Fig. 18.1
Glomerular capillary wall showing thickening and widening (arrows) with basement membrane splitting and lamellations (basket-weave appearance). Note extensive foot process effacement. (x6000). Courtesy Dr. Sanjeev Sethi, Mayo Clinic
COL4A5 mutations; they may be offspring of asymptomatic female carriers, or may have autosomal recessive disease.
Ultrastructural alterations of the GBM were noted in several reports of hereditary nephritis in the 1960s and 1970s [1, 32–36]. The most prominent abnormality is the irregular appearance of the lamina densa, which demonstrates zones of thickening, thinning, and splitting that may be interposed with segments of GBM that appear normal. The abnormalities can affect a portion of a capillary loop, an entire loop, and some or all loops and can even appear to spare individual glomeruli.
The thick segments measure up to 1,200 nm, usually have irregular outer and inner contours, and are found more commonly in males than in females. The lamina densa is transformed into a heterogeneous network of membranous strands, which enclose clear electron-lucent areas. The electron-lucent zones may be entirely clear or contain round granules of variable density that measure between 20 and 90 nm in diameter. The origin of these granules is unknown, but Rumpelt has hypothesized that they represent degenerating islands of visceral epithelial cell cytoplasm [37]. Young children and females, and adult males on occasion [38], may have diffusely attenuated segments of GBM measuring as little as 100 nm or less; in some instances, discontinuities of the GBM have been observed [2, 39].
The specificity of the GBM changes has been questioned [40]. Focal lamina densa splitting and lamellation may be observed in renal biopsies from patients with a variety of glomerular disorders. Clinical correlation and immunofluorescence microscopic examination of all biopsies are necessary when AS is the suspected diagnosis. Diffuse thickening and splitting of the GBM are strongly suggestive of AS. In the absence of immune deposits, these changes are pathognomonic. Occasional patients have an immune complex nephritis such as IgA nephropathy superimposed on AS [41].
Not all Alport kindreds demonstrate these characteristic ultrastructural features [42–44]. Thick, thin, normal, and nonspecifically altered GBMs have all been described. Although diffuse attenuation of GBM has been considered the hallmark of “benign familial hematuria” or thin basement membrane nephropathy (TBMN) [45–47], some patients with this abnormality are members of kindreds with a history of progression to renal failure [48, 49]. Thus, the significance of an ultrastructural finding of thin GBMs must be considered in the context of the family history, basement membrane expression of collagen IV collagen α(alpha) chains (see below), and genetic information.
Rumpelt described a correlation between the percent of GBM showing splitting of the lamina densa and the degree of proteinuria in AS patients [37], suggesting that impaired permselectivity may be a functional consequence of the GBM alteration. Kim and colleagues observed that morphometric parameters such as mesangial volume fraction, cortical interstitial volume fraction, and percent global glomerular sclerosis were inversely correlated with creatinine clearance in AS, while creatinine clearance and surface density of peripheral GBM were directly correlated [50]. Similar relationships have been observed in other diseases, such as diabetic nephropathy and membranoproliferative glomerulonephritis (MPGN). However, patients with AS showed significantly greater impairment of filtration for any degree of structural change, in comparison with patients with diabetic nephropathy or MPGN. This observation suggests that these morphologic abnormalities only partially account for reductions in creatinine clearance in AS. Decreased conductivity of water across the altered glomerular capillary wall could contribute to the decrement in filtration.
Kidney biopsies of patients with advanced AS show nonspecific changes such as glomerulosclerosis, tubular atrophy, and interstitial fibrosis, potentially obscuring the diagnosis. Collagen IV immunohistochemistry and molecular genetic studies are often diagnostic in these ambiguous cases, as they are in patients with GBM thinning without characteristic lamina densa splitting.
Genetics and Pathophysiology
Genetics and Biochemistry of Collagen IV
The GBM is composed of several major constituents, including collagen IV, laminin, nidogen, and heparan sulfate proteoglycan. The collagen IV family of proteins comprises six isomeric chains, designated α(alpha)1 to α(alpha)6(IV) [51, 52]. These chains show extensive sequence homology and share basic structural features, including (1) a major collagenous domain of about 1,400 residues containing the repetitive triplet sequence glycine (Gly)-X-Y, in which X and Y represent a variety of other amino acids; (2) a carboxyterminal noncollagenous (NC1) domain of about 230 residues; and (3) a noncollagenous aminoterminal sequence of 15–20 residues. The major collagenous domain of each chain contains about 20 interruptions of the collagenous triplet sequence, while each noncollagenous domain contains 12 completely conserved cysteine residues that form critical disulfide bonds.
