Thirst and the antidiuretic hormone, arginine vasopressin, are the principal elements of a powerful homeostatic system that regulates the “effective” osmotic pressure of body fluids. This variable, usually referred to as “tonicity,” must be important for survival since mechanisms to regulate it are found throughout the animal kingdom, and abnormalities in humans can have adverse effects, especially on central nervous function. However, it is less clear why it is important. It may be that regulating the tonicity of body fluids is mainly an indirect way to minimize changes in the volume of water within cells, particularly those of the brain. 1 It may also be that tight control of intracellular water and solute concentrations is necessary for optimum function of the cell. 2
Introduction
Thirst and the antidiuretic hormone, arginine vasopressin, are the principal elements of a powerful homeostatic system that regulates the “effective” osmotic pressure of body fluids. This variable, usually referred to as “tonicity,” must be important for survival since mechanisms to regulate it are found throughout the animal kingdom, and abnormalities in humans can have adverse effects, especially on central nervous function. However, it is less clear why it is important. It may be that regulating the tonicity of body fluids is mainly an indirect way to minimize changes in the volume of water within cells, particularly those of the brain. It may also be that tight control of intracellular water and solute concentrations is necessary for optimum function of the cell.
The tonicity of body fluids is regulated primarily by raising or lowering total body water to keep it in balance with solute. In a healthy adult, about 55 to 60% of body weight is water. It is slightly lower in women than men, owing to differences in body fat. About two-thirds of body water is intracellular. The rest is in the extracellular space, where it is subdivided further between the interstitial and intravascular (plasma) compartments in a ratio of about 3:1. Thus, a human weighing 70 kg contains about 39 L of water, of which 26 L is intracellular, 10 L is interstitial fluid, and 3 L is plasma.
A change in total body water results in a proportionate change in the volume of all compartments, providing the solute content of the compartments does not also change. The reason is that almost all cell membranes are penetrated by channels composed of aquaporin proteins that permit the passive diffusion of water selectively in response to an osmotic gradient. In contrast, these membranes resist the passage of sodium, potassium, and most other solutes by actively pumping them in or out of the cell (see Chapter 2 ). Thus, a change in the amount of water in one compartment creates an osmotic gradient that results in a rapid flow of water from one compartment to the other, thereby restoring osmotic equilibrium and redistributing the change in volume in a similar proportion to each compartment. Thus, a reduction in the water content of the extracellular compartment due, for example, to increased perspiration, increases the tonicity and reduces the volume of the intracellular and extracellular compartments in equal proportion. A rise in total body water has the opposite but equivalent effect on the tonicity and volume of the two compartments.
In contrast, the volume of extracellular and intracellular water changes in opposite directions if the amount of osmotically effective solute in either compartment changes, but total body water does not. Osmotically effective solutes are those, such as sodium and its anions, which do not equilibrate readily across cell membranes. Thus, if the amount of sodium and its anions in extracellular fluid increases, the effective osmotic pressure or “tonicity” of this fluid also increases, resulting in a rapid osmotically-driven inflow of water from the intracellular compartment until osmotic equilibrium is re-established. The net effect is again an increase in the osmotic pressure of both compartments, but at a level lower than if the shift of water had not occurred. In fact, because of this shift, the effect on plasma sodium concentration of adding a given amount of sodium to the extracellular compartment is the same as if the sodium was distributed throughout total body water. In this circumstance, however, the volume of extracellular fluid increases, while that of intracellular fluid decreases. A reduction in the amount of sodium in extracellular fluid has opposite effects.
Because sodium and its anions normally make up 95% of all solutes, and nearly 100% of osmotically effective solute in extracellular fluid, the measurement of either plasma sodium or osmolarity probably provides an accurate estimate of the “tonicity” or effective osmotic pressure of both the intracellular and extracellular fluids. Urea and glucose contribute little if anything because, normally, they are present in relatively low molar concentrations, and can enter or leave cells very rapidly. In the absence of insulin, however, glucose becomes osmotically effective because it enters cells much more slowly. In that situation, hyperglycemia induces a shift of water from the intracellular to extracellular space, and the tonicity of body fluids is determined much more reliably by measuring plasma osmolarity than plasma sodium. In intracellular fluid (ICF), the major solutes are potassium and its anions, but others including proteins, polyols, and some as yet unidentified solutes are also present.
