial membrane, perhaps after swelling and rupture. Nevertheless, although there is evidence for these alternatives (see Danial and Korsmeyer ), the precise mechanisms remain uncertain. It is clear, however, that anti-apoptotic Bcl-2 family members (such as Bcl-2 and Bcl-xL) block apoptosis-induced release of these intermembrane space proteins, perhaps by stabilizing and preventing opening of the mitochondrial permeability transition pore. Translocation of both pro- and anti-apoptotic Bcl-2 proteins to the mitochondria appears critical: for example, protection against apoptosis in distal tubular cells after oxidative injury was associated with translocation of Bcl-xL to mitochondria within the surviving distal cells, whereas proximal tubular cells showed reduced survival coupled with decreased expression of Bcl-xL, which did not translocate to the mitochondria. After ischemia-reperfusion in vivo , rat proximal tubules show a marked increase in Bax expression and apoptosis as well as an expected increase in necrosis. The relevance of these and similar experimental rat studies is clear from similar observations in human kidneys after cadaveric renal transplantation, where increases in apoptosis with ischemia time were associated with increased Bax expression and of cytoplasmic cytochrome C, confirming mitochondrial pathway involvement in the observed apoptosis.
Impairment of mitochondrial outer-membrane integrity allows numerous other proteins normally sequestered in the inner membrane space to enter the cytoplasm. These include caspase-independent death effectors such as the apoptosis-inducing factor (4), mitochondrial pools of co-caspases (such as caspases-2, -3, and -9), the second mitochondrial activator of caspases (Smac), the direct inhibitor of apoptosis proteins (IAPs) binding with low pI (Diablo), and serine protease HtrA2/Omi. AIF translocates to the nucleus triggering chromatin collapse and digestion of DNA as described earlier. IAPs regulate caspase activity by acting as pseudosubstrates. By binding IAPs that inhibit caspases-3, -7, and -9 directly, Smac/Diablo frees these caspases to promote cell death.
As shown in Fig. 85.3 , there are three potential levels of interaction between the extrinsic and intrinsic apoptotic pathways. Initial activation of caspase-8 via Fas can induce mitochondrial translocation of Bid with resultant cytochrome C release. Second, the transcription factor p53 activates pro-apoptotic Bcl-2 family members and also Fas-dependent apoptosis. Third, the extrinsic and intrinsic pathways converge at caspase-3 activation, the beginning of the execution phase.
Pro-inflammatory caspases are also involved in ischemic renal injury, reflecting our increasing awareness that even apparently purely ischemic renal injury involves significant inflammation. Caspase-1 (previously known as ICE, interleukin-converting enzyme) proteolytically cleaves the precursor forms of both pro-inflammatory cytokines IL-1β, and IL-18. These are increased after ischemia-reperfusion injury, although from a combination of knockout and protection studies, it appears that only the increase in IL-18 plays a significant but uncertain role. Cisplatin-induced apoptosis does not involve caspase-1 activation.
Nephrotoxic injury is associated with caspase activation and apoptosis. Cisplatin-induced apoptosis is associated with translocation of Bax to mitochondria, release of cytochrome c, and activation of caspase-9 and -3, while inhibition of these caspases provided partial protection confirming a role for mitochondrial pathway activation. Cisplatin also induces increased expression of TNF-α and Fas and with activation of caspase-8, suggesting a significant contribution to apoptosis from extrinsic pathway activation. Similarly, caspase-2 release from human fibroblast mitochondria is associated with cisplatin, etoposide, and UV light–induced apoptosis, which can be prevented by small interfering RNA (SiRNA)-mediated silencing of caspase-2 expression. Since p53 inhibition provides partial protection against cisplatin-induced apoptosis, a role for p53 in cisplatin-induced cell death seems likely.
The ER–specific caspase, caspase-12, appears to be involved in apoptosis following ER stress. The ER contains high concentrations of cytochrome P-450 enzymes, which can lead to generation of reactive oxygen metabolites. Microsomal CYP2E1 is the source of reactive oxygen species generation in cisplatin-induced apoptosis. This oxidative stress and mobilization of ER calcium stores precedes procaspase-12 activation, which then leads to apoptosis of LLC-PK1 cells, directly by bypassing caspase-9 and -3, but also partly mediated by caspase-3 activation, perhaps after oxidant injury to the mitochondria and resultant cytochrome C release.
Necrosis. While there have been considerable advances over the last decade in our understanding of apoptosis, our understanding of molecular pathways in necrosis has been more selective, beginning with the concept that necrosis is a largely “passive” process secondary to acute ATP depletion. Direct consequences of ATP depletion include impairment of ATP-dependent ion channel transport, disruption of ion gradients, actin cytoskeleton disruption, cell swelling, membrane blebbing, and cell disruption (see recent review by Padanilam ) . There is now evidence that both forms of cell death include actively mediated steps. Since both necrosis and apoptosis are frequently found together in the same tissue, distinguishing these death modalities may sometimes be difficult.
Classically ischemic, hypoxic or chemical ATP depletion leads to cell death associated with an increase in cell volume, so called “oncosis,” and was presumed to occur because of sodium and water influx and initial potassium ion efflux associated with increased plasma membrane permeability. This general pattern was confirmed using 23Na, 31P, and 87Rb magnetic resonance spectroscopy of intact kidneys. While the initial sodium influx is balanced by potassium efflux, increased intracellular sodium will further dissipate ATP by activating Na + ,K + -ATPase, and severe ATP depletion will lead to failure of pump-leak balance, further sodium influx, and cell swelling.
Further evidence indicates that whether a lethally injured mammalian cell undergoes necrosis or apoptosis may be determined by early activation of cell membrane ion channels. Most cells can regulate their volume by mechanisms called regulatory volume decrease or increase, after osmotic swelling or shrinkage. In contrast, a major hallmark of apoptosis is “normotonic” shrinkage of cells. Apoptotic volume decrease requires active K + and Cl− efflux. Necrotic volume increase is initiated by uptake of osmolytes, such as Na + , Cl−, and lactate after injury, hypoxia, ischemia, acidosis, or lactacidosis through membrane carriers and through stress-activated nonselective cation channels. Persistence of necrotic volume increase is caused by dysfunction of the regulatory volume decrease mechanism due to impairment of volume-sensitive Cl− channels under conditions of ATP deficiency or lactacidosis. The role of these ion fluxes in the death phenotype is highlighted by the observation that both lactacidosis-induced dysfunction of regulatory volume decrease and necrotic cell death are prevented by pretreatment of cells with the vacuolating cytotoxin-A (VacA) protein purified from Helicobacter pylori , which forms a lactacidosis-resistant anion channel. Similarly, blocking the increase in K + and Cl− conductances following stimulation of either extrinsic or intrinsic pro-apoptotic pathways prevented the biochemical and morphological changes of apoptotic cell death, whereas inhibiting apoptosis by broad-spectrum caspase inhibition did not prevent apoptotic volume decrease.
Stress-activated nonselective cation channels of the transient receptor potential, melastatin-like (TRP) family, such as melastatin-like TRPM2, also known as long transient receptor potential channel-2 (LTRPC2), are dormant in viable cells, but become activated and contribute to necrosis after binding ADP-ribose, or reactive oxygen or nitrogen species. LTRPC2 is permeable to both Ca 2+ and Na + after activation, and contributes to Na + and Ca 2+ overload. Since hypoxic and ischemic injury results in oxygen-derived free radical generation, such channels explain the earlier observation, that the rate of Na accumulation in the hypoxic kidney was reduced by free radical scavengers. Sodium overload coupled with severe ATP depletion leads to a necrotic outcome. Increased cytosolic calcium further increases ATP depletion by activating Ca 2+ -ATPase and by producing mitochondrial depolarization (see previous discussion). Even mild oxidative stress can increase cytosolic Ca 2+ by stimulating release of calcium from intracellular binding sites such as the ER. The role of these TRP channels in nephrotoxic injury is unknown, although the common features of oxidative and mitochondrial injury in cisplatin nephrotoxicity 1 suggest mechanisms likely to activate calcium influx and promote further injury.