Each collagen IV molecule is a trimer composed of three α(alpha) chains. Collagen IV α(alpha) chains form trimers through associations between their carboxyterminal NC1 domains, accompanied by folding of the collagenous domains into triple helices [53–55]. Variable residues within the NC1 domains confer specificity upon chain-chain associations [56]. Collagen IV triple helices form networks through several types of intermolecular interaction: end-to-end linkages between the carboxyterminal domains of two collagen IV triple helices, covalent interactions between four triple helices at their aminoterminal ends, and lateral associations mediated by binding of the carboxyterminal domains of one trimer to the collagenous region of another trimer [57, 58]. Disulfide bonds formed by conserved cysteine residues are critical to the interactions between NC1 domains. These linkages between collagen IV molecules produce a nonfibrillar, open-network assembly that associates with laminin through interactions mediated by nidogen [59].
Various integrins mediate cell attachment to collagen IV. Attachment of glomerular epithelial cells to the α(alpha)3(IV) chain appears to involve the α(alpha)3β(beta)1 integrin [60].
The six collagen IV genes are arranged in pairs on three different chromosomes. The human α(alpha)1 and α(alpha)2(IV) chains are encoded by the genes COL4A1 and COL4A2, respectively, on chromosome 13 [61]. COL4A3 and COL4A4 are located on chromosome 2 and encode the α(alpha)3(IV) and α(alpha)4(IV) chains of type IV collagen, respectively [62], while the α(alpha)5(IV) and α(alpha)6(IV) chains are encoded by the COL4A5 and COL4A6 genes on the X chromosome [63–65]. The 5′ ends of each gene pair are adjacent to each other, separated by sequences of varying length containing motifs involved in the regulation of transcriptional activity [66–68].
Evidence from a variety of sources indicates the existence of at least three collagen IV networks in mammalian basement membranes, and studies of the distribution of these networks in basement membranes using monospecific antibodies have revealed a high degree of tissue specificity. A network composed of heterotrimers with the composition α(alpha)1(IV)2α(alpha)2(IV) is found in all basement membranes, although it is a relatively minor component of mature GBM. A network comprising α(alpha)3(IV)-α(alpha)4(IV)-α(alpha)5(IV) heterotrimers is the predominant collagen in mature GBM and also occurs in Bowman’s capsules and the basement membranes of distal and collecting tubules. A hybrid network consisting of α(alpha)1(IV)2α(alpha)2(IV) and α(alpha)5(IV)2α(alpha)6(IV) heterotrimers is present in Bowman’s capsule and distal and collecting tubule basement membranes, but is not present in GBM. The epidermal basement membrane and basement membranes surrounding aortic smooth muscles cells contain α(alpha)1(IV)2α(alpha)2(IV)/α(alpha)5(IV)2α(alpha)6(IV) networks, but not the α(alpha)3(IV)-α(alpha)4(IV)-α(alpha)5(IV) network[69, 70].
Abnormalities of Collagen IV Genes and Proteins in Alport Syndrome
There are three genetic forms of AS [75]. The X-linked form (XLAS), resulting from mutations in COL4A5, accounts for about 80 % of patients with the disease. About 15 % of patients have autosomal recessive Alport syndrome (ARAS), which arises from mutations affecting both alleles of COL4A3 or COL4A4. The heterozygous parents of children with ARAS often have asymptomatic hematuria, although some have normal urinalyses. Finally, approximately 5 % of patients have autosomal dominant Alport syndrome (ADAS), due to heterozygous mutations in COL4A3 or COL4A4. Heterozygous mutations in COL4A3 or COL4A4 have also been found in families with TBMN (see below). It is not yet clear why many, perhaps most, individuals with heterozygous mutations in these genes have asymptomatic hematuria, while others have progressive disease.
XLAS
Over 600 COL4A5 mutations have been found in XLAS families. These mutations are distributed throughout the gene, and with few exceptions each mutation is unique. In the USA, three missense mutations associated with relatively late onset of ESRD account for a disproportionate number of affected families [76, 77]. About 15 % of COL4A5 mutations are large rearrangements, predominantly deletions [75]. Missense mutations account for about 40 %, about 15 % are splice-site mutations, and 25–30 % are nonsense mutations or small frame-shifting deletions or insertions that result in premature stop codons [75]. About 10 to 15 % of COL4A5 mutations occur as spontaneous events in the proband, explaining why some patients with XLAS lack a family history of the disease.
