Naoki Yoshimura, MD, PhD, Michael B. Chancellor, MD The medical, social, and economical impact of urinary incontinence, overactive bladder, and voiding dysfunction is staggering (Yoshimura and Chancellor, 2002). In the United States, there are an estimated 34 million community-dwelling men and women with an overactive bladder (Hu et al, 2004). It costs an estimated $19.5 billion and $12.6 billion a year in the United States to manage urinary incontinence and the overactive bladder, respectively (Hu et al, 2003; Hu et al, 2004). With the continued aging of the populations in all developed countries, the problems associated with bladder control will certainly continue to increase. In this chapter, we review the neuromuscular physiology and pathophysiology of the bladder and urethra. We will discuss what is new in pharmacology that may better help our patients with voiding dysfunction. Finally, we will speculate about future treatment methods to conquer urinary incontinence. The micturition process can be visualized as a complex of neural circuits in the brain and spinal cord that coordinate the activity of smooth muscle in the bladder and urethra (Torrens and Morrison, 1987; de Groat et al, 1993a; Yoshimura and de Groat, 1997). These circuits act as on-off switches to alternate the lower urinary tract between two modes of operation: storage and elimination. Injuries or diseases of the nervous system in adults can disrupt the voluntary control of micturition, causing the reemergence of reflex micturition and resulting in detrusor overactivity and urgency incontinence (Fig. 60–1) (Torrens and Morrison, 1987; Wein, 1992; de Groat et al, 1993a; Yoshimura and de Groat, 1997). Because of the complexity of the central nervous control of the lower urinary tract, urgency incontinence can result from a variety of neurologic disorders. In addition, urgency incontinence may be due to intrinsic detrusor myogenic abnormalities, resulting in detrusor overactivity (Brading, 1997b). The morphology and function of the detrusor wall are reviewed, including new insights into the urothelium. This chapter reviews studies in humans and animals that provide insights into The bladder can be divided into two parts: a body lying above the ureteral orifices and a base consisting of the trigone and bladder neck, because the two areas are different but homogeneous within themselves regarding neuromorphology and neuropharmacology (Elbadawi and Schenk, 1966). Histologic examination of the bladder body reveals that myofibrils are arranged into fascicles in random directions (Donker et al, 1982). This architecture differs from the discrete circular and longitudinal smooth muscle layers in the ureter or gastrointestinal tract. The bladder outlet is composed of the bladder base, urethra, and external urethral sphincter (Fig. 60–2). The bladder base has a laminar architecture with a superficial longitudinal layer lying beneath the trigone. A muscle layer deep to the superficial layer is continuous with the detrusor (Tanagho, 1982; Dixon and Gosling, 1987; Zderic et al, 1996). The smaller muscle bundles of the deep muscle layer in the bladder base exhibit a predominantly circular orientation. (From Torrens M, Morrison JFB. The physiology of the urinary bladder. Berlin: Springer-Verlag; 1987. p. 1.) It is debatable whether the detrusor or trigone muscles project into the proximal urethra (see Fig. 60–2). Embryologic data support the concept of a separate origin for muscles of the bladder and urethra (Dixon and Gosling, 1987; Zderic et al, 1996). Histologic studies show that the longitudinal muscle of the bladder base extends distally into the urethra to form an inner longitudinal layer (Hutch and Rambo, 1967; Tanagho, 1982). Examination of adult and fetal specimens shows that striated and smooth muscles coalesce in the urethra and interdigitate with the fibrous prostatic capsule (Oelrich, 1980). Conversely, a complete and competent ring of smooth muscle at the male bladder neck has been described (Gosling, 1999). No such collar of muscle is identified in the female. The maintenance of continence in men and some women with destruction or opening of the bladder neck argues that the bladder neck may not be the principal site of urinary continence (Chapple et al, 1989). The importance of the bladder neck as the principal zone of maintaining continence remains controversial. The bladder neck serves an important function in reproduction. In men, closure of the bladder neck facilitates antegrade ejaculation. This is accompanied through a rich noradrenergic innervation by sympathetic nerves that actively contract the bladder neck during ejaculation. However, in women, the density of adrenergic innervation in the bladder neck is reportedly less than that in men (de Groat and Booth, 1993). Understanding voiding and continence requires some working knowledge of the contractile properties of smooth and striated muscle. The contractile properties of bladder smooth muscle cells are well suited for either urine storage or release. Filling the bladder at a slow physiologic rate maintains an intravesical pressure of less than 10 cm H2O (Klevmark, 1974). Acute denervation of the bladder does not appreciably alter this low filling pressure (Langley and Whiteside, 1951). This concept has been used to support the hypothesis that the intrinsic myogenic or viscoelastic properties of cellular and extracellular components are major contributors to low-pressure bladder filling and compliance (see the section Mechanisms of Detrusor Overactivity). Conversely, neural input is required for the rapid and sustained smooth muscle contraction accompanying voiding. What basic bladder hydrodynamics and biomechanical properties should practicing urologists know, and why should they know them? These are some of the questions we attempt to answer in this section. We lay the foundation of the relationship between bladder shape, size, pressure, and tension as expressed by the Laplace law (Chancellor et al, 1996). Marquis Pierre-Simon de Laplace (1779 to 1827) has been called the Newton of France. Laplace made the keen observation that the tension in the wall of a container necessary to contain a given pressure is directly proportional to the radius of curvature at any point. This is the Laplace law. The Laplace equation states that there is a direct relationship between wall tension and intravesical pressure and bladder size. In this equation, T is tension, P is intravesical pressure, R is bladder