Additional molecular events promoting necrosis triggered by ischemia include activation of proteases, phospholipases, endonucleases, poly(ADP-ribose)polymerase (PARP), iNOS, and the protein kinase (PK) family, including PKC and the mitogen-activated protein kinases (MAPK) and the generation of reactive oxygen species. The activated proteases include calpain and meprin (metallopeptidase from renal tissue), both of which mediate further injury. Phospholipases (PL) are activated by phospholipolysis following ischemia. PLA 2 has various isoforms that vary in their dependence on Ca 2+ for activation. Activation leads to membrane phospholipid breakdown, with potential loss of membrane integrity, generation of inflammatory mediators, and cytotoxicity from lysophospholipids and free fatty acids. Separate mention must be made of fatty acids, since β-oxidation of fatty acids is a major energy source in renal cortex. Renal peroxisomes and mitochondria utilize identical pathways for β-oxidation of fatty acids, except that peroxisomes, which are single-membrane–bound organelles, do not use carnitine for entry of fatty acids (in contrast to mitochondria where these are involved in transport into the mitochondrial inner matrix). Peroxisome proliferator–activated receptors (PPARs) are members of the nuclear hormone receptor superfamily of ligand-dependent transcription factors. PPARα is expressed predominantly in proximal tubule and medullary thick ascending limb in the kidney and agonists, including fatty acids, eicosanoids, and fibrate drugs, act as ligands and induce fatty acid oxidation. Peroxisomal as well as mitochondrial fatty acid oxidation are inhibited after ischemia-reperfusion and cisplatin injury to the kidney, while administration of PPARα ligands prior to injury was protective. Cisplatin attenuates PPARα signaling by reducing the expression of a tissue-specific coactivator of PPARα called PPARγ-coactivator-1 (PGC1) which is also a transcriptional coactivator for other transcription factors including nuclear respiratory factor, which are important in the regulation of oxidative metabolism, cellular respiration, and adaptive thermogenesis. Survival of renal tubular epithelial cells after cisplatin appears to depend on intact PGC-1/PPARα function. PPARα agonists appear to have therapeutic potential for cisplatin-induced ARF. Use of PPARα ligands prevented acute tubular necrosis by ameliorating cisplatin-induced inhibition of mitochondrial medium-chain, acyl-CoA dehydrogenase–mediated fatty acid oxidation and pyruvate dehydrogenase complex activity. PPARα ligand also protects against cisplatin-induced renal injury by reducing the expression and enzyme activity of proximal tubule endonuclease G.
The PKC family comprises serine-threonine kinases, with 12 isozymes activated by Ca 2+ , phosphatidyl serines, and diacylglycerol. Activation of PKCs leads to signal transduction via activation of G protein–coupled receptors, tyrosine kinase receptors, and nonreceptor tyrosine kinases. As a result of controlling 70% of the phosphorylating activity in the proximal tubule, PKC regulates numerous physiological functions of the renal epithelium including Na + ,K + -ATPase activity, gluconeogenesis, and transport of amino acids, glucose, sodium, potassium, chloride, water, organic anions, and cations. PKC isozymes also play a crucial role in the regulation of other major cellular functions, including proliferation and differentiation. After ischemia, the increased Ca 2+ and phospholipids hydrolysis products induce PKC isoenzymes and activated C-kinase receptor in kidney. PKC induction appears to be protective. Induced PKCα, -βII, and -ζ protect cultured renal cells from oxidant injury and subsequent necrosis. PKC is involved in ischemic preconditioning and heat shock–induced protection.
PKC isoenzymes directly influence mitochondrial function, and are therefore involved in recovery from both ischemic and apoptotic injury to renal cells. For example, while initiation of apoptosis by many agents is preceded by mitochondrial dysfunction and depolarization of the mitochondrial inner membrane, Nowak demonstrated that, in renal proximal tubular cells, cisplatin induces mitochondrial dysfunction associated with hyperpolarization of the mitochondrial membrane, and that these events are mediated by protein kinase C (PKC)-α and extracellular-regulated protein kinase (ERK)1/2. Nowak also demonstrated that PKC-α and ERK1/2 mediate activation of caspase-3 by acting downstream of cytochrome c release from mitochondria, and that ERK1/2 activation by cisplatin occurred through a PKC-α–independent pathway. Nowak subsequently observed that PKC inhibition decreases recovery of physiological function, recovery of PKC activity precedes the return of physiological functions after oxidant injury, and that activation promotes recovery of mitochondrial function and active Na + transport. Since oxidant injury–induced tert -butylhydroperoxide was associated with recovery of total PKC activity, while that associated with the nephrotoxic cysteine-S conjugate, S-(1,2-dichlorovinyl)-L-cysteine (DCVC), was not, Nowak concluded that the repair of renal function is mediated through PKC-dependent mechanisms, and that cysteine conjugates inhibit renal repair, partly through inhibition of PKC signaling. Subsequently, Nowak observed that serine but not threonine phosphorylation of the beta-subunit of F 1 F 1 -ATPase was increased during late recovery following DCVC. Inhibition of PKC-α activation decreased this phosphorylation, confirming that PKC-α regulation of repair of mitochondrial function involves phosphorylation of the catalytic subunit of F 1 F 1 – ATPase.
Repair and regeneration of renal cells after injury is less well studied than the initiation of injury but is known to involve proliferation and migration as well as repair of injured proximal tubular cells, and thus involves growth factors, integrins, and extracellular matrix protein interactions. Growth factors administered pre- or post-ischemia–reperfusion injury ameliorate injury and enhance regeneration through their anti-apoptotic, proliferative, and pro-angiogenic actions. Insulin-like growth factor (IGF-1) and hepatocyte growth factor may also enhance GFR after injury by increasing NO production, increasing local blood flow and vasodilating renal microvessels. However, the utility of growth factors in ARF therapy remains uncertain.
MAP kinases are serine/threonine kinases ubiquitously expressed in mammalian cells and known to be modulated in response to ischemic and toxic stress. MAPK activation is important in determining whether cellular stress leads to proliferation, differentiation, or apoptosis. Three MAPK cascades have been identified, the ERK, JNK, and p38 pathways. Cellular stress, such as toxic injury, induces generation of growth factors and inflammatory cytokines. The ERK pathway is activated by growth factors, vasoactive peptides, and nephrotoxins, including aminoglycosides and cephaloridine via a cytoplasmic-activating protein, MEK1. After activation, ERK proteins translocate to the nucleus, and phosphorylate and activate several Ets-domain transcription factors including Elk-1, SAP1, Ets-1, and Ets-1. ERK activation is involved in cell proliferation and differentiation and appears to be protective in the preconditioned post-ischemic kidney and in distal tubule cells. The JNK (c-Jun N-terminal kinase) pathway, also designated as stress-activated protein kinases (SAPK), are activated by genotoxic and osmolar stress, mechanical stretch, shear stress, and the inflammatory cytokines TNFα and IL-1β. They are also activated by ischemia-reperfusion. The p38 pathway is activated by many of the same stimuli that activate JNK/SAPK, but is independently regulated and plays a significant role in inflammatory cytokine production. Activation of the JNK/SAPK and p38 pathways is antiproliferative and can lead to cell death. While the effect of toxins on JNK activation in the kidney is unknown, JNK activation and subsequent apoptosis of hair cells have been implicated in gentamicin ototoxicity. Activation of ERK in distal tubule cells relative to JNK may contribute to the differential survival of these cells in contrast to proximal tubular cells after ischemia-reperfusion injury and with the renal protection associated with preconditioning.
PARP is a nuclear enzyme that adds ADP-ribose to various proteins including PARP itself after activation by DNA strand breaks. PARP is involved in both DNA repair and gene transcription and acts to open up the condensed structure of chromatin. After injury, activation of PARP leads to consumption of its substrate β-NAD + . Resynthesis of β-NAD + consumes further ATP. Whether the cell proceeds to apoptotic or necrotic cell death varies, possibly depending on the degree of initial ATP depletion or on PARP cleavage. While fibroblasts from PARP(-/-) mice are protected from necrosis, TNF-α can still induce death by apoptosis. However, in fibrosarcoma cells, TNF-α can activate PARP and induce death by necrosis. It appears that NAD + depletion induced by PARP activation may act as a trigger for AIF translocation from mitochondria to cytoplasm and nucleus to induce chromatin fragmentation. Moderate oxidative stress activates PARP, but it is then inactivated by caspase cleavage, which prevents further ATP depletion and enhances apoptosis.
The inflammatory cascade is initiated by ATP depletion and exacerbated by reperfusion. Endothelial, epithelial, and infiltrating inflammatory cells all participate in and mediate the inflammatory process after ischemia-reperfusion. Endothelial cells express adhesion molecules including ICAM-1, VCAM, P and E selectins, and β2-integrins. ICAM-1 binds to LFA-1 (CD11α/CD18) and Mac-1 (CD11β/CD18) receptors on leucocytes producing recruitment and activation. Involvement of adhesion molecules has been confirmed by blockade of ICAM-1 and selectin. Adherent leukocytes release reactive oxygen species, proteases, and other enzymes, and, together with the activated endothelial cells, release inflammatory cytokines such as IL-1, IL-6, IL-8, IL18, TNF-α, chemokines, and MCP-1. The relative importance of neutrophils, macrophages, and T and B lymphocytes as critical leukocytes in postischemic inflammatory events remains unclear. However, inhibition of various inflammatory pathways, including the CD28-B-7 costimulatory pathway by selectin blockade, the melanocortin-stimulated inflammatory gene stimulatory pathway by α-melanocyte–stimulating hormone, α-MSH, IL18 cytokine depletion in neutrophil-depleted mice, and of multiple pathways including cell infiltration, ICAM-1 and iNOS upregulation, matrix molecule expression, MAP kinase ERK1/2 activation, and transcription factor activation by cerivastatin, all ameliorate ischemia-reperfusion injury, thus supporting a critical role for inflammation in both necrotic and apoptotic injury.
Overlaps Between Necrosis and Apoptosis
While this review has delineated distinct molecular pathways that are triggered by pro-necrotic or pro-apoptotic events, it is important to emphasize that there is considerable opportunity for intersection and overlap between these pathways after both ischemic and nephrotoxic injury. Mitochondrial and peroxisomal involvement in both pathways appears likely. PARP activation may be crucial as highlighted above. Inflammatory mediators are involved in necrosis, but the observation that inhibition of apoptosis can ameliorate both inflammation and necrosis, for example, by pan-caspase inhibition after ischemia-reperfusion, emphasizes that apoptosis is frequently if not always a normal component of injury previously regarded as pure necrosis. Recruitment of stress-activated pathways and cell cycle proteins may be critical in determining the final outcome after injury. These overlaps highlight new therapeutic opportunities that may be available in the treatment and prevention of nephrotoxic injury.