The association of XLAS with leiomyomatosis of the esophagus and tracheobronchial tree has been reported in several dozen families [78]. Affected members of these families exhibit large deletions that span the adjacent 5′ ends of the COL4A5 and COL4A6 genes [79, 80]. These deletions involve varying lengths of COL4A5, but the COL4A6 breakpoint is located in the second intron of the gene [81–83]. Leiomyomatosis does not occur in patients with deletions of COL4A5 and COL4A6 that extend beyond intron 2 of COL4A6. Mutations of COL4A6 alone do not appear to cause Alport syndrome, consistent with the absence of the α(alpha)6(IV) chain from normal human GBM [84].
Two families have been described in which COL4A5 deletions that extend beyond the 3′ end of the gene cause XLAS, mental retardation, and midface hypoplasia [85, 86]. In one of these families, affected individuals also displayed elliptocytosis.
The great majority (about 85 %) of missense COL4A5 mutations are guanine substitutions in the first or second position of glycine codons that result in the replacement of a glycine residue in the collagenous domain of α(alpha)5(IV) by another amino acid [75]. Such mutations are thought to interfere with the normal folding of the mutant α(alpha)5(IV) chain into triple helices with other collagen IV α(alpha) chains [87]. Glycine lacks a side chain, making it the least bulky of amino acids and small enough to allow three glycine residues to fit into the interior of a tightly wound triple helix [88]. The presence of a bulkier amino acid in a glycine position presumably creates a kink or an unfolding in the triple helix. Glycine substitutions in the α(alpha)1 chain of type I collagen account for the majority of mutations causing osteogenesis imperfecta and are common in other genetic disorders of collagen [89, 90]. Abnormally folded collagen triple helices exhibit increased susceptibility to proteolytic degradation [89]. The position of the substituted glycine, or the substituting amino acid itself, may influence the effect of the mutation on triple helical folding and ultimately the impact of the mutation on the severity of the clinical phenotype [77, 91].
ARAS
To date, mutations causing ARAS have been found in the COL4A3 or COL4A4 gene in over 100 patients [75]. Some of these patients are homozygous for their mutations and others are compound heterozygotes. As with COL4A5, there appear to be no mutation hot spots in COL4A3 or COL4A4. Although it is possible that ARAS could result from the combination of a mutation in one allele of COL4A3 and a mutation in one allele of COL4A4, no such example has been described. It is worth noting that the mating of two individuals with asymptomatic hematuria due to heterozygous COL4A3 or COL4A4 mutations can result in a child who has mutations in both alleles of COL4A3 or COL4A4 and, as a result, ARAS.
The reported COL4A3 and COLA4 mutations in ARAS include nonsense (10–15 %), frameshift (about 25 %), splicing (about 15 %), and missense mutations (about 50 %) [75]. As in XLAS, the majority (about 80 %) of missense mutations produce a glycine substitution in the collagenous domain of α(alpha)3(IV) or α(alpha)4(IV).
ADAS
Heterozygous COL4A3 and COL4A4 mutations have been described in several families transmitting AS as an autosomal dominant disorder [92–94]. It is not clear why some individuals with heterozygous COL4A3 or COL4A4 mutations are asymptomatic or exhibit only isolated microhematuria while others have a progressive nephropathy. Several possibilities can be proposed: the type and/or site of the mutation may be critical, the presence of certain polymorphisms in these genes may influence the effect of a pathogenic mutation, or a polymorphism or mutation in another gene may modify the effect of the mutation. In some cases, a heterozygous missense mutation in COL4A3 or COL4A4 might be more detrimental than a deletion or nonsense mutation, because the mutant chain can then induce the degradation of normal chains with which it forms abnormal trimers.
Phenotype–Genotype Correlations in Alport Syndrome
Male patients with COL4A5 deletions consistently exhibit sensorineural deafness and progression to ESRD during the second or third decade of life [4]. Most of the missense, nonsense, and splicing COL4A5 mutations described thus far have also been associated with early progression to ESRD in the second or third decade of life and sensorineural deafness. Single amino acid substitutions that alter an mRNA splice site, leading to exon skipping, or small deletions or insertions that shift the transcriptional reading frame, can clearly produce such aberrant protein products that a severe phenotype results. Several COL4A5 mutations have been associated with late-onset (after the third decade) ESRD and deafness [76].
Large rearrangements, nonsense mutations, and frameshift mutations of the COL4A5 gene confer a 90 % probability of ESRD before age 30 years, with 50 % reaching ESRD by age 20 years [4, 77, 91]. Splice-site mutations are associated with a probability of ESRD before age 30 years of 70 %, with 50 % reaching ESRD by age 25 years. Patients with missense mutations have a 50 % probability of ESRD before age 30 years. Non-glycine missense mutations and glycine mutations in the 3′ portion of COL4A5 are associated with earlier development of ESRD, compared with glycine missense mutations in the 5′ portion of the gene [77, 91].