radius, and d is wall thickness. During bladder filling, Pves is relatively constant. With a fully distended bladder, d, because of its relative thinness, is ignored relative to the other parameters unless a hypertrophied wall exists. Thus, T = P • R/2 approximates tension in the full normal bladder (Fig. 60–3). The viscoelastic behavior of the bladder and urethra depends on both neuromuscular and mechanical properties. Mechanical properties vary with the magnitude of stretch (distention), even in tissue deprived of adenosine triphosphate (ATP) (e.g., postmortem). Mechanical properties are extremely sensitive to tissue structure and composition. In the bladder and urethra, collagen and elastin content have a profound influence on the viscoelastic properties when these tissues are subjected to stress (force per area). Besides smooth muscle, the human bladder is composed of roughly 50% collagen and 2% elastin. With injury, obstruction, or denervation, collagen content increases (Macarak and Howard, 1999). When contractile protein content exceeds collagen, greater distensibility is achieved (compliance). Conversely, when collagen levels increase, compliance falls. Bladder compliance (C) is defined as the change in volume (V) relative to the corresponding change in intravesical pressure (P): A decrease in compliance or efferent neural input can alter wall tension, cause afferent firing, and thereby change bladder sensations and the volume threshold for micturition. With increasing bladder volume, wall stress or tension (T) increases, as defined by the Laplace equation (see Fig. 60–3). The changes in the thickness of the lamina propria and the detrusor are mechanical requirements for the bladder to accommodate increasing urine volume. During filling, the lamina propria thins at a faster rate than the muscle wall. It has been proposed that bladder wall thinning during filling is the result of a rearrangement of the muscle bundles and also alteration of collagen coil structure (Macarak and Howard, 1999). A combination of muscle and connective tissue spatial changes is required to accommodate urine at low intravesical pressures (Chang et al, 1999). Micturition relies on a neurally mediated detrusor contraction, causing Pdet to rise without a significant change in Pabd. To assess the strength of a detrusor contraction, Pdet alone is an insufficient measure. A muscle can use energy either to generate force or to shorten its length. Because the bladder is a hollow viscus, the force developed contributes to Pdet, whereas the velocity of shortening relates to urine flow (Q). There is a trade-off between generating Pdet and urine flow. This has been nicely reviewed by Griffiths (1988). If urethral resistance is low, as in women with sphincter insufficiency and even in normal continent women, Pdet may be almost undetectable; yet, these women with modest Pdet would have normal flow rates. The trade-off between Pdet and Q resembles a curve for constant mechanical power (W) in which In addition to important molecular and cellular parameters, tissue- and organ-level bladder properties are important to the function of the bladder during filling (Damaser, 1999). Fundamental mechanical properties include the stress-strain relationship, viscoelasticity, and deformation of bladder tissue. Whole-bladder properties include bladder shape, mass, and distention. Organ-level pressure-volume assessment is usually achieved by the cystometrogram. Although it is an essential tool for the urologist, the cystometrogram alone cannot rigorously distinguish between the effects of changes in tissue compliance, and the shape and wall stress distribution of the whole organ. For example, changes in the cystometrogram in spinal cord injury could be a result of alterations in both bladder shape (Ogawa et al, 1988) and tissue properties. Thus to assess intrinsic changes in bladder wall properties, proper assessment of the changes in bladder function requires an understanding of bladder wall biomechanical properties. The biomechanical components contributing to tissue mechanical responses have been studied in isolated detrusor tissue (Wagg and Fry, 1999). However, an ongoing problem is to determine whether clinically observed alterations in detrusor function are due to changes in the contractile apparatus or in the surrounding extracellular matrix. The complexity of the bladder shape raises similar questions. The bladder can be mechanically idealized as a thin-walled shell. In the theory of thin shells, surface geometry is an integral part of how wall tensions are spatially distributed (Flügge, 1973). That is, a change in surface geometry can alter the stress distributions independent of changes in the mechanical properties. Thus focal points of high curvature on the bladder surface (Fig. 60–4) can potentially induce regions of localized stress concentrations and may be related to bladder deformity, for example, during the spinal cord injury disease process. These phenomena have been underscored by the bladder shape modeling work of Damaser and Lehman (1995), who demonstrated the sensitivity of bladder wall stress distribution on bladder shape. Although it is typically modeled as a spheroid, the bladder is probably one of the more irregularly shaped anatomic structures. Irregularity in bladder shape, especially when it is full, is due to contact with surrounding pelvic structures. Note in particular the complex spatial distribution of surface curvatures, including pointed regions of high curvature and rapid transitions between elliptical (both principal curvatures are positive) and hyperbolic (or saddle-shaped, where one principal curvature component is negative) regions (see Fig. 60–4). By use of the techniques developed by Sacks and colleagues (1993, 1999), this roughness can be removed and the original smooth in-vivo surface recovered (Fig. 60–5). There are several universal characteristics of smooth muscle: Based on the assumption of a spherical bladder, the circumference of a 400-mL capacity bladder is approximately 30 cm. If the bladder empties to a residual urine volume of 10 mL, the circumference would be only 8 cm. To accomplish this feat, the detrusor would have a change in muscle length of 75%. If the bladder were to be made of skeletal muscle instead, the maximum length change would be only about 30%. The maximum “skeletal muscle bladder” emptying would be only 70% of its contents, leaving a residual urine of 120 mL. Thus the bladder requires