Biomarkers of Nephrotoxic Injury
While serum creatinine and urea (BUN) have been used routinely to detect and monitor AKI, these conventional biomarkers of renal function only increase beyond the normal range when nearly half of the functional reserve of the kidney has been compromised. Thus, they detect kidney injury only after it is far advanced. They are also slow to respond to change in renal function. Since extrapolations to GFR from serum creatinine require that creatinine is in steady state equilibrium, this requires three to five half lives before such extrapolations are valid. The half-life of creatinine is four hours when GFR is normal and this increases in proportion to any decline in GFR. Thus after the one third decline in GFR required to be diagnosed as having AKI by the corrected RIFLE criteria, creatinine will only reach a steady-state after 12 to 20 hours or longer if the decline is greater. The implication is that at least 12 and, more likely 24 to 48 hours, must elapse before a new creatinine value is a reliable index of changed renal function. This creates an unavoidable delay in diagnosis of and intervention in AKI. In nephrotoxic injury, these delays may be greater since accumulation of drug or injury or both may be needed to induce measurable changes in renal function. In addition, drug-induced kidney injury in nonclinical animal models is a major cause of elimination of compounds during drug development; these compounds are eliminated from the development process even when it is unclear whether the toxicity would translate to humans. More sensitive biomarkers are needed to enable more reliable diagnosis of nephrotoxin-induced AKI in both clinical and pre-clinical studies and to facilitate earlier intervention and monitoring.
A biomarker is any parameter that can be objectively measured and evaluated, which acts as an indicator of a normal or pathological process, or of a response to intervention. The identification of biomarkers of renal cellular injury along with the re-discovery and quantitation of the predictive relationship between enzymuria and renal injury have re-kindled widespread interest in the nephrology and diagnostic communities, since these new tools offer the possibility of a paradigm shift in diagnosis of AKI and open the door to early intervention. These biomarkers are present in urine, plasma and sometimes both. Since plasma or serum biomarkers have many potential sources, urinary biomarkers generally have higher renal specificity and are easily acquired in non-oliguric AKI. Consequently, urinary biomarkers have been the main focus of recent studies. The urinary biomarkers fall into at least five categories: filtered biomarkers where glomerular permeability has increased, filtered biomarkers where renal reabsorption is impaired as a result of tubular injury, pre-formed cellular biomarkers released following injury, upregulated biomarkers, and inflammatory biomarkers released into the urine following recruitment of inflammatory cells. An alternative way of classifying these is as as inflammatory, excreted tubular proteins and surrogate markers of tubular injury, with different mechanisms for increase and significance. At present, the most studied biomarkers are the tubular enzymes GGT, GST and N-acetyl-beta-glucosaminidase (NAG), and the novel induced biomarkers, kidney injury molecule-1, (KIM-1), interleukin 18 (IL-18) and neutrophil gelatinase associated lipocalin (NGAL). New biomarkers are appearing regularly in the literature, for example, Trefoil factor 3, Clusterin, Netrin-1 and Aprotinin. Albumin may have a specific role as a biomarker in AKI since proximal tubular receptor-mediated uptake may be impaired.
Because nephrotoxic potential is critical in the both safety and commercial decisions regarding the continuation or abandonment of the development of candidate drugs, tubular enzymuria, particularly NAG, GGT, alanine-aminopeptidase, lactate-dehydrogenase and alkaline phosphatase have been widely used by the pharmaceutical industry to assess nephrotoxicity during drug development. However this process has not been regulated until recently. The United States Food and Drug Administration (FDA) and the European Medicines Evaluation Agency (EMEA) have taken an active role together with the pharmaceutical industry in developing a process for context-dependent qualification of biomarkers to improve the drug development and regulatory review process. The FDA and the EMEA have also expanded the context in which qualification for biomarkers is determined, and an expedient biomarker-qualification process is currently in place. The Critical Path Institute (C-Path) created the Predictive Safety Testing Consortium (PSTC) as a neutral ground where scientists from 16 pharmaceutical companies and academia could share and test new methods that are more reliable predictors of human safety. The PSTC developed the legal framework needed to share the cost of qualification and to protect intellectual property associated with biomarker qualification and it submits the data to the FDA and EMEA to support the biomarker certification process. The first FDA and EMEA review of biomarker qualification data involved biomarkers of renal injury and qualified seven biomarkers as supplemental data for evaluation of nephrotoxicity in nonclinical safety studies. These new biomarkers qualified for specific contexts of use are the urinary concentrations of KIM-1, albumin, total protein, β2-microglobulin, cystatin C, clusterin, and trefoil factor-3. The PSTC submitted data supported claims that three of these urinary biomarkers—total protein, β2-microglobulin, and cystatin C concentrations—could outperform levels of BUN and serum creatinine in detecting and monitoring drug-induced glomerular injury, while four urinary biomarkers—levels of KIM-1, albumin, clusterin, and trefoil factor-3—could either outperform or add value to levels of BUN and serum creatinine in detecting and monitoring certain drug-induced tubular injuries. More recently, renal papillary antigen-1 RPA-1 was qualified as marker of site-specific injury in the collecting duct and clusterin was requalified as a voluntary biomarker for use in detecting drug-induced tubular injury, particularly when signs of regeneration are present. These biomarkers were qualified for use as adjuncts in detecting nephrotoxic injury on a voluntary basis in pre-clinical rat studies while other biomarkers remain “exploratory.” The qualification process and need for more data on nephrotoxins and also on reversibility has encouraged much further study, e.g.
From both drug development and safety viewpoints, better biomarkers could enable safer and easier-to-monitor therapies by reducing the margin between efficacy and safety needed for clinical and preclinical applications. Much of the current work involves comparing biomarker performance in preclinical or clinical contexts. The sensitivity, specificity and time course of biomarkers are critical factors in determining utility in a particular disease. The favored metric for assessing biomarker performance is currently the receiver operator characteristic (ROC) curve analysis. When sensitivity is graphed against (1-specificity), the area under the ROC curve (AUC) (± the confidence interval) defines performance. The AUC for a perfect biomarker is 1.0, for a non-performing biomarker it is 0.50; clinical utility requires a minimum AUC of 0.70, although, realistically, much higher values are needed for clinical utility. Comparison of individual predictors and multivariable models is usually undertaken following the method of DeLong. The prognostic benefit of adding a biomarker to a baseline clinical model is calculated from the net reclassification improvement (NRI) and integrated discrimination Improvement (IDI) indices.
Thus in the detection of histological injury in one study of rat kidney injury induced by ten different nephrotoxins, urinary total protein (ROC AUC 0.86), cystatin C (0.92) and β2-microglobulin (0.89) showed a better diagnostic performance than BUN (0.80) and SCr (0.53) for detecting glomerular injury, while urinary clusterin (ROC AUC 0.88), outperformed BUN (0.79) and SCr (0.73) for detecting proximal tubular injury. Similarly, urinary KIM-1, outperformed the traditional biomarkers SCr, BUN and urinary NAG for the diagnosis of proximal tubular damage, using both using both exclusion and inclusion analysis approaches. For all observed histopathology grades following eight different nephrotoxicants, KIM-1 had the highest AUCs (exclusion analysis, 0.91; inclusion analysis, 0.88), whether compared to SCr (exclusion analysis, 0.73; inclusion analysis, 0.72), BUN (exclusion analysis, 0.79; inclusion analysis, 0.75) or urinary NAG (exclusion analysis, 0.82; inclusion analysis, 0.76). A total of tubular nephrotoxicants, including gentamicin, cisplatin and cyclosporine, and ischaemia-reperfusion injury were examined in that study. Regardless of whether the kidney injury was induced by well-established kidney toxicants or ischemia, urinary KIM-1 outperformed the conventional markers, BUN, SCr and urinary NAG. In addition, the very high ROC-AUC values of 0.91 to 0.99 for urinary Kim-1 demonstrated that urinary KIM-1 measurements were sensitive, specific and accurate in diagnosing either drug-induced or ischaemic kidney injury of various histological types as well as regenerative basophilia when lesions were either subtle with little organ involvement, or very severe with disturbed renal function. KIM-1 was subsequently qualified by the FDA as an early diagnostic biomarker of drug-induced acute kidney tubular alterations in rat toxicology studies, for regulatory decision making as a biomarker used by sponsors on a voluntary basis to demonstrate that drug-induced acute kidney tubular alterations are monitorable in good laboratory practice rat studies (used to support the safe conduct of clinical trials). Urinary KIM-1 was also considered qualified for regulatory decision making as a clinical bridging biomarker for use by sponsors on a voluntary basis in phase 1 and 2 clinical trials for monitoring kidney safety when animal toxicology findings generated a concern for tubular injury.