Body water is in a constant state of flux, owing to obligatory unregulated losses and gains produced by physical activity, the environment, diet, and the metabolism of fat. The rate of evaporative water loss from skin and lungs can vary markedly, depending on ambient temperature and physical activity, but it amounts to at least 10 mL/kg a day (0.7 L in a 70 kg human), even at rest in a comfortably cool environment. The rate of solute-free water loss in urine is tightly regulated by vasopressin but, in humans, it cannot be reduced below a certain minimum required to excrete the load of sodium, chloride, potassium, urea, and other waste solutes derived from the diet and metabolism. This load varies with the diet, but is usually about 700 mosmols a day. Since humans cannot increase their urine osmolarity much above 1400 mosmols/L, the amount of urine required to carry this solute load is at least 7 ml/kg a day (0.5 L a day in a 70 kg human) even under conditions of maximum antidiuresis. The total daily obligatory water loss in a healthy adult is, therefore, at least 17 mL/kg (1.2 L in a 70 kg human), and may be much more at times depending on diet, activity, and temperature. Normally, this loss does not result in dehydration because it is replaced by an equivalent intake of water. Much of the intake is incidental to other physiological needs. About 7 mL/kg (0.5 L in a 70 kg human) comes from water in food, and about 4 mL/kg (0.3 L/day in a 70 kg human) is derived from metabolism of fat. The rest is consumed as beverages with meals in response to various influences, including a slight increase in plasma osmolarity and thirst induced by adsorption of salt and other solutes in food. Thus, water balance is usually maintained at relatively low levels of urine output and discretionary fluid intake. Alterations in this basic state bring into play corrective changes in water excretion and/or intake under control of the antidiuretic and thirst mechanisms.
Vasopressin and Related Peptides
Chemistry
Arginine vasopressin (AVP) is a nonapeptide containing an intrachain disulfide bridge and a tripeptide tail on which the terminal-carboxyl is amidated ( Figure 42.1 ). Substitution of lysine for arginine in position 8 yields lysine vasopressin (LVP), the antidiuretic hormone found exclusively in pigs and other members of the suborder Suina. Vasopressin is structurally similar to oxytocin, another nonapeptide hormone found in the posterior pituitary of all mammals Oxytocin differs chemically from vasopressin by the substitution of isoleucine for phenylalanine at position 3, and of leucine for arginine at position 8. Desmopressin is a potent synthetic analog of vasopressin used therapeutically because of its longer half-life and reduced effects on smooth muscle.

Anatomy
Vasopressin and oxytocin are produced and stored in large amounts by magnocellular neurons in the hypothalamic neurohypophyseal tract. These neurons arise bilaterally in the supraoptic and paraventricular nuclei of the hypothalamus, project medially to merge in the pituitary stalk, and continue through the diaphragm sella into the sella turcica, where they form the posterior lobe of the pituitary ( Figure 42.2 ). Microscopically, the posterior pituitary appears as a densely interwoven network of capillaries, pituicytes, and large nonmyelinated neurons containing many electron-dense secretory granules. The neurons terminate as bulbous enlargements on capillary networks at many different levels throughout the stalk and body of the neurohypophysis. In healthy adults and children, the posterior pituitary usually appears as a hyperintense signal or “bright spot” on T-l weighted magnetic resonance images (MRI) of the brain. The origin of this signal is still unknown, but it appears to be closely related to the content or turnover of vasopressin, and is almost invariably absent not only in patients with the pituitary form of diabetes insipidus, but also in many of those with nephrogenic diabetes insipidus (unpublished data). Vasopressin is also present in the suprachiasmatic nucleus and parvocellular neurons that originate in the paraventricular nucleus and project to the portal veins, anterior pituitary, and many other areas of the brain. Oxytocin is also present in many parvocellular neurons of brain, as well as in the uterus, prostate, and other peripheral tissues.

The hypothalamic neurohypophyseal tract is supplied with blood by branches of the superior and inferior hypophyseal arteries that arise from the posterior communicating and intracavernous portions of the internal carotids. In the body of the neurohypophysis, the arterioles break up into the aforementioned capillary networks that drain directly into the jugular vein by way of the sellar, cavernous, and lateral venous sinuses. In the stalk or infundibulum, the primary capillary networks coalesce into another system, the portal veins, which perfuse the anterior pituitary before discharging into the systemic circulation.
Biosynthesis
Vasopressin is synthesized as part of a protein precursor composed of a signal peptide at its amino terminus, vasopressin, the vasopressin-binding protein, neurophysin II, and a glycosylated peptide, copeptin, at its carboxyl-terminus ( Figure 42.3 ). During or after translocation to the endoplasmic reticulum, the signal peptide is removed and the N-terminus of the vasopressin moiety binds to a pocket in the neurophysin moiety. The prohormone then folds, forms a number of intrachain disulfide bridges, and dimerizes, before moving through the Golgi and into the neurosecretory granules where it is transported down the axon and further processed to yield amidated vasopressin, neurophysin, and copeptin. Vasopressin and its neurophysin are stored in nerve terminals as insoluble complexes which dissociate completely after release into the systemic circulation. Biosynthesis of vasopressin appears to be accelerated by stimuli, such as dehydration or hypertonic saline infusion, which increase secretion. However, this compensatory response develops slowly, and may not completely offset the increased rate of release because pituitary stores of the hormone are severely depleted by a strong, sustained secretory stimulus such as prolonged water deprivation.