the unique property of smooth muscle to accomplish its job. What is assumed to be the stronger skeletal muscle is not up to the job of bladder emptying (Gabella, 1995; Brading et al, 1996; Brading, 1999). The individual smooth muscle cells in the bladder wall are small spindle-shaped cells with a central nucleus; fully relaxed, they are several hundred micrometers long with a 5- to 6-µm maximum diameter. Skeletal muscle fibers are some 20 times wider and thousands of times longer (Smet et al, 1996). Smooth muscle has a unique range of physiologic properties and consists of sheets containing many small spindle-shaped cells linked together at specific junctions. Each cell has a single nucleus. No cross striations are visible under the microscope, but like skeletal and cardiac muscle, smooth muscle cells contain actin and myosin. In addition, they contain cytoskeletal intermediate filaments that assist in transmission of the force generated during contraction to the neighboring smooth muscle cells and connective tissue. Although there are no Z lines in smooth muscle, they have a functional counterpart in dense bodies that are distributed throughout the cytoplasm and that serve as attachments for both the thin and the intermediate filaments. The thin and thick filaments of smooth muscle fibers are arranged as myofibrils that cross the fibers obliquely in a lattice-like arrangement. The filaments of contractile proteins are attached to the plasma membrane at the junctional complexes between neighboring cells (Fig. 60–6). A comparison of some of the properties of smooth and skeletal muscle is listed in Table 60–1 (Chacko et al, 1999). Table 60–1 Comparison of the Properties of Skeletal and Smooth Muscle Detrusor smooth muscle contracts and shortens by interaction between thin and thick filaments. The thin actin filaments are anchored at the membranes on the dense bands, or in the cytoplasm on the dense bodies, and interact with the thick filaments through cross bridges formed from the heads of myosin molecules. In both cases, the contractile mechanism is a myosin-activated system. Cross-bridge cycling is initiated when the ATPase activity of the myosin heads is switched on. This is achieved by phosphorylation of two light chains on the cross bridge, through a specific enzyme, myosin light-chain kinase, that is activated by a rise in the intracellular calcium concentration (Hai and Murphy, 1989; Gunst et al, 1993; Andersson and Arner, 2004). Changes in the contractile proteins occur in developing bladders and also during bladder hypertrophy (Wang et al, 1995; Wu et al, 1995; Sjuve et al, 1996). The bladder muscle has a broad length-tension relationship, allowing tension to be developed over a large range of resting muscle lengths (Uvelius and Gabella, 1980). The tissue shows viscoelasticity that influences muscle tension and is manifested as total bladder wall tension (Venegas, 1991). Isolated detrusor strips show spontaneous mechanical activity to a variable extent. It is more frequently seen in bladders from small mammals (Sibley, 1984) but can also be seen in muscle strips from human detrusor. However, spontaneous fused tetanic contractions, such as those commonly seen in smooth muscles from the gastrointestinal tract and uterus, are almost never seen in normal bladders. The smooth muscle of the detrusor is able to support a regenerative action potential from a resting potential of −50 to −60 mV (Montgomery and Fry, 1992; Fry et al, 2002). The action potentials have marked after-hyperpolarizations, and several different K+ channels, such as delayed rectifier and transient outward channels and both small and large calcium-activated K+ channels (SKCa and BKCa, respectively), appear to be involved in determining their shape (Fujii et al, 1990; Brading and Turner, 1996; Heppner et al, 1997; Andersson and Arner, 2004). The upstroke is supported by calcium influx through L-type Ca2+ channels (Rivera, 1998). In addition, a K+ channel opened by reduced intracellular ATP has been demonstrated (Bonev and Nelson, 1993), which, on activation, is profoundly inhibitory to the spontaneous activity (Foster et al, 1989). Several other conductances, which include a nonspecific cation channel linked to the P2X receptor (Inoue and Brading, 1990) and stretch-activated cation channels (Wellner and Isenberg, 1993), have been demonstrated in detrusor smooth muscle. In response to acetylcholine released from parasympathetic nerve terminals, muscarinic M3 receptors are thought to induce detrusor muscle contractions by calcium entry through nifedipine-sensitive L-type Ca2+ channels (Andersson and Arner, 2004; Andersson and Wein, 2004; Schneider et al, 2004a, 2004b), in addition to increased polyphosphoinositide hydrolysis resulting in inositol 1,4,5-trisphosphate (IP3) production and release of intracellular calcium stores (Iacovou et al, 1990; Eglen et al, 1994; Harriss et al, 1995; Hashitani, Bramich et al, 2000; Fry et al, 2002; Braverman et al, 2006a), although the importance of L-type channel activation in muscarinic M3 receptor–mediated detrusor bladder contractions is still a matter of debate (Fry et al, 2002; Hashitani et al, 2000; Schneider et al, 2004a, 2004b; Braverman et al, 2006a, 2006b; Frazier et al, 2008). The calcium influx through L-type Ca2+ channels also triggers calcium-induced calcium release through ryanodine receptors and activates BKCa channels to generate the membrane after-hyperpolarization (Hashitani and Brading, 2003). The opening of the K+ channel repolarizes the membrane potential by the efflux of positively charged potassium ions from the cell (Mostwin, 1986). In addition, increased polyphosphoinositide hydrolysis after M3 receptor activation can also generate diacylglycerol in the membrane; diacylglycerol can activate protein kinase C, which may be involved in generating the tonic element of the response through modulation of Ca2+ and K+ channels (Zhao et al, 1993). Like skeletal and cardiac muscle, smooth muscle contracts when intracellular calcium concentration rises. Because smooth muscle does not possess the tubules of the “T system,” the rise in calcium concentration occurs through calcium influx through voltage-gated Ca2+ channels in the plasma membrane or release from the sarcoplasmic reticulum after activation of receptors that increase the formation of IP3. Although calcium serves the same triggering role in all muscle types, the mechanism of activation is different in smooth muscle. The contractile