Using the same approach, the comparative performance, temporal profiles and recovery after gentamicin injury have been published for several candidate urinary biomarkers for kidney injury, including KIM-1, NGAL, osteopontin, glutathione-s transferases, RPA-1, albumin and clusterin. During the evolution of injury, KIM-1, RPA-1, and clusterin best mirrored the histopathologic lesions, while during injury resolution, KIM-1, osteopontin, and BUN best reflected recovery. Based on histopathology, necrosis, or apoptosis scoring, KIM-1 was the best biomarker of overall renal injury. RPA-1 outperformed the other biomarkers using regeneration scores. Clinical studies of KIM-1 have also yielded promising results. FDA/EMEA qualification of KIM-1 as a biomarker for clinical applications will involve a systematic evaluation of diagnostic performance in well-controlled observational and/or interventional clinical protocols using both standard-of-care agents with known nephrotoxic properties and/or exploratory agents with renal safety concerns. As pointed out by the authors, “the opportunity to use the same translational marker, such as Kim-1, for both the preclinical and clinical setting facilitates clinical monitoring of toxicity that has been demonstrated at higher doses in preclinical development or in a single test species when human relevance is suspected. ”
An additional potential role of biomarkers reflects their unique role in specific metabolic pathways of cell injury. Detected in serum or urine, their presence should flag recruitment of these specific pathways. Since many recently discovered biomarkers were uncovered by genomic or proteomic strategies, understanding their role in injury can be viewed as linking empirical outcomes to mechanisms. Three examples of novel biomarkers reflecting different injury pathways in AKI are IL-18, KIM-1 and NGAL.
IL-18 is produced in the kidney predominantly by recruited inflammatory cells, probably macrophages but also by activated injured renal parenchymal cells and has a significant role in AKI. Mice deficient in Caspase-1 or IL-18 or wild-type mice treated by IL-18-binding protein are protected from AKI induced by IL-18 injection. KIM-1 is a transmembrane protein express highly expressed in de-differentiated proximal tubular cells in response to injury. It has multiple roles including a role as a phosphatidylserine receptor that confers a phagocytic phenotype on epithelial cells. This allows these cells to recognize apoptosis cell surface-specific epitopes and thereby enables injured tubular cells to contribute to the clearance of apoptotic cells after AKI. The simultaneous detection of urinary KIM-1 and IL-18 could be regarded as good evidence of significant proximal tubular cell injury leading to cell death and de-differentiation. KIM-1 was the best performing biomarker by histopathology score in models of nephrotoxic injury using cisplatin and gentamicin.
The most highly upregulated biomarker after ischemia-reperfusion injury is NGAL, an acute-phase protein initially found in activated neutrophils but also expressed by a wide range of tissues in response to injury. NGAL participates in host innate immune defence by binding bacterial siderophores to limit bacterial iron acquisition. NGAL forms a complex with iron binding siderophores, which is renoprotective in murine ischemia-reperfusion injury. In the kidney NGAL mRNA expression is only detectable in the distal tubule, including the thick ascending limb of Henle’s loop and collecting duct, but the protein is freely filtered and reabsorbed by megalin-mediated transport in the proximal tubule. After ischemia and after cisplatin-induced toxic injury NGAL is detected in a punctate pattern corresponding to endosomes and predominantly in proliferating cell nuclear antigen-positive proximal tubular cells. This suggests that proximal tubular NGAL accumulates after injury but that the primary source is the distal tubule belonging to the injured nephrons. Urinary NGAL was recognized immediately as an important biomarker of acute kidney injury, with presence in urine suggesting renal specificity despite the possibility of alternative systemic sources. However, it was confirmed only recently, using cross-transplants between NGAL knockout (NGAL −/− ) and wild-type C57BL/6 mice (NGAL +/+ ) followed by renal artery clamping, that urinary NGAL definitely originates from the kidney. While urinary NGAL was increased in mice after cisplatin, it inexplicably did not appear to increase in some studies in the rat. Interestingly, urinary NGAL had a perfect score (ROC-AUC 1.0) after PAN, a nephrotoxicant that causes marked proteinuria and glomerular morphological changes in rats, similar to those seen in human minimal change nephropathy. Given the potential for albumin and protein to compete with NGAL (and other low molecular weight protein biomarkers) for megalin-mediated transport, the explanation for increased urinary NGAL following PAN may simply be that the proximal reabsorption of NGAL is inhibited. Urinary NGAL has not (yet) been qualified as a nephrotoxicity biomarker, although there is little doubt that both urinary and plasma NGAL have already proven to be a useful as a clinical biomarker of AKI, at least after ischemic injury where it clearly outperforms serum creatinine and blood urea nitrogen. Recent clinical studies have demonstrated that in high risk patients screened with urine or serum NGAL and serum creatinine, that creatinine negative but NGAL positive patients were at higher risk of both dialysis and had higher mortality that creatinine negative NGAL negative patients. This also highlights the described delay inherent in using creatinine to make a diagnosis of AKI and suggests that injury biomarkers should be included in the definition of AKI.
In addition to illustrating potential as markers of nephrotoxicity in drug development or in clinical studies, these three biomarkers illustrate the potential for injury biomarkers to provide useful information about timing, location and mechanisms of renal injury in disease. Since NGAL and KIM-1 are produced in different parts of the nephron, the performance of these biomarkers in urine samples should be different as these studies highlight. Recent clinical studies suggest that other factors also modify biomarker performance, including duration of injury and baseline renal function. Further refinements may allow biomarkers to differentiate the specific drug causing renal injury when the patient has been exposed to several potential nephrotoxins. For example urinary levels of regenerating islet-derived protein III beta and gelsolin were increased following gentamicin but not by cisplatin.
Large volumes of data are now needed. Well-designed preclinical and clinical studies that allow biomarker profiles to be followed after different types of AKI, including combinations of as well as individual nephrotoxins and in the presence of various comorbidities that may themselves contribute to biomarker generation or modify biomarker performance.
To illustrate the concepts of drug-induced nephrotoxicity, a number of important drugs with well-defined pathways of injury are discussed in depth.
Cyclosporin A
Since the introduction of cyclosporin A into clinical transplantation in 1978 it perhaps more than any other agent, has expanded our understanding of the mechanisms involved in drug-induced nephrotoxicity. Cyclosporin has been shown to modify many different aspects of cellular function, from hormone-receptor activation, second messenger pathways, transcription and translation of mRNA, alterations in protein synthesis and formation, changes in cellular metabolism, cellular repair mechanisms and cell death, the expression of various cytokines and growth factors, and changes in renal haemodynamics, all of which contribute to the final expression of nephrotoxicity.
Cyclosporin-induced renal functional impairment occurs in all forms of solid organ transplantation, bone marrow transplantation as well as in autoimmune disorders. The clinical manifestations of cyclosporin A nephrotoxicity can be classified into two groups, that are not necessarily exclusive. Cyclosporin A induces a marked increase in renal vascular resistance associated with a fall in renal blood flow and a decrease in glomerular filtration rate. These changes are often associated with the development of de novo hypertension. Cyclosporin A has been shown to modify renal tubular function with impaired distal tubular excretion of potassium and hydrogen ions consistent with a voltage dependent distal renal tubular acidosis, increased proximal tubular sodium reabsorption with decreased delivery of solute to the distal tubule, and impairment of urinary concentrating ability.
The clinical presentation of cyclosporin A nephrotoxicity may occur in three different time frames. Primary non-function of renal grafts, usually associated with kidneys that have been subjected to prolonged warm ischemia, donor and/or recipient hypotension, or concurrent drug nephrotoxicity. Cyclosporin-induced acute alterations in renal haemodynamics augment the damage already sustained by the kidney. The acute toxicity is not necessarily associated with an adverse outcome of long-term graft function although this is debated.
At a cellular level the changes in renal haemodynamics are mediated through a variety of hormone- receptor activated pathways, and subsequent intracellular signalling. Cyclosporin A has been shown to enhance the transmembrane Ca 2+ flux and increase intracellular Ca 2+ stores in response to agonist stimulation in vascular smooth muscle cells and mesangial cells which leads to enhanced contractility. Cyclosporin A has been demonstrated to inhibit activation of protein kinase C (an important component of the second messenger pathway) in vitro which also requires Ca 2+ for its activity. More recent studies have suggested that cyclosporin A inhibits phospholipase A2 directly. On this basis it is reasonable to assume that cyclosporin A modulates the hormone receptor activated pathway at several different points with subsequent impairment of cellular function. These alterations in transmembrane signal transduction induced by cyclosporin A have lead Skorecki and colleagues to propose that acute cyclosporin nephrotoxicity is a prototype for a renal membrane signalling disorder.
The acute administration of cyclosporin A causes a dose-dependent increase in renal vascular resistance and a reduction in RBF and GFR in experimental animal and human studies. These early changes are functional, with no structural damage evident and are reversible on withdrawl of the drug. In the renal microcirculation, similar reductions were observed.