The gene encoding the vasopressin–neurophysin precursor in humans is located distally on the short arm of chromosome 20 (20p13). It contains three exons which code, respectively, for: (1) the signal peptide, vasopressin, a dipeptide link, and the N-terminal variable portion of neurophysin; (2) the middle, highly-conserved part of neurophysin; and (3) the C-terminal, variable portion of neurophysin, a peptide link and the glycopeptide, copeptin ( Figure 42.3 ). The 5′ untranslated region upstream of the transcription start site also has many conserved sequences, suggesting a role in regulation of gene expression. Dehydration or osmotic stimulation increases expression of the vasopressin gene in the supraoptic nucleus, whereas adrenal insufficiency or febrile stress does so in parts of the paraventricular nucleus. In contrast, sustained hypoosmolarity inhibits expression. The vasopressin genes in rats, cows, and mice are similar to those in humans, but have several substitutions at either end of the highly-conserved central portion of the neurophysin moiety.
The oxytocin gene is closely linked to the vasopressin gene on chromosome 20 with an intergenic region of only 11 kilobases. However, the two genes are transcribed from opposite DNA strands, implying a tail-to-tail orientation, differ significantly in putative regulatory regions, and are expressed in mutually-exclusive sets of neurons. The oxytocin gene also differs significantly from the vasopressin gene, in that it does not contain a copeptin encoding sequence in exon 3. Expression of the oxytocin gene in brain and some peripheral tissues is promoted by gonadal steroids.
Secretion
Vasopressin- and oxytocin-containing secretory granules are released from the terminals of magnocellular neurohypophysial neurons by a process of calcium-mediated exocytosis that is triggered by action potentials generated in the cell bodies of the supraoptic or paraventricular nucleus. Many different neurotransmitters or neuromodulators including, possibly, vasopressin itself, can stimulate or inhibit this activity. However, it is not yet known which, if any, of them are important in the regulation of secretion in health or disease. Secretory activity may also be influenced by the many glial cells in close proximity to the dendrites of the vasopressin cell bodies in the supraoptic nucleus.
Vasopressin as well as the neurophysin and copeptin moieties with which it is associated are released into capillaries scattered throughout all levels of the posterior pituitary. From there, they travel via the cavernous sinus to the subclavian vein, and thence to the inferior vena cava, heart, and general circulation. Neurophysin, which binds vasopressin in magnocellular neurons, dissociates from the hormone at the concentrations and pH present in plasma. Binding of vasopressin to other plasma proteins has not been demonstrated. However, it does attach to platelets. It is unclear whether or not the platelet-bound vasopressin represents a store of the hormone in equilibrium with free plasma vasopressin. However, the bound and unbound fractions of vasopressin do not appear to track together, at least during acute changes in secretion. Oxytocin is also co-secreted with its neurophysin.
Assay
The measurement of vasopressin at physiologic levels in plasma presents an unusual combination of concerns that must be addressed with exceptional care. One problem is the large amount of hormone bound to platelets (see above). Because of it, vasopressin must be assayed not in serum, but in plasma that has been harvested very carefully to avoid contamination with platelets or fragments thereof.
A second problem is the fastidious requirements of the vasopressin assay itself. It must be unusually sensitive, because the hormone normally circulates and acts at very low concentrations. In healthy, normally hydrated, recumbent humans, the plasma concentration of “free” vasopressin is usually between 0.5 and 2.5 pg/mL (~10 −12 M), an amount sufficient to concentrate the urine ( Figure 42.4a ). Basal levels in other mammals are similar. The assay must also be very specific, because plasma also contains oxytocin, a nonapeptide that is structurally similar to vasopressin but has different biologic effects. The only practical method now available that can meet both requirements is radioimmunoassay. However, it too presents unusual problems, because vasopressin is relatively small and weakly antigenic. To complicate matters, most, if not all, the vasopressin antisera generated to date are susceptible to non-specific interference by one or more other unidentified components of plasma. Moreover, the characteristics and abundance of this interference can vary with the antiserum and type of anticoagulant used to prevent the blood from clotting. Consequently, vasopressin must be extracted from plasma, even if the antiserum employed is sensitive enough to detect the hormone at physiologic levels in unconcentrated extracts. Unfortunately, there is no one extraction method that is suitable for all assays. Therefore, the extraction method, as well as the anticoagulant, must be selected and tailored for the particular antiserum being used.

A third problem for vasopressin assays is the lack of a universal reference standard. Judging from the advertised biologic potencies, those available vary by as much as two-fold in purity, and unknown values reported in weight usually are not corrected for these differences. Therefore, unless the purity or potency of the standards is specified, it is impossible to compare absolute values reported by different laboratories unless they use the same standard, antiserum, and extraction technique.
The radioimmunoassay of vasopressin in urine would appear to be much easier than plasma, because the concentrations in urine are usually much higher, and interference by other substances is much less. However, urine samples must usually be diluted or concentrated to a constant solute concentration (preferably hypotonic), because some antisera are affected by high concentrations of salt or urea. Also, to adjust for the effect of changes in antidiuresis per se , the total amount of vasopressin in a urine sample must be expressed as a function of the length of time over which the sample was collected or of the amount of a solute such as creatinine that is excreted at a relatively constant rate. Finally, although changes in urinary excretion of vasopressin usually parallel the changes in plasma, this relationship can be distorted by changes in glomerular filtration or solute clearance.