response is slower and longer lasting than that of skeletal and cardiac muscle. This can be explained by the slowness with which smooth muscle is able to hydrolyze ATP during the contractile process. A calcium-binding protein called calmodulin regulates the interaction between actin and myosin. The thin filaments lack troponin and are always ready for contraction. The slow and relatively steady generation of tension enables smooth muscle to generate and to maintain tension with relatively little expenditure of energy (Fig. 60–7 and Table 60–2). (Redrawn from Brading A. Cellular biology. In: Abrams P, Khoury S, Wein A, editors. Incontinence. Plymouth [UK]: Health Publications; 1999. p. 73.) Table 60–2 Detrusor Smooth Muscle Contraction Sequence The rise in cytoplasmic calcium concentration brought on by the action potential results in binding of calcium to calmodulin. Calcium-bound calmodulin is then capable of activating myosin light-chain kinase, permitting it to phosphorylate the myosin type II light chain. Phosphorylation of the light chain allows the myosin to interact with actin, leading to force generation (White et al, 1993; Chacko et al, 1994; Andersson and Arner, 2004). The actual geometric arrangement of the actin-myosin complex that permits force generation is unclear. Different isoforms of the myosin heavy chain have been defined by Chacko and associates (1994), as well as the components of the regulatory system linking cytoplasmic calcium levels to contraction (White et al, 1993). The smooth muscle myosin heavy chain is encoded by a single gene, and two heavy-chain variants formed by alternative splicing are identified (SM1 and SM2). Two additional myosin heavy-chain isoforms, SM-A and SM-B, are then formed by alternative splicing in the amino-terminal region, enabling four possible isoforms: SM1-A, SM1-B, SM2-A, and SM2-B (Babu et al, 2000). Because the SM-B isoform is shown to propel actin at a faster velocity (Lauzon et al, 1998), the relatively high expression of SM-B isoform in the bladder shown at the mRNA level (Arafat et al, 2001) would contribute to a comparatively fast smooth muscle type of the bladder (Andersson and Arner, 2004). The lack of fused tetanic contractions in normal detrusor smooth muscle strips suggests that there is poor electrical coupling between smooth muscle cells (Uvelius and Mattiasson, 1986). Measurements of tissue impedance support the observation that the detrusor is less well coupled electrically than other smooth muscles (Brading and Mostwin, 1989; Parekh et al, 1990). Poor coupling could be a feature of a normal detrusor that prevents synchronous activation of the smooth muscle cells during bladder filling. Nevertheless, some degree of coupling within a muscle bundle clearly does exist, because it is possible to measure the length constant of a bundle (Seki et al, 1992). There is also evidence for gap-junction coupling between detrusor cells in humans and guinea pigs, detected by whole-cell patch clamp recordings (Wang et al, 2006) and Ca2+ imaging (Neuhaus et al, 2002), respectively. Significant expression of connexins 43 and 45, gap-junction proteins, is found in human detrusor muscles (John et al, 2003; Wang et al, 2006). However, electrical couplings between detrusor cells seem to be reduced during postnatal development because coordinated, large-amplitude, low-frequency contractile activity is seen in the neonate rat bladder declines and is replaced by low-amplitude, high-frequency, more irregular activity in older rats, which appears to depend on the disruption of the intercellular smooth muscle communication (Szell et al, 2003). It has also been suggested that a change in the properties of the cell coupling may underlie the generation of the uninhibited detrusor contractions occurring in overactive and aging bladders (Seki et al, 1992; Brading, 1997b; Brading, 2006). As the bladder fills, the myocytes are stretched, leading to activation of nonselective cation channels that permit rapid entry of sodium and of some calcium (Wellner and Isenberg, 1993). Entry of cations depolarizes the smooth muscle membrane potential. If the extent of stretch is mild, there is low activation, and the membrane potential rests at a more depolarized level, predisposing the cell to activation by lower levels of muscarinic agonists. If the extent of the stretch is more significant, the activation of cation channels may be sufficient to depolarize the cell sufficiently for initiation of an action potential. Although individual cells may contract spontaneously, contraction of the bladder as a whole generally requires stimulation by parasympathetic nerves (Andersson, 1993). When the membrane is sufficiently depolarized, L-type Ca2+ channels open and Ca2+ channels in the sarcoplasmic reticulum open, flooding the cell with calcium and resulting in an action potential, thereby leading to spontaneous phasic activity in the bladder (Mostwin, 1986; Kumar et al, 2005). Between contractions, the sarcoplasmic reticulum accumulates calcium to levels far above those of the cytosol by means of a calcium ATPase (Wall et al, 1990). Calcium stores in the sarcoplasmic reticulum can be released in the absence of action potentials by exposure to caffeine, which renders the Ca2+ channels sensitive to normal ambient cytoplasmic calcium levels. Recent evidence suggests that the “normal” bladder may be spontaneously active and that exaggerated spontaneous contractions could contribute to the development of detrusor overactivity. In a rat model for detrusor overactivity, local areas of spontaneous contractions are increased and more coordinated in partial outlet-obstructed rat bladders (Drake et al, 2003). However, it is still not clear which cells generate spontaneous activity in the bladder. As mentioned before, detrusor myocytes could be spontaneously active, and electrical coupling through gap junctions could trigger spontaneous contractions (Brading, 1997b, 2006). Alternatively, another population of cells in the bladder known as interstitial cells, or myofibroblasts, has been proposed for a pacemaking role in spontaneous activity of the bladder (Andersson and Arner, 2004; Kumar et al, 2005). Interstitial cells have been identified in the human and guinea pig ureter, urethra, and bladder body (Kumar et al, 2005; Hashitani, 2006; Fry et al, 2007). In the human bladder, subepithelial interstitial cells, which are also called myofibroblasts, stain for vimentin and α–smooth muscle actin but not for desmin (Fry