Baxter et al. have shown that cyclosporin A activates the renin angiotensin system in vivo and in vitro ,. Similarly, cyclosporin A has been shown to stimulate and enhance the release of renin from juxtaglomerular cells in culture. Stimulation of mesangial cells by cyclosporin A alone does not modify the release of the vasodilatory prostaglandins or increase in the vasoconstricting prostaglandin thromboxane A 2 (TXA 2 ). However, cyclosporin A inhibits angiotensin II (AII) stimulated prostaglandin release from mesangial cells, as well as in vascular smooth muscle cells in culture. Exaggerated glomerular thromboxane synthesis has been suggested as a potential mechanism in producing the cyclosporin A induced reductions in GFR. Perico and colleagues have shown, in vivo , that glomerular synthesis and urinary excretion of thromboxane progressively increase with cyclosporin A therapy. Other studies have demonstrated that the increase in urinary thromboxane excretion is a consequence of intrarenal platelet and macrophage activation probably triggered by cyclosporin induced endothelial damage rather than arising from glomerular mesangial cells. The increased release of the vasoconstricting thromboxane from these nonrenal sources could potentate the reduction in glomerular filtration rate associated with cyclosporin A.
Alterations in nitric oxide (NO) and endothelin (ET) have been implicated in cyclosporin-mediated changes in vascular haemodynamics. Cyclosporin enhanced release of ET has been implicated as a significant mechanism contributing to cyclosporin-induced vasoconstriction. Cyclosporin has been demonstrated to inhibit NO production by inhibition of inducible nitric oxide synthetase (iNOS) activity, possibly by inhibition of transcription factors in mesangial and vascular smooth muscle cells A number of studies have demonstrated that NO blockade enhances acute cyclosporin nephrotoxicity.
Chan et al. examined endothelial–dependent and independent vascular reactivity in ex vivo studies using aortic rings from rats treated with cyclosporin (5 mg/kg/day) for 7–21 days. They failed to demonstrate impaired endothelial–dependent vasorelaxation but reported enhanced responsiveness to vasoconstricting agents. Therefore, it would seem probable that cyclosporin A induces a decrease in vaso-relaxant mediators (NO, PGI 2 , PGE 2 ) associated with an enhanced response to vasoconstricting agents (AII, ET) which would allow mesangial cell and vascular smooth muscle cell contraction to predominate. Subacute nephrotoxicity frequently occurs in the first six months post transplant, and it is associated with a slow rise in plasma creatinine or a persistently elevated plasma creatinine compared to azathioprine treated control patients with or without other signs of cyclosporin-induced alterations in renal function. A significant proportion of patients will have sufficient damage due to interstitial fibrosis that results in irreversible renal impairment. Renal histological changes include an arteriopathy with the development of constrictive intimal proliferation with mucoid thickening and arteriolar hyalinosis, and injury to the proximal tubule with foci of tubular dilatation, epithelial cell degeneration, fine reticulin deposition in the interstitium and giant mitochondria. In addition, a significant infiltration of macrophages into the interstitium, associated with an increase in interstitial and tubular cell proliferation has been demonstrated following chronic cyclosporin exposure. Activated infiltrating macrophages will amplify the inflammatory and profibrotic response by stimulating fibroblast proliferation and migration as well as increased collagen synthesis. Endothelial cell injury associated with the vascular changes may also be associated with a haemolytic-uremic like syndrome.
Prolonged administration of cyclosporin A, particularly at higher concentrations, has led to the development of chronic nephrotoxicity associated with hypertension, significantly elevated renal vascular resistance and deteriorating renal function that may progress to end stage renal failure. Histopathological changes associated with chronic cyclosporin nephrotoxicity are diffuse interstitial fibrosis or striped interstitial fibrosis with tubular atrophy, an obliterative arteriolopathy and ischaemic changes in some glomeruli.
Lesions characteristic of cyclosporin nephrotoxicity seen in human kidneys have been reproduced in sodium depleted rats treated with cyclosporin. Jackson et al. found that cyclosporin-induced focal areas of tubular atrophy and interstitial fibrosis in the outer cortex and an increase in cell proliferation, the majority of which were located in the interstitium and not the renal tubules. Chronic cyclosporin A administration (12.5 mg/kg/day for 10 weeks) to rats was shown to produce histological injury to the inner stripe of the outer medulla and medullary rays of the kidney similar to that seen in the striped interstitial fibrosis associated with chronic cyclosporin A administration in humans. It is significant that these changes were demonstrated in nephron segments particularly sensitive to limited oxygen availability. Thus hypoxia secondary to cyclosporin-induced vasoconstriction may further potentiate cellular injury. This may also play an important role in the subsequent development of interstitial fibrosis as discussed below in Figure 85.4 .

Cyclosporin A has a high lipid solubility and is extensively distributed to extravascular tissues, with the highest concentrations found in fat, liver, and kidney. Metabolism of cyclosporin A is via the cytochrome P-450 dependent mixed function oxidases. Experimentally, cyclosporin A has been shown to significantly inhibit hepatic mixed function oxidases by uncompetitive inhibition. The renal cortex contains similar cytochrome P-450 dependent mixed function oxidases located predominantly in the S3 segment of the proximal tubules which is the main site of histological injury produced by cyclosporin A. Walker et al. have demonstrated that cyclosporin A produces a significant uncompetitive inhibition of renal cortical NADPH cytochrome C reductase, an essential component of the mixed function oxidase enzyme complex.. The combined effect of lower enzyme activity, a greater degree of uncompetitive inhibition and lower enzyme concentrations acting as a rate limiting step, may in part explain the increased propensity for renal tubular damage as compared to hepatocellular damage despite similar tissue concentrations of cyclosporin A in the liver and kidney. Ischaemic damage to the kidney associated with transplantation, coupled with cyclosporin A induced reduction in renal blood flow, would alter oxygen availability and metabolic requirements in the S3 segment of the nephron. This reduced oxygen gradient would further limit the activity of the renal mixed function oxidases already compromised by the inhibitory effects of cyclosporin.
Cyclosporin A has been shown to reduce renal glutathione content and produce an increase in lipid peroxidation, in vitro , in renal cortical microsomes, mitochondria and membrane fractions. Cyclosporin A has been shown to generate superoxide through an as-yet-unclarified alteration in cytochrome P450 dependent mixed function oxidase. A reduced availability of glutathione could impair the ability of glutathione peroxidases to metabolise fatty acid peroxides generated by lipid peroxidation. The net effect could be an increase in oxidant injury to renal tubular cells exposed to a hypoxic environment. The hypoxic environment may also impair cellular mechanisms essential for maintaining cell integrity (see below).
Cyclosporin A has been demonstrated to alter cellular macromolecular synthesis in various cell lines in culture within two hours of exposure to cyclosporin, suggesting that cyclosporin might be altering the normal regulatory mechanisms that control the cellular enzyme systems. At a cellular level cyclosporin has also been shown to modify the intracellular assembly of the renal tubular Na + D-glucose cotransporter with subsequent intracellular accumulation of the transporter components. Similarly cyclosporin has been demonstrated to selectively reduce the gluconeogenic capacity of rat proximal tubules via a decrease in phosphoenolpyruvate carboxykinase (PEPCK) activity. This is due to a selective inhibition of renal PEPCK gene transcription and a corresponding reduction in renal PEPCK mRNA. Protein kinase C, a ubiquitous enzyme important in regulating a variety of intracellular functions, can be inhibited by cyclosporin A both in vivo , and in vitro . These results would suggest a selective inhibition of the activity of a transcription factor(s) required for the expression of specific genes in renal tubules may play a role in cyclosporin-induced nephrotoxicity. The net effect of direct inhibition of various intracellular enzymes or inhibition of gene transcription–translation controlling the synthesis of these enzymes, would be to impair the ability of renal cells to maintain their function and integrity in response to the toxic insult. Histological evidence of cellular damage either apoptosis or necrosis will only be apparent if the toxic injury exceeds the capacity of the cellular mechanisms to respond to the damage or alternatively if the cellular reparative mechanisms are modified by changes in intracellular regulation ( Fig. 85.4 ).
Buss et al. have demonstrated that exposure to cyclosporin directly interferes with renal microsomal protein translation or the regulation of translation. Subsequent studies have shown that cyclosporin binds to cyclophilin A (the intracellular cyclosporin binding protein), which is a microsomal peptidyl prolyl cis-trans isomerase, that facilitates protein folding and maturation. Inhibition of cyclophilin A results in an accumulation of unfolded protein which in turns contributes to endoplasmic reticulum stress that further contributes to cellular injury.
Within cells almost all soluble and membrane proteins of the cell are processed or degraded by the endoplasmic reticulum (ER). Proteins enter the ER in an unfolded state as linear polypeptides. In any situation in which an increased burden of unfolded proteins exceeds the ER’s ability to deal with them results in a state of ER stress. Pallet and colleagues have provided further evidence supporting the role of cyclophilin A inhibition and ER stress in contributing to tubular injury. If the ER’s capability to deal with the increased load of protein via resident enzymes and/or chaperone proteins is exceeded, this may then lead to cellular injury, transformation to a more profibrotic phenotype and cellular death via apoptosis.
Cyclosporin has been demonstrated to induce apoptosis in a number of models of cyclosporin toxicity via increased expression of pro-apoptotic mediators. The induction of apoptosis was associated with increased TGFβ synthesis which will further favor the development of interstitial fibrosis. Cyclosporin has been shown to upregulate Fas and Fas ligand expression, p53 expression, alter the ratio of Bax and Bcl-2, and upregulate ICE and caspase 3 activity. This favours the induction of apoptosis leading to tubular cell dropout and impaired ability to remodel after cyclosporin-induced injury. More recently Justo and colleagues have demonstrated that cyclosporin-induced apoptosis was associated with Bax translocation to the mitochondria and release of cytochrome c that was caspase-independent as well as a caspase-mediated alteration in mitochondrial membrane potential.