Regulation
Osmotic
The most important determinant of vasopressin secretion under physiologic conditions is the “tonicity” or effective osmotic pressure of body water. This influence is mediated by a group of cells known collectively as osmoreceptors, that are located near but separate from the supraoptic nucleus, in or near a part of the anterior hypothalamus known as the organum vasculosum lamina terminalis ( Figure 42.2 ). Unlike the neurohypophysis, which receives its blood supply from a branch of the internal carotic artery, the area containing the osmoreceptors is supplied by small perforating branches of the anterior cerebral or communicating arteries. Thus, the interruption of these perforators (or damage to the anterior hypothalamus by other diseases or experimental lesions) can eliminate the osmoregulation of vasopressin secretion, without affecting the neurohypophysis or its response to hemodynamic or other nonosmotic stimuli. This dissociation indicates that the osmoregulation of vasopressin is mediated not by the magnocellular neurons that make the hormone, but by afferent inputs from osmosensitive cells located in other, anatomically discrete areas of the anterior hypothalamus. It is not yet known exactly how many such areas there are, whether they contain inhibitory as well as stimulatory elements, how they integrate with other regulatory afferents or how they communicate with vasopressin-producing neurons. Therefore, it may be more appropriate to refer to them as an osmoregulatory system.
The functional properties of the system that osmoregulates vasopressin resemble those of a discontinuous or “set-point” receptor ( Figure 42.4b ). Thus, in healthy adults and children, plasma vasopressin is very low or undetectable (<0.5 pg/mL) when plasma osmolarity is below a certain minimum or threshold level. Above that “set-point,” plasma vasopressin increases steeply in direct proportion with the increase in plasma osmolality. The slope of the line describing this relationship indicates that, on average, a rise in plasma osmolality of 1% increases plasma vasopressin by 1 pg/mL, an amount sufficient to significantly alter urinary concentration and flow ( Figure 42.4 ).
The functional properties of the osmoregulatory system are similar in men and non-pregnant women, as well as in blacks and whites. Within each group, however, they vary considerably from person to person. Individual differences in the slope or sensitivity of the response are particularly large, varying as much as 10-fold. Differences in the apparent threshold or set-point are smaller, but still range from 274 to 293 mosmoles/L. These individual differences are constant over time, and appear to be determined largely by genetic factors. However, neither the sensitivity or the set-point is totally immutable, because they can be altered by a variety of conditions, drugs, and hormones (see below).
It is uncertain whether the threshold concept accurately represents the operation of the osmoreceptor at its most fundamental level. The relation of plasma vasopressin to plasma osmolarity in the population as a whole appears to be slightly curvilinear ( Figure 42.4 ). This curvilinearity could be due simply to individual variation, since there as a positive correlation between slope and threshold, i.e., the higher the threshold the steeper the slope. It is also possible, however, that the stimulation of vasopressin secretion is self-amplifying. At present, however, the precision and reproducibility of the vasopressin assays at the low levels present under basal conditions are insufficient to determine if the best fit of the relationship is linear or exponential. It is also possible that vasopressin secretion reflects the balance of inhibitory as well as stimulatory inputs from a bimodal osmoregulatory system, because patients and animals with adipsic hypernatremia due to destruction of the osmoreceptors may lose the capacity to osmotically suppress, as well as to stimulate, vasopressin secretion. As a practical matter, however, the concept of an osmotic threshold remains, for the present, a valid and useful way of describing many aspects of normal and abnormal osmoregulatory function in the intact animal.
It is also uncertain whether vasopressin is secreted continuously or episodically in response to osmotic stimulation. When nonosmotic stimuli such as posture, activity, and blood pressure are controlled, infusion of hypertonic saline in humans almost always produces a smooth progressive increase in systemic venous plasma vasopressin that correlates very closely with the increase in plasma osmolality. However, samples obtained from experimental animals nearer the source of the hormone, for example, from the internal jugular vein, exhibit large fluctuations in plasma vasopressin during osmotic stimulation. Whether these fluctuations reflect an intrinsic property of the neurohypophysis or the osmoreceptors, or are artifacts of the experimental conditions is unknown. Irregular phasic firing of the neurosecretory neurons has been observed by unit recording techniques during stimulation, but this activity is unlikely to be related to episodic fluctuations in plasma vasopressin because the discharge cycles have a much shorter periodicity and are not synchronized from cell to cell.