The severity of disease in a female heterozygous for a COL4A5 mutation is probably influenced to some extent by the nature of the mutation, but the extent of inactivation of the X chromosome carrying the normal COL4A5 allele is likely a more important factor. Jais et al. were unable to demonstrate a genotype effect on outcome in XLAS heterozygotes, presumably due to the stronger influence of X inactivation [3]. Guo et al. described a woman with a severe Alport phenotype (ESRD at age 30), one of whose COL4A5 alleles carried two missense mutations [95]. Analysis of DNA isolated from the patient’s kidney and leukocytes showed inactivation of greater than 90 % of the X chromosomes carrying the normal COL4A5 allele. Other investigators, however, have not been able to confirm that X-inactivation pattern of leukocytes predicts phenotype in XLAS heterozygotes [96]. In a mouse model of X-linked Alport syndrome [97], the relative activity of the X chromosome carrying the mutant col4a5 allele determines the outcome of heterozygous females [98].
Collagen IV in Alport Basement Membranes
Several studies in the 1980s established that the native kidneys of male AS patients failed to bind anti-GBM antibodies from patients with Goodpasture syndrome or from AS patients with posttransplant anti-GBM nephritis [102–106]. These early studies, combined with the observation by Hudson and colleagues that collagen IV was the target of Goodpasture antibodies [72], provided the first indication that AS might represent a primary disorder of type IV collagen. The availability of chain-specific antibodies has more recently allowed detailed investigation of the expression of the six collagen IV α(alpha) chains in AS basement membranes.
GBM, distal and collecting TBM, and Bowman’s capsules of males with XLAS usually lack expression of the α(alpha)3(IV), α(alpha)4(IV), and α(alpha)5(IV) chains but do express the α(alpha)1(IV) and α(alpha)2(IV) chains [107, 108]. The α(alpha)6(IV) chain is not expressed in Bowman’s capsule or distal TBM of XLAS males whose basement membranes lack α(alpha)5(IV) expression [109, 110]. Women who are heterozygous for XLAS mutations frequently exhibit mosaicism of GBM expression of the α(alpha)3(IV), α(alpha)4(IV), and α(alpha)5(IV) chains [108]. The epidermal basement membrane (EBM) normally expresses the α(alpha)1(IV), α(alpha)2(IV), α(alpha)5(IV), and α(alpha)6(IV) chains, but not the α(alpha)3(IV) or α(alpha)4(IV) chains [103, 109, 111]. Most males with XLAS show no EBM expression of α(alpha)5(IV) or α(alpha)6(IV), while female heterozygotes frequently display mosaicism [111]. Lens capsules of some males with XLAS do not express the α(alpha)3(IV), α(alpha)4(IV), α(alpha)5(IV), or α(alpha)6(IV) chains [112, 113].
In many patients with ARAS, GBMs show no expression of the α(alpha)3(IV), α(alpha)4(IV), or α(alpha)5(IV) chains, but α(alpha)5(IV) and α(alpha)6(IV) are expressed in Bowman’s capsule, distal TBM, and EBM [114]. Therefore, XLAS and ARAS may be distinguishable by immunohistochemical analysis of renal biopsy specimens.
It is important to note that immunostaining for collagen IV is normal in some patients with XLAS and ARAS. Consequently, normal expression of α(alpha)3(IV), α(alpha)4(IV), and α(alpha)5(IV) chains in basement membranes does not exclude a diagnosis of AS.
The abnormalities of collagen IV expression observed in AS indicate that a mutation affecting one of the chains involved in the α(alpha)3-α(alpha)4-α(alpha)5(IV) network can prevent GBM deposition of the entire network. Similarly, a mutation involving the α(alpha)5(IV) chain can interfere with basement membrane expression of the α(alpha)5(IV)-α(alpha)6(IV) network. Several lines of evidence suggest that the effects of a collagen IV α(alpha) chain mutation on the basement membrane expression of its partner chains are mediated by posttranslational events. Substitution of glycine residues in the α(alpha)1 chain of collagen I comprises the majority of mutations causing osteogenesis imperfecta [115]. These mutations impair the folding of α(alpha)1(I) chains with α(alpha)2(I) chains into trimers, and the resulting trimers exhibit enhanced susceptibility to extracellular proteolytic degradation [89]. By similar means, an α(alpha)5(IV) chain carrying a glycine substitution could bring about the destruction of normal α(alpha)3(IV) and α(alpha)4(IV) chains. Studies of mutations in the α(alpha)1 chain of collagen IV in C. elegans suggest another possible mechanism. Whether the α(alpha)1(IV) mutation results in a null allele (deletion or nonsense mutation) or a glycine substitution, the α(alpha)2(IV) chain accumulates intracellularly and never reaches the basement membrane [116]. Thus, mutations producing null COL4A5 alleles, or α(alpha)5(IV) proteins that cannot form trimers (due to alterations of the carboxyterminal domain), or some glycine substitutions may simply prevent secretion of α(alpha)3(IV) and α(alpha)4(IV) chains, which are eventually degraded. Results of in vitro studies support the hypothesis that α(alpha)5(IV) mutations impair the assembly of α(alpha)3-α(alpha)4-α(alpha)5(IV) heterotrimers [87].