Activation of anti-apoptotic pathways offers a possible therapeutic option. Erythropoietin binding to its receptor activates a number of intracellular pathways including the phosphatidyl-inositol 3 phosphate kinase/Akt axis which is a key regulator of cell proliferation and survival. Pallet and colleagues have demonstrated the recombinant human erythropoietin protects against acute cyclosporine nephrotoxicity in part through Akt signaling and inhibition of caspase 3 activation. This would be consistent with other experimental studies that have demonstrated a protective role for EPO in acute kidney injury.
A number of studies have demonstrated a dissociation between cyclosporine–induced changes in GFR and progressive interstitial fibrosis. Angiotensin II has been shown to be an important renal growth factor independent of its vascular actions. Chronic exogenous AII exposure produces significant renal interstitial proliferation and fibrosis. In addition, renal type I interstitial fibroblasts contain a high density of AII receptors. Experimentally, blockade of the renin – angiotensin system with either AII receptor antagonists or angiotensin converting enzyme inhibitors prevented cyclosporin induced interstitial fibrosis without modification of renal functional parameters.
Angiotensin II has been shown to stimulate the release of TGFβ in vascular smooth muscle cells. Furthermore, interactions between TGFβ and PDGF determine the nature of the growth response of cells to AII in vitro . Both PDGF and TGFβ are potent fibrogenic growth factors that stimulate fibroblast proliferation, collagen synthesis and alterations in extracellular matrix synthesis. Enhanced renal expression of both PDGF and TGFβ during cyclosporin administration has been demonstrated both experimentally and clinically. Insulin-like growth factor 1 (IGF1) has also been implicated as a mediator of cyclosporin-induced renal tubular interstitial fibrosis.
More recently, the active non-hypercalcemic vitamin D analog, paricalcitriol has been shown to attenuate cyclosporin A induced renal tubular acidosis and fibrosis in an experimental rat model of nephrotoxicity. Park and colleagues propose that the renoprotective effects are due to down regulation of nuclear factor–kappa B (NF-κB) and nitric oxide signalling with subsequent down regulation of TGF β1 signalling pathways. However the role of paricalcitrol on other recognised pathways for CSA-induced nephrotoxicity also need to be explored.
The transmembrane efflux pump P-glycoprotein has been linked to the development of chronic cyclosporin nephrotoxicity. P-glycoprotein is associated with cyclosporin elimination by proximal tubular epithelial cells. Reduced renal expression of tubular P-glycoprotein has been shown to be related a greater degree of cyclosporin nephrotoxicity. Recently clinical studies have demonstrated that low levels of renal P-glycoprotein, in the donor kidney leads to decreased cyclosporin clearance and subsequent cyclosporin accumulation and toxicity.
The chronic interstitial fibrosis associated with cyclosporin therapy appears to be related to a complex interplay between cellular regulatory pathways controlling cell death or survival, various cytokines and growth factors whose expression will also be modified degree of cellular injury, as well as by the relative ischemia associated with cyclosporin-induced alterations in renal perfusion as outlined in Figure 85.5 .

Thus cyclosporin appears to affect many facets of renal function, from changes in whole-kidney function in vivo , to alterations at a subcellular level demonstrated in cell culture models. Despite the large number of studies investigating cyclosporin nephrotoxicity, there are still many unanswered questions as to how this unique agent produces the various changes that are manifested in the development of nephrotoxicity.
Cisplatin
Cisplatin is an antineoplastic agent which acts by inhibiting DNA synthesis. It has been used success fully in solid organ tumours, particularly in testicular germ cell tumours. The clinical use of cisplatin is limited by the development of nephrotoxicity which are manifest clinically as renal tubular defects in potassium, calcium, magnesium and hydrogen ion handling, inability to concentrate the urine and with more severe toxicity, changes in RBF and GFR. The extent of nephrotoxicity has been reduced significantly by the use of appropriate hydration schedules with normal saline.
The high renal concentrations of cisplatin, and subsequent development of nephrotoxicity, are a consequence of renal tubular cell handling predominantly via peritubular uptake and metabolism of cisplatin. Renal clearance of platinum is very efficient, with high clearances at low plasma levels of the drug, due to net tubular secretion in addition to filtration of platinum. Filtration of cisplatin does not appear to be a prerequisite for the initiation of nephrotoxicity; rather, the tubular uptake of cisplatin and metabolites including the basolateral transport of cisplatin and/or metabolites may be important in the generation of nephrotoxicity. The kidney specific organic cation transporter 2 (SLC22A) mediates the basolateral uptake of cisplatin and thus its subsequent toxicity. Cisplatin excretion from the cell is via the multidrug and toxin extrusion 1 transporter (MATE1), but the extent to which this may or may not influence intracellular concentrations of cisplatin is less clear.
The current concept of cisplatin nephrotoxicity is that cisplatin uptake by tubular epithelial cells activates complex intracellular signalling pathways including DNA damage response pathways, caspase activation (extrinsic, intrinsic and ER stress pathways), induction of free oxygen radicals along with inactivation of anti-oxidant systems, leading to cell apoptosis and necrosis. This is associated with an intense inflammatory response with TNFα a major mediator further exacerbating tubular cell injury ( Figure 85.6 ).

Morphological studies have shown that cisplatin induces focal damage to the proximal tubules, predominantly confined to the S3 segment located in the outer stripe of the outer medulla. The earliest changes of nucleolar segregation, microsomal dispersion and aggregation of smooth endoplasmic reticulum are evident within six hours of cisplatin administration. These early changes are consistent with apoptotic changes. Functional changes leading to depression of glomerular filtration rate (GFR) are not apparent until 48 hours post administration, by which stage there is focal loss of the tubular epithelial cells’ brush border, cellular swelling, and focal areas of necrosis. With established renal failure and maximal nephrotoxicity, there is widespread tubular necrosis, predominantly involving the S3 segment and to a lesser extent, the S1, and S2 segments of the proximal tubule.
It has been demonstrated that the cis isomer of the platinum complex is necessary for tumoricidal actions and is also responsible for the development of nephrotoxicity. Unlike other heavy metal-induced nephrotoxicity, the onset of cisplatin toxicity is delayed, with functional changes not readily apparent until 48–72 hours. This suggests that toxicity is not due directly to the effects of the platinum ion but rather to the biotransformation of cisplatin to various platinum metabolites and subsequent binding to intracellular components. The cisplatin metabolites, especially the hydrolysis product [Pt(NH 2 ) (OH 2 ) 2+ ], when infused into rats at equivalent concentrations to cisplatin, generate greater nephrotoxicity.
Within the kidney, cisplatin and metabolites are preferentially localised to the juxtamedullary cortical regions with a greater degree of injury seen in the S3 segments of the proximal tubules. Cellular concentrations are high in the nuclear and microsomal fractions, with quantitatively the greatest concentrations of cisplatin in the protein fraction of the cytosol associated with the sulfhydryl rich metallothioneins. Levi et al. demonstrated that following cisplatin administration in vivo there was a marked fall in protein bound sulfhydryl groups. The binding to sulfhydryl groups suggests that glutathione dependent transferases may be important in the modulation and/or mediation of cisplatin nephrotoxicity. It has been suggested that either an active platinum metabolite binds to the sulfhydryl group, or alternatively the platinum metabolite(s) inhibits oxidative metabolism, preventing regeneration of reducing equivalents necessary to maintain the reduced sulfhydryl groups. Exogenous reduced glutathione, at a high dose, has been shown to reduce cisplatin-induced nephrotoxicity, further supporting the role of binding to sulfhydryl groups as well as the generation of free oxygen radicals is important in mediating toxicity. Depletion of mitochondrial GSH has been shown to be an early and critical step in cisplatin-induced cell injury which results from oxidative stress to the mitochondria. Kruidering and colleagues using porcine proximal tubular cells, have demonstrated that cisplatin specifically affected mitochondrial function by inhibition of the enzymatic complexes I to IV of the respiratory chain with decreased intracellular ATP levels as a consequence. GSH reductase activity was also impaired leading to reduced intracellular GSH levels. Alterations in mitochondrial function will also lead to increased oxidative stress.
Thus the formation of reactive oxygen species may be one of the early signals that contribute to the activation of various intra-cellular signalling pathways leading to cellular injury. A number of experimental models ( in vitro and in vivo ) have demonstrated renoprotective effects for a number of different anti-oxidants including vitamin E, N-acetyl cysteine, and dimethylthiourea (DMTU).
Recent studies have demonstrated several pathways by which cisplatin-induced apoptosis occurs ( Figure 85.7 ) that include the extrinsic pathway mediated by death receptors such as Fas and TNFα, the intrinsic pathway via mitochondrial-dependent pathways and the endoplasmic reticulum (ER) stress pathway. In the extrinsic pathway, the binding of ligands to the major death receptors Fas and TNFα receptors leads to activation of caspase 8 and subsequent downstream pathways leading to apoptosis. It has been shown that cisplatin upregulates the expression of Fas and Fas ligand in both lymphocytes and proximal tubular epithelial cells. TNFα plays a major role in the inflammatory response associated with cisplatin injury, which may be the major route of subsequent injury rather than the activation of the extrinsic pathway.