The system that osmoregulates vasopressin secretion is not equally sensitive to all the solutes in plasma and extracellular fluid. The most potent stimuli are sodium and its anions, the solutes that ordinarily account for more than 95% of measured plasma osmolarity. However, certain sugars, such as mannitol and sucrose, are also very effective when infused intravenously. In fact, particle-for-particle, mannitol is as potent as sodium chloride. In contrast, an increase in plasma osmolarity produced by infusion of urea or glucose causes little or no increase in plasma vasopressin in healthy adults. Thus, the concentration of sodium and its anions is the principal, if not the only, determinant of osmotically-mediated vasopressin secretion under normal conditions. The basic mechanism by which the osmoregulatory system senses changes in the plasma concentration of sodium and its anions has not been completely established. On the basis of studies in dogs, Verney proposed that the osmoreceptor is stimulated when its intracellular volume is reduced by an osmotically driven efflux of water. If so, solutes that enter the osmoreceptor cells slowly or not at all would be “effective” stimuli, whereas those that penetrate rapidly would have little or no effect. This theory is consistent with the observations that vasopressin secretion is stimulated similarly by infusing hypertonic saline or mannitol, both of which are excluded from cells. In addition, vasopressin is not stimulated by a rise in plasma osmolarity produced by rapid infusion of hypertonic glucose, a solute which enters cells rapidly in the presence of insulin. If anything, the hyperglycemic hyperosmolarity produced by infusion of hypertonic glucose suppresses plasma vasopressin, probably because it also lowers the plasma concentration of sodium salts by inducing an osmotically driven efflux of water from the intracellular to the extracellular compartment. This disparity suggests that, in the presence of insulin, glucose enters osmoreceptor cells even more rapidly than many other cells of the body.
Another theory posits that the osmoreceptors are actually sodium receptors located on the brain side of the blood–brain barrier. It is consistent with the observed effects of hypertonic mannitol infusion, because this solute does not cross the blood–brain barrier and, as a consequence, causes an osmotically driven efflux of water from brain that probably raises the extracellular concentration of sodium in that organ. However, the sodium receptor theory is not consistent with the relatively small rise in plasma vasopressin produced by an acute rise in plasma urea, because this solute also crosses the blood–brain barrier slowly and, as a consequence, reduces brain water and raises brain extracellular sodium concentration much like mannitol. This singular disparity indicates that most, if not all, of the osmoreceptors are probably located outside the blood–brain barrier, and that another factor, most likely the solute permeability of the osmoreceptor cell itself, determines the solute-specificity of the system. This concept is also consistent with two other observations: (1) the osmoregulatory system appears to be located in or near the organum vasculosum lamina terminalis, a region of the anterior hypothalamus that is known to lack a blood–brain barrier; and (2) a severe deficiency of insulin sensitizes the osmoreceptors to stimulation by hyperglycemia, presumably by decreasing its permeability to glucose. It is not yet known, however, what distinguishes the cells of the osmoregulatory system from all the others whose volume and solute concentrations are similarly affected by changes in the extracellular concentration of sodium and its anions.
Non-Osmotic
Hemodynamic
An acute decrease of blood pressure or blood volume increases plasma vasopressin by an amount that is roughly proportional to the degree of hypotension or hypovolemia. However, the stimulus–response relationship follows a distinctly exponential pattern ( Figure 42.5 ). Small decreases in blood pressure or volume of 5 to 10% usually have little or no perceptible effect on plasma vasopressin, whereas decreases of 20 to 30% result in plasma hormone levels many times those required to produce maximum antidiuresis. Acute orthostasis, which decreases “effective” blood volume by 10 to 15%, usually doubles plasma vasopressin. In rats, chronic or sustained hypovolemia of 24 to 48 hours duration has even less effect on vasopressin, even though pituitary stores of the hormone are undiminished. This suggests that chronic stimulation selectively desensitizes the volume control mechanism.

The effects of acute hypovolemia on vasopressin secretion appear to be mediated largely by neuronal afferents that arise in pressure-sensitive receptors in the left side of the heart and project by way of the vagus and glossopharyngeal nerves to primary synapses on the nuclei of the solitary tracts in the medulla. From there, signals ascend to the hypothalamus via postsynaptic pathways that appear to be mediated in part by a highly selective opioid neurotransmitter of the kappa subclass in the lateral parabrachial nucleus. The vasopressin response to acute hypotension is mediated by neurogenic afferents that arise in high-pressure receptors in the aorta and carotid sinus, and project via the vagus and glossopharyngeal nerves, presumably, to the nuclei of the solitary tracts. From there, pathways that are at least partly noradrenergic ascend to the hypothalamus. At least for part of their length, these ascending pathways are separate from those that mediate the vasopressin response to hypovolemia, because they are not blocked by opioid antagonists that abolish the response to hypovolemia.
Acute hypervolemia or hypertension can inhibit vasopressin secretion. However, the increase in blood volume or pressure required is relatively large, and the inhibition of AVP is slight. The pathways that mediate the hemodynamic inhibition have not been fully-elucidated, and they may be the same as those that mediate stimulation. Interruption of primary vagal afferents eliminates the effect of hemodynamic stimuli, but also results in an acute rise in basal vasopressin secretion. This suggests that the baroregulatory afferents tonically inhibit vasopressin secretion under basal, normovolemic, and normotensive conditions. If so, the slight decrease in vasopressin produced by acute hypervolemia and hypertension may be simply enhancement of this tonic inhibitory effect. Likewise, the increase in vasopressin produced by acute hypovolemia or hypotension could result from a reduction in basal inhibition of secretion. The effect of a chronic increase in blood volume or pressure is largely undefined. The osmoregulation of vasopressin secretion appears to be normal in patients with uncomplicated essential hypertension, but may be inhibited slightly in primary hyperaldosteronism, suggesting that chronic expansion of the blood volume has an effect opposite to that of hypovolemia.