et al, 2004). These cells are linked by gap junctions consisting of connexin 43 proteins and make close appositions with C-fiber nerve endings in the submucosal layer of the bladder, suggesting that there is a network of functionally connected interstitial cells immediately below the urothelium that may be modulated by other nerve fibers (Fry et al, 2004) (Fig. 60–8). ATP can induce inward currents associated with elevated intracellular Ca2+ in isolated suburothelial interstitial cells (Fry et al, 2007). Immunohistochemical studies show the expression of P2Y receptors, most notably P2Y6 receptors, and M3 muscarinic receptors in suburothelial interstitial cells from guinea pigs (Fry et al, 2007; Grol et al, 2009). In the human bladder, increased expression of muscarinic M2 and M3 receptors in vimentin-stained suburothelial interstitial cells is found and correlates with the urgency score in humans with idiopathic detrusor overactivity (Mukerji et al, 2006). Because ATP or acetyl choline (ACh) is known to be released from the urothelium during bladder stretch, suburothelial interstitial cells are in an ideal position between the urothelium and nerve endings to modify a sensory feedback mechanism. Application of a nitric oxide donor sodium nitroprusside (SNP) also attenuates an increase in intracellular Ca2+ and current responses to ATP in guinea pig interstitial cells, suggesting the cGMP-dependent inhibition of cell activity (Sui et al, 2008). Interstitial cells are also found in the detrusor layer and shown to be spontaneously active (Kumar et al, 2005). These cells are stained for c-Kit and located along both boundaries of muscle bundles in the guinea pig bladder (McCloskey and Gurney, 2002; Hashitani et al, 2004; Hashitani, 2006). These cells can fire Ca2+ waves in response to cholinergic stimulation by M3 muscarinic receptor activation and can be spontaneously active, suggesting that they could act as pacemakers or intermediaries in transmission of nerve signals to smooth muscle cells (McCloskey and Gurney, 2002; Johnston et al, 2008) (Fig. 60–9). However, Hashitani and colleagues (2004) have also suggested that interstitial cells in the detrusor may be more important for modulating the transmission of Ca2+ transients originating from smooth muscle cells rather than being the pacemaker of spontaneous activity because spontaneous Ca2+ transients occur independently in smooth muscles and interstitial cells. It has also been demonstrated that the c-Kit tyrosine kinase inhibitor Glivec decreased the amplitude of spontaneous contractions in the guinea pig bladder (Kubota et al, 2004, 2006) and in muscle strips from the overactive human bladder, in which c-KIT–positive cells were increased compared with normal subjects (Biers et al, 2006), suggesting that targeting these receptors expressed in intradetrusor interstitial cells may provide a new approach for treating overactive bladder. In addition, following application of SNP (a nitric oxide [NO] donor), interstitial cells throughout the bladder, but not detrusor muscle cells, demonstrate cyclic guanosine monophosphate (cGMP) immunoreactivity (Smet et al, 1996; Gillespie et al, 2004). Thus increased levels of cGMP found in interstitial cells by using phosphodiesterase-5 inhibitors, for example, may diminish synchronicity between detrusor muscle bundles (Hashitani, 2006). These cells are also a source of prostaglandin E2 (PGE2) because of their expression of cyclooxygenase and a reduction in spontaneous activity of bladder muscle strips by use of prostaglandin E2 receptor (EP) antagonists in rabbits (Collins et al, 2009). If a smooth muscle is stretched, there is a corresponding increase in tension immediately after the stretch. This is followed by a progressive relaxation of the tension toward its initial value. This property is unique to smooth muscle and is called stress relaxation (Chancellor et al, 1996). The main constituents of bladder wall stroma are collagen and elastin in a matrix composed of proteoglycans. The main cells are fibroblasts. The passive mechanical properties of the bladder wall depend on the viscoelastic properties of the stroma and of the relaxed detrusor muscle (Cortivo et al, 1981). The stroma has commonly been considered a passive low-metabolic tissue that fills out the space between muscle bundles, vessels, and nerves. In recent years, the important role of the stroma in the adaptation of the bladder to pathophysiologic conditions has been more appreciated (Macarak and Howard, 1999). Bladder hypertrophy is likely to involve an interaction of stroma and smooth muscle. In arteries, disruption of elastin in the stroma can stimulate proliferation of smooth muscle (Li et al, 1998). Although no such mechanisms are yet known in the bladder, it is possible that there could be a more intimate relationship between changes in the composition of the stroma and muscle function and growth than is appreciated at present. Most of the bladder wall collagen is found in the connective tissue outside the muscle bundles. Changes in the relative amounts of muscle and nonmuscle tissue in the bladder wall would therefore influence collagen concentration. A number of different collagen types have been identified. In the bladder, types I, III, and IV are the most common (Macarak et al, 1995; Andersson and Arner, 2004). Landau and coworkers (1994) developed morphometric and histochemical techniques to determine the percentage volume of connective tissue in the bladder wall and to measure the two major types (I and III) of collagen. These methods quantitate three parameters of bladder ultrastructure: percentage volume of connective tissue, ratio of connective tissue to smooth muscle, and ratio of type III to type I collagen. These parameters have been shown to be abnormally elevated in patients with bladder disease compared with normal patients. They further studied the ultrastructural changes that occur in the wall of dysfunctional bladders to determine the ability of new urodynamic techniques to reliably detect the clinical effect of these histologic changes. The study included 29 consecutive patients undergoing bladder augmentation. Preoperative urodynamic evaluation included measurement of the total bladder capacity, pressure-specific bladder volume, and dynamic analysis of bladder compliance. Full-thickness bladder biopsy specimens were obtained from the dome of the bladders during augmentation. The percentage of connective tissue and the ratio of connective