Experimental evidence suggests that the intrinsic pathway via mitochondrial injury is the major pro-apoptotic pathway in cisplatin injury. Bax activation, mitochondrial release of cytochrome c and activation of caspase 9 are key steps in the pathway.
The third pathway is via endoplasmic reticulum stress with activation of caspase 12 and more recently a role for COX-2 and membrane bound prostaglandin E synthase 1 in mediating cisplatin nephrotoxicity has been demonstrated. In addition cisplatin may induce reactive oxygen species in microsomes via cytochrome P450 activation.
Renal cells mount a cytoprotective response to oxidative stress. In an hypoxic setting, up-regulation of hypoxic–inducible factor 1α (HIF-1α) plays an important protective role against injury. Cisplatin has been shown to suppress HIF-1α in cell culture models. Downstream mediators of HIF-1α actions include heme-oxygenase 1, vascular endothelial growth factor and erythropoietin, all of which have been demonstrated to provide a degree of protection against cisplatin-induced nephrotoxicity. Therefore HIF-1α is a potential target for protection against cisplatin nephrotoxicity.
DNA fragmentation has been observed in both apoptotic and necrotic pathways related to cisplatin injury, suggesting a common link at the level of cell death endonucleases. Basnakian and colleagues, using a deoxyribonuclease 1 (DNase 1) knock out mouse model, recently demonstrated that DNase 1 is important in mediating cisplatin-induced DNA fragmentation, tubular necrosis and possibly tubular necrosis. They demonstrated that the inactivation of DNase 1 was protective against cisplatin-induced DNA fragmentation. Another important step in the cellular response to DNA damage is activation of p53. A number of studies have demonstrated that p53 is rapidly phosphorylated and induced prior to tubular cell apoptosis. It is thought that activated p53 mediates its action through increased transcription of target genes. Studies have shown that p53 activation co-localises with apoptosis and nephrotoxicity can be ameliorated in p53 deficient mice. Interventions that reduce cisplatin-induced nephrotoxicity have been shown to reduce apoptosis and decrease p53 activation.
In cisplatin-induced nephrotoxicity, there is an intense inflammatory response to injury and experimental studies have demonstrated that TNFα is a key regulator of the inflammatory response. Inhibition of TNFα results in a marked amelioration of cisplatin injury. Of interest, is recent evidence that renal tubular cells contribute significantly to TNFα production during cisplatin nephrotoxicity. This in conjunction with the studies by Linkerman who demonstrated a close crosstalk between proximal tubular epithelial cells and distal tubular epithelial cell, suggest that the local production of TNFα by renal cells plays an important role in regulating cellular response to injury including apoptosis or necrosis pathways, referred to as fratricide.
Cisplatin nephrotoxicity appears to be dependent on renal tubular uptake and probable conversion to more toxic intermediates. Nephrotoxicity is probably secondary to alterations in cellular mitochondrial function, generation of reactive oxygen species and the balance between the activation of cell death pathways and cell survival signals and inflammation.
Acetaminophen Nephrotoxicity
Acetaminophen (paracetamol) is an antipyretic and analgesic agent that is freely available as a proprietary medicine and is the most widely used analgesic in the United States. Acetaminophen is the major metabolite of phenacetin, which has been used in compound analgesics. Both agents, either alone or in combination with other analgesics, have been implicated in the pathogenesis of analgesic nephropathy. In addition, Sandler et al. demonstrated a significant association between excessive acetaminophen intake and the subsequent development of chronic renal failure. The renal handling of phenacetin and acetaminophen exemplifies the heterogeneity of renal anatomical, biochemical, and physiological characteristics that influence the development of acute and chronic nephrotoxicity. Phenacetin is metabolised extensively to acetaminophen by the liver and gut. Only very low concentrations of the parent compound enter the systemic circulation. Phenacetin is filtered at the glomerulus and undergoes passive reabsorption along the nephron at a rate equivalent to water, related to the lipid solubility of the drug. Acetaminophen is moderately lipid soluble and it is filtered at the glomerulus with passive diffusion of the non-ionic form. Clearance is independent of plasma concentrations or tubular reabsorption. Reabsorption is not localised to a particular nephron segment. Acetaminophen clearance is related to the rate and the concentration gradient generated between intracellular/interstitial compartments and the tubular fluid. Under conditions of antidiuresis, acetaminophen increases in concentration in the inner medulla, conversely diuresis results in a diminution of the concentration gradient, with the concentration of acetaminophen being the same as that of the cortex. The clearance of phenacetin is increased by changes in urine flow rate directly proportional to the increase in urinary flow. The accumulation of acetaminophen in the medulla is important in the subsequent development of chronic nephrotoxicity. Renal metabolism of acetaminophen is essential in the development of nephrotoxicity and is linked to the proportional conversion of acetaminophen to its non toxic and toxic metabolites. The generation of non-toxic conjugated metabolites is potentially rate limited and increases in renal concentrations of acetaminophen will favour the formation of toxic metabolites. The distribution of the enzymes involved in the metabolism of acetaminophen is important in the development and localisation of the renal injury in acute and chronic nephrotoxicity. The predominant pathways for acetaminophen metabolism involve the NADPH + -dependent cytochrome P-450 mixed function oxidases located in the renal cortex and the NADPH + -independent prostaglandin H synthase (prostaglandin endoperoxidase synthetase system), consisting of a fatty acid cyclooxygenase and prostaglandin hydroperoxidase, localised predominantly in the inner medulla.
Acute Nephrotoxicity
This occurs clinically in the context of an acute overdose of acetaminophen but is not always associated with acute hepatic toxicity. The concentration of acetaminophen in the kidney is important in the development of acute nephrotoxicity. Acute toxicity is dependent on metabolic activation of acetaminophen to reactive metabolites. This has been shown to be a prerequisite for the development of acute tubular necrosis which is confined predominantly to the renal cortex. Following an acute overdose, there is insufficient GSH generated to bind all the metabolites generated by cytochrome P450 mixed function oxidases and intracellular reduced glutathione stores become depleted. Reactive metabolites bind covalently to intracellular constituents, as well as causing depletion of cellular GSH and lipid peroxidation, producing cell damage and eventual cell death. In the renal cortex, acetaminophen may be deacetylated to p-aminophenol, which is five to 10 times more nephrotoxic. Deacetylase activity is predominantly located in the renal cortex and is significantly more active than in the liver. Cytochrome P-450, prostaglandin H synthase, and possibly other peroxidases can convert acetaminophen and p-aminophenol into reactive intermediates through peroxidative reactions with the formation of semiquinoneimines [N-acetyl-p-benzoquinoneimine (NAPQI)], quinoneimines, or p-benzoquineimines (PQI). In the absence of GSH and other reducing equivalents, acetaminophen and p-aminophenol may undergo catalytic conversion mediated by mixed function oxidases, peroxidation by cytochrome P-450 or prostaglandin H synthase, as well as auto oxidation of p-aminophenol to reactive arylating intermediates. Cytochrome P-450 mediated peroxidative activation of both acetaminophen and p-aminophenol can continue to occur despite the depletion of cellular GSH and NADPH + . The arylation of renal macromolecules and subsequent cellular damage has been postulated as the initiating event in acetaminophen-induced acute nephrotoxicity. The toxicity of acetaminophen occurs only after the depletion of cellular GSH. GSH protects against toxicity induced by acetaminophen metabolites by several potential mechanisms. GSH may form a glutathione conjugate with NAPQI, which will then be excreted. GSH can react with NAPQI to reform acetaminophen. GSH may protect against lipid peroxidation due to further reactions of NAPQI. Recent evidence also suggests a possible nephrotoxic role of p-aminophenol glutathione S-conjugates and other hepatic metabolites. These S-conjugates after γ-glutamyl transpeptidase dependant cleavage are translocated into renal tubular epithelial cells where p-aminophenol derived S-conjugates accumulate as has been demonstrated for toxic S-conjugates from other xenobiotics.
GSH is a prerequisite for the activity of glutathione peroxidases, which protect the cell from peroxidative injury. Depletion of GSH by acetaminophen and p-aminophenol reactive intermediates would prevent glutathione peroxidase activity, increase lipid peroxidation, and enhance cytochrome P-450 mediated peroxidase activation of acetaminophen and p-aminophenol. Acute acetaminophen nephrotoxicity involves the metabolic activation by cytochrome P-450, mixed function oxidases, deacetylase, and prostaglandin H synthase with the generation of reactive intermediates. When the cellular protective mechanisms, predominantly GSH and GSH-related enzymes, cannot detoxify the reactive intermediates, tissue damage and subsequent acute tubular necrosis occur.