Changes in blood volume or pressure large enough to affect vasopressin secretion do not interfere with osmoregulation of the hormone. Instead, they appear to act by shifting the set of the system in such a way as to increase or decrease the effect on vasopressin of a given osmotic stimulus. This means that the osmoregulatory and baroregulatory systems, although different in location and function, ultimately converge and act upon the same population of neurosecretory neurons ( Figure 42.2 ). Exactly how and where this integration occurs is unknown.
Emetic
Nausea is an extremely potent stimulus for vasopressin secretion in humans. The pathways that mediate this effect have not been defined, but they probably involve the chemoreceptor trigger zone in the area postrema of the medulla. The effect on vasopressin is instantaneous and extremely potent. Increases of 100 to 500 times basal levels are not unusual, even when the nausea is transient and unaccompanied by vomiting or changes in blood pressure. Pretreatment with fluphenazine, haloperidol or promethazine in doses sufficient to prevent nausea completely abolish the vasopressin response. The inhibitory effect of these dopamine antagonists is specific for emetic stimuli, because they do not alter the vasopressin response to osmotic or hemodynamic stimuli. Water-loading blunts, but does not abolish, the effect of nausea on vasopressin release, suggesting that osmotic and emetic influences interact in a manner similar to osmotic and hemodynamic pathways. The effect of emetic stimuli is also species-dependent. Whereas emetics such as apomorphine, cholecystokinin, lithium chloride, and copper sulfate stimulate the secretion of vasopressin but not oxytocin in humans and monkeys, they increase the secretion of oxytocin but have little effect on vasopressin in rodents.
Emetic stimuli probably mediate many pharmacologic and pathologic effects on vasopressin secretion. These include not only apomorphine, but also high doses of morphine, nicotine, alcohol, cholecystokinin, and motion sickness. They may also be responsible, at least in part, for the increases in vasopressin secretion that have been observed with intravenous cyclophosphamide, acute hypoxia, diabetic ketoacidosis, vasovagal syncope, and hyperemesis gravidarum. Because nausea and vomiting are frequent side-effects of many other drugs and diseases, additional examples of nausea-induced vasopressin secretion doubtlessly will be observed. The potency and ubiquity of emetic stimuli create special problems for research studies of vasopressin secretion in animals and unconscious subjects, because the occurrence of nausea is difficult to ascertain except by verbal report.
Other Influences
Glucopenia
Acute insulin-induced hypoglycemia also stimulates vasopressin release in proportion to the decrease in plasma glucose. The rate of decrease in glucose is probably the critical determinant, however, because the increase in plasma vasopressin is not sustained even when the hypoglycemia persists. The receptor and pathway that mediate this effect are unknown. They are probably separate from those that mediate the effects of other recognized stimuli, because hypoglycemia stimulates vasopressin secretion in patients who have selectively lost the capacity to respond to osmotic, hemodynamic or emetic stimuli. However, the vasopressin response is accentuated by dehydration and abolished by water-loading, indicating that hypoglycemic stimuli probably act in concert with osmotic influences even though the osmoreceptors per se are unnecessary for the response. The effect of hypoglycemia is not due to nonspecific stress, because it can occur in the absence of symptoms and is more pronounced in rats, a species in which vasopressin secretion appears to be unaffected by pain and other noxious stimuli. The variable that actually triggers the release of vasopressin may be an intracellular deficiency of glucose or one of its metabolites, because 2-deoxyglucose is also an effective stimulus.
Angiotensin
The renin–angiotensin system has also been implicated in the control of vasopressin secretion. The precise site and mechanism of action have not been defined, but one or more central receptors seem likely, because angiotensin II is most effective when injected directly into brain ventricles or cranial arteries. The magnitude of the vasopressin response may depend on the concurrent osmotic stimulus. This dependency may account for the failure of some investigators to demonstrate stimulation by peripherally administered angiotensin. However, if angiotensin is directly involved in the physiologic control of vasopressin, it is more likely that produced by the renin–angiotensin system of the brain.
Stress
Nonspecific stress caused by pain, emotion or physical exercise has long been thought to release vasopressin. However, it has never been determined whether this effect is mediated by a specific pathway or is secondary to other stress-induced stimuli, such as the severe hypotension and/or nausea that often accompanys the vasovagal reaction to pain or fear. In rats and humans, stresses severe enough to activate the pituitary adrenal axis and sympathetic nervous system do not stimulate an increase in plasma vasopressin unless they also lower blood pressure or alter blood volume. If anything, stress seems to transiently suppress plasma vasopressin, possibly as a consequence of an acute rise in blood pressure or activation of other inhibitory input. However, stresses of various types have been found to stimulate the release of vasopressin (as well as corticotropin releasing hormone) from parvocellular neurons which project to portal veins of the anterior pituitary and appear to play a role in the regulation of ACTH secretion.