tissue to smooth muscle were determined for all patients. These histologic results were compared with previously established normal values. All 29 patients had decreased bladder compliance, even though 9 had a normal bladder capacity. The ratio of connective tissue to smooth muscle was significantly increased in poorly compliant versus normal bladders. The ratio of type III to type I collagen was also significantly elevated. One can conclude that the poor storage function of poorly compliant bladders is secondary to an alteration in the connective tissue content of the bladder wall, especially increased collagen type III. In the rat, infravesical obstruction or bladder denervation induces hypertrophy of the detrusor smooth muscle and, in turn, a decrease in the collagen concentration (Uvelius and Mattiasson, 1984, 1986). Aging is associated with a relative decrease in smooth muscle, in both men and women, relative to collagen content (Susset et al, 1978; Lepor et al, 1992). This could perhaps be related to the decreased packing density of submucosal collagen during aging (Levy and Wight, 1990). Perhaps the most comprehensive work on bladder collagen has been performed by Macarak and Howard (1999), who have speculated that connections must exist between the tension-generating elements (i.e., the smooth muscle cells) and the other components of the bladder. In bladders that become noncompliant (e.g., from spinal cord injury), it is likely that there is some interference with the ability of the collagen fibers to alter their tortuosity. This, predictably, would reduce total bladder capacity. Further studies are required to establish the relationship between compliance changes and the passive mechanical elements of the bladder wall that make up its structural protein matrix. Elastic fibers are amorphous structures composed of elastin and a microfibrillar component located mainly around the periphery of the amorphous component (Rosenbloom et al, 1995). In the mature fiber, the amorphous component composes about 90%. The microfibrils contain a number of glycoproteins. Elastin fibers are sparse in the bladder, compared with collagen, but are found in all layers of the bladder wall (Murakumo et al, 1995). In spinal cord–injured rats, the elastin/collagen ratio first increased and is well correlated with increased bladder compliance due to bladder overdistention up to 6 weeks after injury, including a bladder areflexia phase. Then the ratio is reduced as bladder compliance is decreased due to the emergence of detrusor overactivity 10 weeks after injury, suggesting a potential role for elastin in the modulation of bladder compliance (Nagatomi et al, 2005; Toosi et al, 2008). The bladder has a multilayer vascular plexus, and this fact has morphologic as well as functional implications. When it is carefully examined, the underside of the epithelium is depressed by numerous grooves that are occupied by a dense network of blood capillaries. These vascular grooves allow a large number of capillaries to run at a distance of only a few tenths of a micron from the epithelium (Inoue and Gabella, 1991). The subepithelial capillary plexus may be associated with maintenance of the barrier function of the urothelium, reducing any exposure of the detrusor smooth muscle to substances diffusing from the urine (Hossler and Monson, 1995). It may also play a role in epithelial transport function and be necessary for urothelial metabolism. Because of the large increase in surface area of the bladder wall during filling, the blood vessels must be able to lengthen considerably. To maintain good blood flow, mechanisms may be needed to ensure that the overall resistance of the vessels, as they lengthen, does not increase sufficiently to reduce the effective perfusion of the tissue. Several groups have investigated the effects of bladder filling on the blood flow. The majority of reports have shown that the blood flow is reduced by distention (Batista et al, 1996; Greenland and Brading, 1996). In patients with a low compliant bladder, there is a marked increase in the intravesicular pressure and a more pronounced decrease in bladder blood flow compared with normal controls (Ohnishi et al, 1994). The principal determinant of blood flow in the bladder wall seems to be intramural tension. During normal filling, the blood flow is able to adapt to the large increase in surface area until the pressure increases in the bladder (Greenland and Brading, 1996). When the detrusor is deprived of oxygen or a metabolic substrate, as would occur in ischemia, its contractile ability rapidly declines (Levin, 1983; Zhao et al, 1991; Pessina et al, 1997; Levin et al, 2003). It has been suggested that ischemia and reperfusion might lead to damage to intramural neurons and result in the patchy denervation and altered smooth muscle function seen in bladders of patients with detrusor overactivity (Brading, 1997a). The urothelium consists of a variable number of cell layers, depending on the species (Fawcett, 1984). There are generally three distinct layers. A layer of basal cells are germinal in nature and 5 to 10 µm in diameter. Intermediate cells are superficial to these and approximately 20 µm in diameter, and a layer of umbrella cells forms the luminal surface of the urothelium (Lewis, 2000; Apodaca, 2004). These umbrella cells are the largest epithelial cells in the body, measuring 100 to 200 µm in diameter; they are polyhedral, are generally hexagonal, and can flatten and have more surface area with stretching. They may also be multinucleate (Kelly, 1984). The surface of the umbrella cells is covered with a glycosaminoglycan layer (Fig. 60–10). The glycosaminoglycan (GAG) layer has been a controversial subject of research into urothelial barrier function. Parsons and associates (Parsons et al, 1990) reported that intravesical treatment of the rabbit bladder with protamine sulfate increased urothelial permeability, to water, urea, and calcium both in vivo and in vitro. This effect was reversed with pentosan polysulfate. They concluded that the protamine sulfate affected the GAG layer and that this was repaired by pentosan polysulfate. However, no microscopic evidence of the anatomic changes was presented in this paper. This study was confirmed by Nickel and coworkers (1998), who compared pentosan polysulfate, heparin, and hyaluronic acid as treatments. The authors concluded that heparin was the best of the three agents in efficacy but pointed out that this may be due