Chronic Nephrotoxicity
With chronic administration, acetaminophen achieves a higher concentration in the cells of the renal inner medulla compared to the renal cortex and plasma. This relatively higher concentration is important in the subsequent development of chronic nephrotoxicity. The prostaglandin H synthase enzyme complex, which appears to be the main mediator of chronic nephrotoxicity, is predominantly located in the inner medulla. The hydroperoxidase component reduces the hydroperoxy group of PGG 2 to the alcohol producing the endoperoxide PGH 2 , with the oxidation of a suitable electron donor. Acetaminophen has shown to act as a suitable substrate in this enzyme reaction. Mohandas et al. have demonstrated in vitro , using rabbit renal cortical and medullary microsomes, that the prostaglandin synthesis is enhanced by acetaminophen at low concentrations, comparable to those that would be achieved during chronic ingestion (up to O.5 mM), and inhibited at higher concentrations (as would be seen in acute overdose situations). More recently, similar results were demonstrated using human renal cortical, outer and inner medullary microsomes. The prostaglandin hydroperoxidase component of the enzyme complex converts acetaminophen to its reactive metabolite probably by a one electron oxidation reaction and hydrogen abstraction to form the phenoxy radical of acetaminophen, which then undergoes further oxidation to the more reactive intermediate NAPQI. This metabolite reacts rapidly with GSH with the regeneration of acetaminophen or the formation of a stable conjugate. The sharing of the conversion of PGG 2 to PGH 2 by both glutathione peroxidase and prostaglandin hydroperoxidase would influence the extent of co-oxidative activation of acetaminophen, since depletion of GSH by binding to NAPQI would decrease glutathione peroxidase participation in this reaction as opposed to prostaglandin hydroperoxidase which does not require GSH as a substrate. The increased prostaglandin hydroperoxidase activity would increase the co-oxidation of acetaminophen and formation of active metabolites. The cellular concentration of GSH is critical in preventing damage initiated by the reactive acetaminophen metabolites. The metabolite NAPQI may form a conjugate or is reduced back to acetaminophen with the production of oxidised glutathione (GSSG). GSSG is reduced by glutathione reductase requiring NADPH + . Thus, depletion of cellular GSH may occur due to decreased availability of NADPH + , low activity of glutathione reductase in the renal medulla, or the movement of excess GSSG out of the cell, in addition to conjugation with metabolites. In addition, extracellular GSSG is not readily available to the renal inner medulla cells due to very low levels of gamma glutamyl transpeptidases on the cell membranes as opposed to high enzyme concentrations in renal cells in the cortex.
More recently, it has been demonstrated that acetaminophen may cause direct toxicity to renal inner medullary cells. Cai and colleagues using passage 1 rat inner medullary collecting duct (p1rIMCD) cells maintained in a osmotic environment that represents the osmolality of the inner medulla in vivo , demonstrated a toxic effect of acetaminophen and caffeine. Acetaminophen (0.5–2.0 mM), either alone or in combination with caffeine or salicylic acid induced proliferation of p1rICD cells when they were confluent. This was in contrast to an inhibitory effect of acetaminophen on cell growth if the cells were subconfluent. Normally there is little proliferation of inner medullary cells in vivo and proliferating inner medullary cells have a reduced tolerance for hyperosmolality. Thus it would appear that acetaminophen-induced proliferation appears to sensitize the cells to toxicity, which was demonstrated by evidence of DNA damage and apoptosis. Other workers have also suggested that acetaminophen induced renal cell injury may be mediated via an apoptotic process.
Lorz and colleagues demonstrated that acetaminophen can induce apoptosis in murine tubular cells that was not mediated via the Fas Fas-ligand pathway or mitochondrial injury. Rather, apoptosis was associated with a decrease in antiapoptotic Bcl-xL protein levels and evidence of increased endoplasmic reticulum stress.
Compound analgesics containing aspirin, caffeine and phenacetin (or its major metabolite, acetaminophen) have a synergistic effect in the development of chronic nephrotoxicity. Aspirin inhibits the action of cyclooxygenase but not prostaglandin hydroperoxidase in the prostaglandin synthase complex. Clinically little or no aspirin reaches the kidney as aspirin is deacetylated to salicylate. Salicylate, as well as depleting renal glutathione levels increasing the potential for oxidative injury, potentiates acetaminophen effects on renal inner medullary cell proliferation and susceptibility to hyperosmotic injury. Likewise caffeine can potentiate acetaminophen induced cellular injury. Caffeine influences a number of pathways involved in cellular response to DNA damage. Caffeine also blocks p53 activation in response to DNA damage as well as blocking DNA repair.
Clearly, acetaminophen, either alone or in combination with other agents can have significant effects on renal cellular function leading to nephrotoxicity. These may be mediated via intracellular metabolic pathways producing reactive metabolites and/or by direct effects on cell survival.
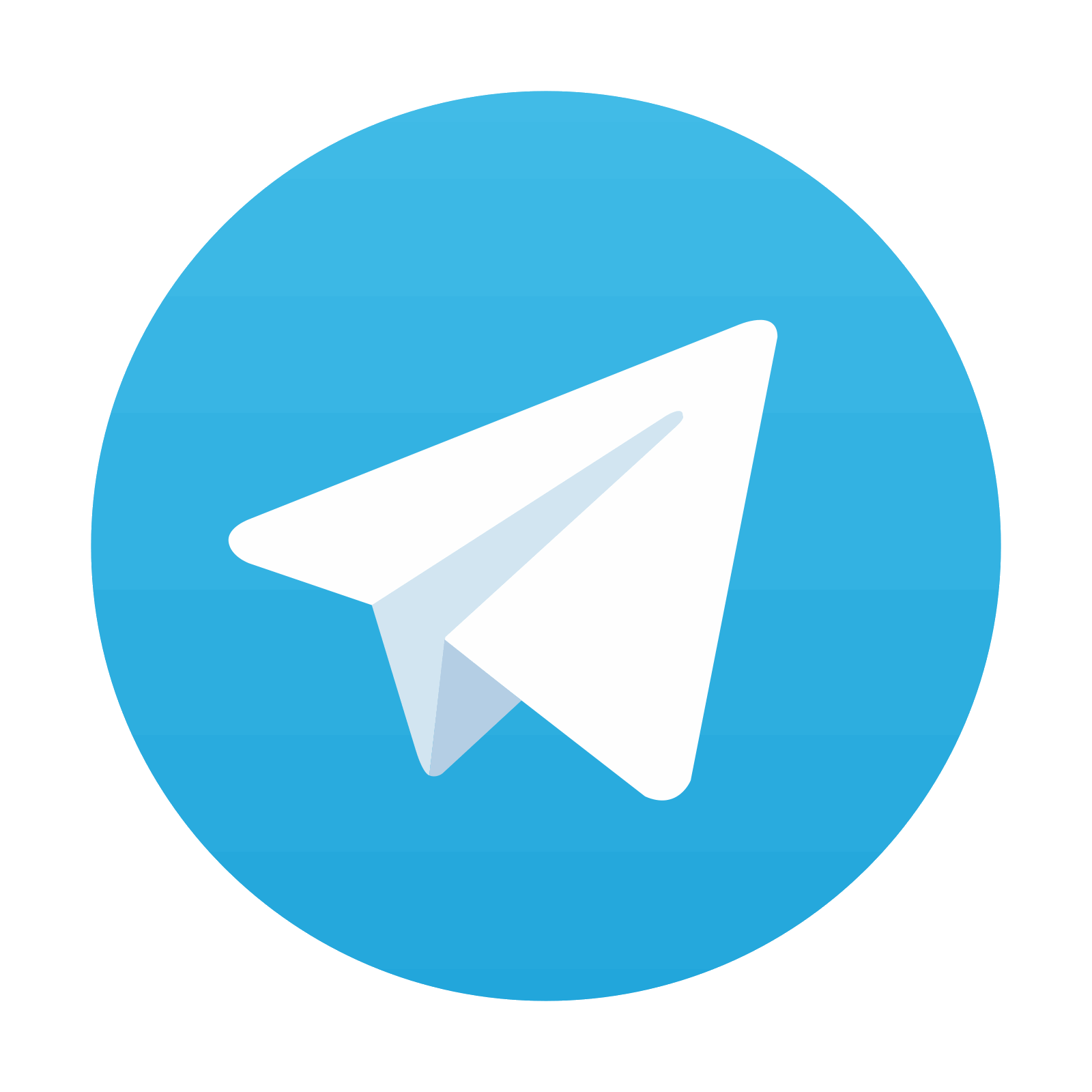
Stay updated, free articles. Join our Telegram channel
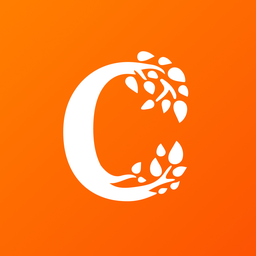
Full access? Get Clinical Tree
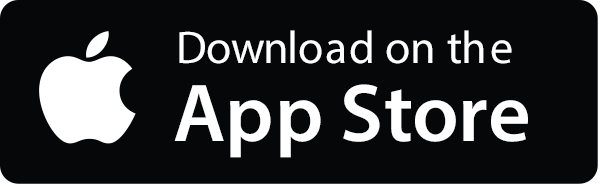
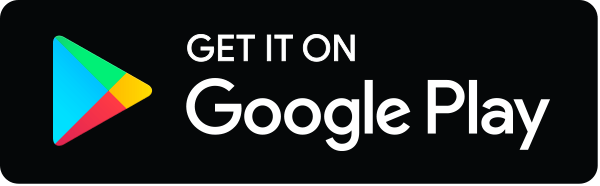