Temperature
Environmental temperature can also influence plasma vasopressin. In healthy adults, exposure to cold for a relatively short period of time depresses plasma vasopressin, and heat has the opposite effect. These changes are independent of changes in plasma osmolality, but they cannot as yet be divorced from changes in effective blood volume or blood pressure. Experimentally-induced hypothermia or fever in animals is also associated with changes in plasma vasopressin, but the effects seem to vary, possibly as a function of the species, the dose of endotoxin administered or the time interval. Thus, it is still uncertain how exogenous or endogenous changes in temperature effect vasopressin secretion by magnocellular neurons.
Oropharynx
Drinking can also inhibit vasopressin secretion, even before it produces a detectable decrease in plasma osmolarity or sodium. This effect does not depend on the water reaching the stomach, and is unrelated to changes in blood pressure or blood volume. It may depend on the volume or temperature of the fluid ingested because, in humans at least, small volumes of water (100 mL) at room temperature are less inhibitory than larger volumes (700–1200 mL) or small amounts of ice. Thus, the inhibition may be mediated by neural afferents that originate in taste, temperature or other sensory receptors in the oropharynx. Inhibition of vasopressin by oropharyngeal receptors is rapid and transient. This may explain why it is not associated with a concurrent decrease in urine concentration. The rapidity of the decrease is also unexplained, since it appears to exceed the rate at which vasopressin is cleared from plasma under steady-state conditions (see below).
Age
Normal aging also appears to alter the osmoregulation of vasopressin secretion in humans, but the nature of the change is controversial. Some find that the vasopressin response to an osmotic stimulus is enhanced, but others report no change or a decreased response. These differences are unexplained, and may represent individual variation in the impact of aging on various components of the osmoregulatory and other interacting control systems. Histologic studies of the human brain show variable changes in the suprachiasmitic nucleus and other vasopressin-containing parvocellular pathways, but no decrease in the number of large cell bodies in the supraoptic and paraventricular nucleus. MRI studies indicate that the posterior pituitary bright spot is diminished or absent more often in the elderly than in the young, but this decrease can be due either to atrophy of the gland or to more rapid turnover of vasopressin due, for example, to decreased fluid intake or decreased renal effect of the hormone. Aging does not seem to impair the ability to osmotically suppress vasopressin secretion and dilute the urine, but it does diminish the capacity to excrete a water-load, because of the large age-related reduction in glomerular filtration rate. The capacity to maximally concentrate the urine also diminishes with age, but this may also be due largely to renal factors.
Gender, Pregnancy, the Menstrual Cycle, and Gonadal Hormones
The effects of gender on the osmoregulation of vasopressin secretion are complex and unsettled. There appears to be little or no difference between adult human males and non-gravid females, but pregnancy results in a relatively large reduction in the osmotic threshold for vasopressin release in rats as well as in humans. Downward resetting of the osmostat also occurs during the luteal phase of the human menstrual cycle, but the shift is small relative to that in pregnancy and is smaller even than the range of individual variation in men and non-gravid women. The cause of the resetting is uncertain, but it may be mediated by relaxin, an increase in estrogens or chorionic gonadotropin, since they also lower the set of the osmostat in humans. Progesterone appears to have no effect. In rats, large doses of estrogen seem to have a different effect than in humans, since they enhance the slope or sensitivity of the vasopressin response to plasma osmolality, but do not significantly alter its set-point.
Drugs and Other Hormones
Many drugs and hormones also influence vasopressin secretion ( Table 42.1 ). Those that stimulate, such as histamine, bradykinin, prostaglandin, β-endorphin, and high doses of morphine, have not been studied sufficiently to define their mechanisms of action, but may act by decreasing blood pressure or producing nausea. Vincristine may have a direct toxic effect on the neurohypophysis or peripheral neurons involved in the regulation of vasopressin secretion. Lithium, which antagonizes the antidiuretic effect of vasopressin, also increases secretion of the hormone. This effect is independent of changes in water balance, and appears to result from an increase in sensitivity of the osmoregulatory system. The stimulatory effects of chlorpropamide and clofibrate are still controversial, and a mechanism of action has not been proposed.