to its anti-inflammatory properties. Indeed, the role of the GAG layer may be more in line with an antibacterial adherence function as outlined by Hanno and coworkers (1981). The GAG layer may also be important for the formation and attachment of particulates to the urothelium and stone formation (Hurst, 1994; Grases et al, 1996). However, there are a number of problems with the theory that the GAG layer is the urothelial plasma barrier: Protamine sulfate increases the apical membrane (luminal surface of umbrella cells) permeability to both monovalent cations and anions. This may be reversed on the basis of the concentration of the protamine, the composition of the bathing solution, and the exposure time of the urothelium to protamine. Prolonged exposure to protamine (>15 minutes) is poorly reversible and is thought to be caused by a decrease in paracellular resistance from cell lysis (Tzan et al, 1993, 1994). In an indirect method of examining the GAG layer, Madin-Darby canine kidney (MDCK) cells were transfected with MUC-1, the major GAG of the bladder, with different tandem repeat units to vary the length of the glycosylated chain outside the cell. After this treatment, no difference in the transcellular water and urea permeability was found (Lavelle et al, 1997). In summary, the GAG layer may have importance in bacterial antiadherence and in prevention of urothelial damage by large macromolecules. However, there is no definite evidence that the GAG layer acts as the primary epithelial barrier between urine and plasma. Umbrella cells are so called for their shape, in that they look like umbrellas over the intermediate cell layer. They may cover several intermediate cells and have a stem that may extend to the lamina propria of the urothelium. They are capable of changing shape and can increase their surface area as the bladder fills and, conversely, decrease their surface area as the bladder empties. How this phenomenon occurs is still under debate. The primary theory is that there are a large number of subapical discoid vesicles with an asymmetrical membrane structure just under the apical membrane. These vesicles decrease in number with bladder stretch and increase the electrical capacitance of the luminal surface of the umbrella cells. This is consistent with the exocytosis of these vesicles to increase the cell surface area (Porter et al, 1967). Conversely, the discoid vesicles may be infoldings of membrane that stretch out and disappear with the requirement for increased surface area (Staehelin et al, 1972). These discoid vesicles are associated with a dense network of filaments (Hicks, 1965), which may be connected to the uroplakin plaques (Minsky and Chlapowski, 1978). The insertion of these vesicles into the apical membrane is a microfilament-dependent system, requiring ATP for insertion but not collapse, (Sarikas and Chlapowski, 1986) that does not require an intact microtubule system (Lewis and de Moura, 1982). In membrane physiology, the primary determinant of the permeability of the lipid bilayer is the permeability of the individual leaflets. The leaflet that is least permeable will determine the overall permeability of the membrane (Negrete et al, 1996). In the umbrella cell luminal membrane, there is a unique structure where the membrane has most (70% to 90%) of the apical surface covered with protein plaque, with the remaining area consisting of hingelike regions. The composition of the hinge regions is unclear but may be a protein or lipid. The large plaque units are 0.5 µm to 12 nm thick, with subunits approximately 16 nm from center to center (Walz et al, 1995). They are composed of a class of proteins called uroplakins, which are highly conserved across several species (human, monkey, sheep, pig, dog, rabbit, rat, and mouse). There are four uroplakin proteins: UP Ia (27 kD), UP Ib (28 kD), UP II (15 kD), and UP III (47 kD). Antibodies raised against UP I proteins have shown them to be present only in the umbrella cells of the urothelium, and therefore they may act as specific markers of terminally differentiated umbrella cells (Yu et al, 1994; Sun et al, 1999). The primary function of the uroplakin proteins is hypothesized to be part of the primary plasma-urine barrier. Uroplakins have also been shown to act as the primary attachment site of type 1 piliated uropathogenic Escherichia coli in mice, leading to apoptosis of the umbrella cells that were inhibited by the caspase inhibitor Boc-aspartyl(OMe)-fluoromethylketone (BAF) (Mulvey et al, 1998). Given the highly conserved nature of uroplakins, the same is likely to happen in humans. Coupled with the theory that the apical asymmetrical membrane of the umbrella cells is the primary barrier of the bladder to urine is the observation that cells are joined by tight junctions consisting of four to six interconnecting strands (Peter, 1978). It is the combination of the umbrella cells and tight junction connections that creates the physical barrier to the movement of substances between urine and blood. Once the umbrella cell layer of the urothelium is breached, there is little morphologic (Peter, 1978) or electrophysiologic (Lewis et al, 1976) diffusive permeability evidence (Negrete et al, 1996; Lavelle et al, 1998, 2000) for a barrier to the movement of water and urea. Descriptions of finite passage of substances across the urothelium are well known. In 1856, Kaupp reported that the composition and volume of urine were altered with 12-hour voiding patterns instead of hourly voiding. These changes in volume have also been noted in rats during isovolumetric cystometrograms during 3-hour periods (Sugaya et al, 1997), and the rate of water loss has also been estimated by direct measurement of passive water diffusion in vitro in the rabbit (Negrete et al, 1996). There is a passive permeability to most substances in the blood or urine (Hicks, 1975). The diffusive permeability of the mammalian bladder to water and urea has been directly measured in several species (Lavelle et al, 2000). The permeability of water in the bladder remains remarkably constant, ranging from 4.01 to 5.74 × 10−5 cm/sec, and that of urea, 1.5 to 4.51 × 10−6 cm/sec. Water permeability value in humans is similar at 6.5 × 10−5 cm/sec (Fellows and Marshall, 1972). This value was obtained by estimating the absorption of tritiated water into the plasma after instillation of the tritiated water into the bladder of volunteers. A direct measurement of urothelial diffusive permeability in the human has not yet been made. Breakdown of the umbrella cells in animal models of cystitis has shown increased water and urea permeability. Presumably, leakage of urine into the detrusor is also responsible for the symptoms of cystitis (Lavelle et al, 1998, 2000). This increase in urothelial permeability with cystitis is increased further by distention of the bladder. The hypothesis is that with distention of the bladder, the weakened urothelium with denuded apical umbrella cells and no real barrier in the intermediate or basal cells is further disrupted, thus allowing further egress of urine constituents into the detrusor. Similar breakdown of the apical cells is thought to occur in most forms of infectious cystitis and also in radiation cystitis. Direct measurements of the osmotic effect on permeability have not been performed on urothelium. However, the urothelium maintains an osmotic gradient between plasma (300 mOsm/kg approximately) and urine (100 to 1500 mOsm/kg), depending on the level of water balance and diuresis of the individual. In the normal bladder, the osmotic effects of the urine appear to go unnoticed, and the patients have few or no symptoms. However, once the bladder is inflamed, such as in bladder pain syndrome/interstitial cystitis (BPS/IC), the effects of osmotic gradients become important (Gao et al, 1994). Patients with spinal cord injury or with myelodysplasia tend to have chronic cystitis with bacteriuria and inflamed urothelium. When detrusor activity was increased in the rat by instillation of hyperosmolar compounds, this was accompanied by neurogenic inflammation, including plasma extravasation of Evans blue that could be decreased by pretreatment with the C-fiber afferent neurotoxin capsaicin (Maggi et al, 1990) indicating that hyperosmolar solutions excite afferent nerves. With increased osmolality, detrusor contractions were much stronger and accompanied by blood pressure elevations. These effects were enhanced when the bladder was pretreated with dimethyl sulfoxide to simulate cystitis conditions (Hohlbrugger and Lentsch, 1985; Hohlbrugger, 1987). Increased permeability of the urothelium in inflammatory conditions has become the basis of the potassium sensitivity test, during which the bladder is distended with 400 mM of potassium chloride solution. Potassium penetrates the urothelium in the inflamed bladder and causes pain by activation of bladder afferent nerves (Parsons, 1996). This does not occur in normal bladders. The validity and accuracy of the intravesical potassium chloride test has not yet been proven clinically by multicenter randomized double-blind studies. The fact that changes in the osmolality, pH, and ionic composition of the urine can influence the activity of the detrusor is important in the performance of cystometry. The nature of the infusate could alter the outcome of the test, particularly in the neurogenic bladder, where there are usually some changes in the urothelium because of the chronic bacteriuria (Hohlbrugger, 1995). The apical membrane of the urothelium has a high electrical resistance (Lavelle et al, 1998, 2000), whereas the basolateral membrane resistance is approximately 10-fold lower (Clausen et al, 1979). Active sodium transport across the urothelium has been demonstrated (Wickham, 1964; Lewis and Diamond, 1976). Na+ channels that exist on the apical surface of the umbrella cells and in the cytoplasmic vesicles below the apical surface are primarily amiloride sensitive (inhibition) and aldosterone responsive. However, amiloride-insensitive, cation-selective, as well as amiloride-insensitive, unstable cation channels have also been identified. Both of these channels were found to be degradation products of the amiloride-sensitive Na+ channel. The amiloride-sensitive Na+ channel is hydrolyzed by serine proteases such as kallikrein and urokinase and plasmin (normally found in the urine but produced by the kidney) (Lewis et al, 1995). Sodium that is transported into the cell is removed at the basolateral membrane by a Na+–K+ exchanger. This leaves the cell with a negative intracellular charge. The basolateral membrane contains K+ and Cl− channels, Na+–H+ exchangers, and Cl−–HCO3− exchangers. These channels and exchangers are important in recovery of cell volume during an increase in serosal osmolality (Donaldson and Lewis, 1990). Unfortunately, the precise role of the Na+ channel in the apical membrane of the umbrella cell is unknown. It is possible that the degradation of the channel might follow the filling of the bladder and that the changes in conductance of sodium may be a signaling factor for the bladder and micturition when it reaches capacity. Alternatively, it may be involved in the signaling pathway that allows insertion or removal of apical membrane on expansion of the bladder. Key Points: Urothelium
Relevant Anatomy and Biomechanics
General Concepts
Bladder Biomechanics
Filling Mechanics
Bladder Accommodation
Voiding Mechanics
Tissue Biomechanics and Bladder Function
The Urinary Bladder
Smooth Muscle
Morphology
PROPERTY
SKELETAL MUSCLE
SMOOTH MUSCLE
Cell characteristics
Long cylindrical cells with many nuclei
Spindle-shaped cells with a single nucleus
Maximum cell size (length × diameter)
30 cm × 100 µm
200 µm × 5 µm
Visible striations
Yes
No
Ultrastructure
Sarcomere pattern
No sarcomere pattern
No immediate filaments
Intermediate filaments
Dense bodies
Motor innervation
Somatic
Autonomic
Type of contracture
Phasic
Mostly tonic, some phasic
Contractile activity
Disinhibition of tropomyosin
Active myosin phosphorylation
Sliding filaments
? Sliding filaments
Rapid contraction
Formation of “latch state”
Calcium regulation
Rapid Ca2+ influx via T tubule
Voltage- and receptor-operated Ca2+ channels
Release from internal stores
Basic muscle tone
Neural activity
Intrinsic, extrinsic factors
Force of contraction regulated by hormone
No
Yes
Smooth Muscle Cellular Mechanics
Membrane Electrical Properties and Ion Channels
Excitation-Contraction Coupling in Smooth Muscle
Propagation of Electrical Responses
Smooth Muscle during Filling
Interstitial Cells
Suburothelial Interstitial Cells
Intradetrusor Interstitial Cells
Length-Tension Relationship
Stroma
Bladder Wall Collagen
Bladder Wall Elastin and Matrix
Blood Vessels
Urothelium
Structure
Glycosaminoglycan Layer
The Umbrella Cells
Urothelial Permeability
Ionic Transport
Stay updated, free articles. Join our Telegram channel
Full access? Get Clinical Tree
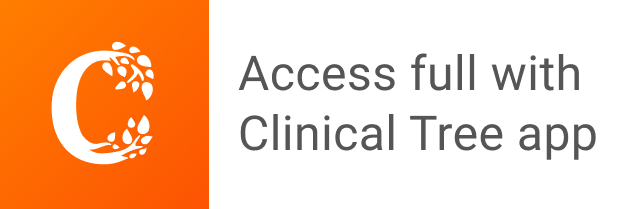