Stimulatory | Inhibitory |
---|---|
Acetycholine | Fluphenazine |
Morphine (high doses) | Haloperidol |
Epinephrine | Promethazine |
Histamine | Oxilorphan |
Bradykinin | Butorphanol |
Prostaglandins | Opiods (κ and δ agonists) |
β-Endorphin | Morphine (low doses) |
Cyclophosphamide | Alcohol |
Vincristine | Carbamazepine |
Lithium | ? Glucocorticoids |
? Chlorpropamide | ? Phenytoin |
? Clofibrate | |
? Corticotropin-releasing factor |
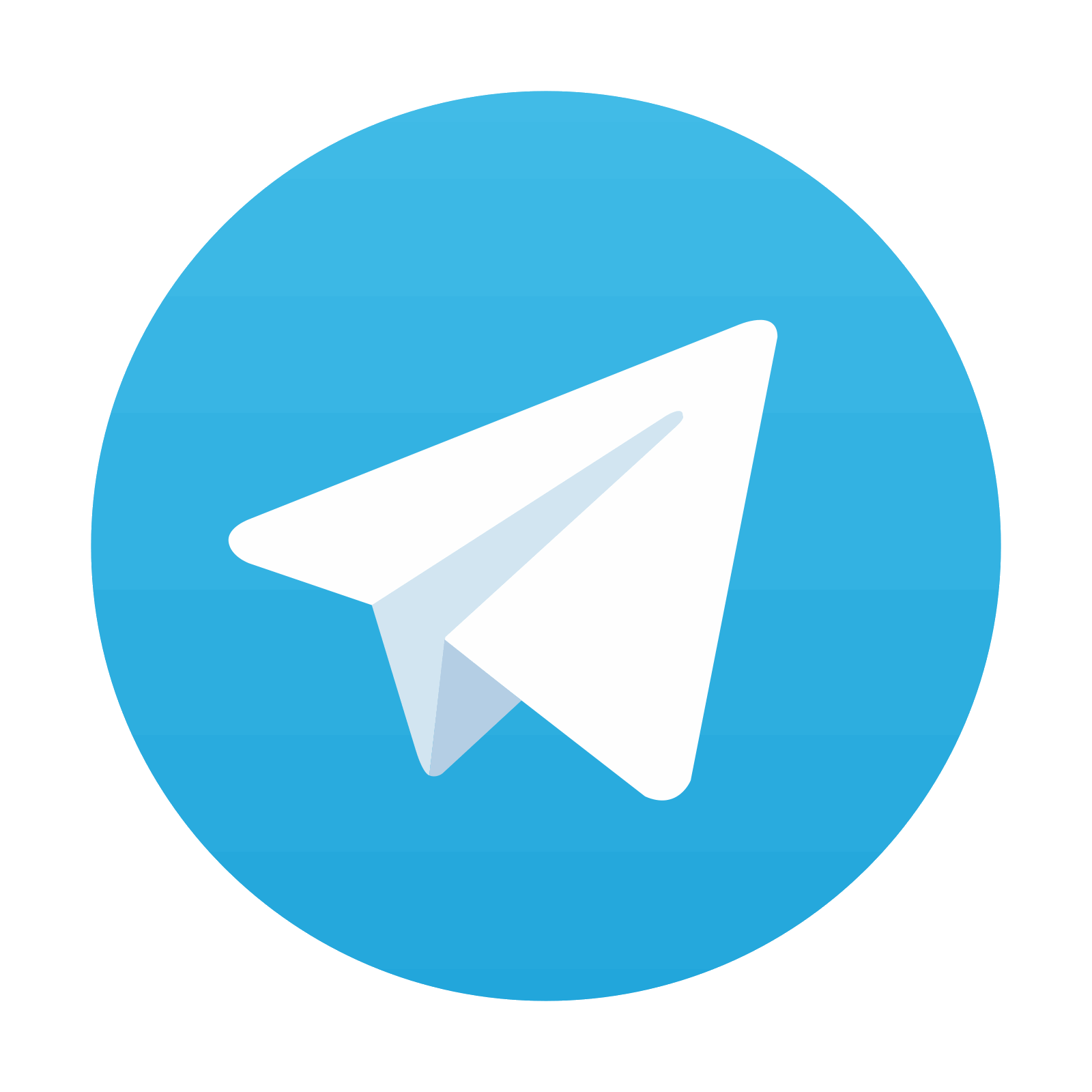
Stay updated, free articles. Join our Telegram channel
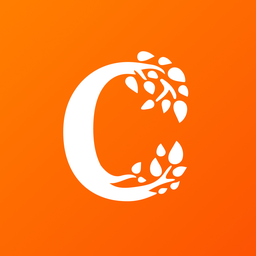
Full access? Get Clinical Tree
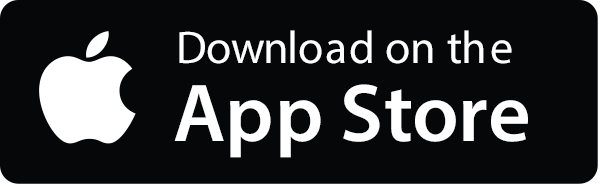
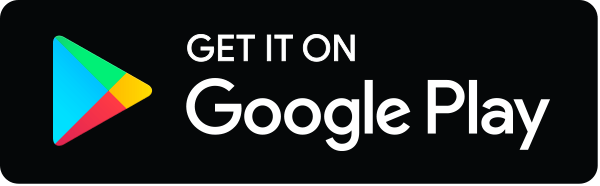
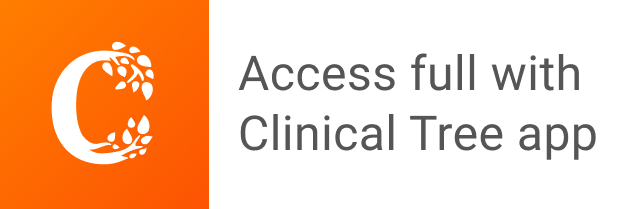