Pathogenesis of Basement Membrane Lesions in Alport Syndrome
The tissue pathology of AS arises from abnormal basement membrane expression of the collagen IV network composed of α(alpha)3-α(alpha)4-α(alpha)5(IV) chains. This network is usually absent from or under-expressed in the basement membranes of AS patients, although in the basement membranes of some patients, the networks are present but defective in structure and function. Anterior lenticonus may represent the most straightforward demonstration of the consequences of the absence of this network from basement membranes. Absence or defective expression of these chains results in thinning and mechanical weakness of the lens capsule, interfering with its capacity to maintain the normal conformation of the lens [17, 112, 113]. Microhematuria, the earliest renal manifestation of AS, may likewise reflect GBM thinning and a tendency to develop focal ruptures due to absence or defective expression of the α(alpha)3-α(alpha)4-α(alpha)5(IV) network. The abnormal composition of AS GBM may increase its susceptibility to proteolysis, perhaps explaining episodes of gross hematuria precipitated by infections, which are not uncommon during the first two decades of life in AS patients [117]. On the other hand, the resolution of gross hematuria, which typically occurs by adolescence, may be a function of progressive GBM thickening [2, 5].
The relentless thickening and lamellation of the AS GBM feature the accumulation of extracellular matrix proteins that are normally absent from GBM or present in only small amounts. The α(alpha)1(IV)-α(alpha)2(IV) network is highly expressed in the basement membranes of primordial glomeruli but is normally replaced by the α(alpha)3-α(alpha)4-α(alpha)5(IV) network at the capillary loop stage of glomerular maturation [118]. In mature glomeruli the α(alpha)1(IV)-α(alpha)2(IV) network is confined to the mesangium and the subendothelial region of GBM [119]. This developmental switch fails to occur in AS glomeruli [117, 120]. Instead, the α(alpha)1(IV)-α(alpha)2(IV) network persists in mature AS glomeruli and accumulates, spreading from its normal subendothelial location to occupy the full width of GBM [107, 114]. Similarly, collagen V and collagen VI, which are normally found in the mesangium and in small quantities in the subendothelial GBM, are markedly overexpressed in AS GBM [107]. In addition, laminin chains that are normally confined to the mesangium are expressed in AS GBM [121, 122]. The molecular events that underlie these anomalies in protein expression may represent maladaptive responses to biomechanical events in glomeruli [123].
AS resembles other chronic glomerulopathies in that deterioration of glomerular filtration rate is closely correlated with fibrosis of the renal interstitium [124]. Measurable increases in cortical interstitial volume are unusual in males with XLAS before the age of 10, but progressive expansion of the interstitium is common during the second decade of life [124]. Renal tubular epithelial cell toxicity associated with reabsorption of filtered proteins may contribute to tubular injury [125]. At least some of the factors driving interstitial fibrosis in AS are nonspecific, such as increased TGF-β1 activity, monocytic infiltration, epithelial-mesenchymal transformation, and enhanced metalloproteinase expression [126–130]. This suggests that therapies that nonspecifically interfere with interstitial fibrosis may be of benefit to AS patients, without correcting the primary abnormalities of collagen IV expression [131, 132].
Diagnosis
AS should be considered in the differential diagnosis of patients with persistent microhematuria. Electron microscopic examination of renal tissue remains the most widely available and applied means for diagnosing AS. The presence of diffuse thickening and multilamellation of the GBM predicts a progressive nephropathy, regardless of family history. Unfortunately, ultrastructural information alone does not establish the mode of transmission in a particular family. In a patient with a negative family history, electron microscopy cannot distinguish de novo X-linked disease from autosomal recessive disease. In some patients the biopsy findings may be ambiguous, particularly females and young patients of either sex. Furthermore, rare families with progressive nephritis and COL4A5 mutations in association with thin GBMs have been described, indicating that the classic GBM lesion is not present in all AS kindreds.
In families with a firm diagnosis of AS, evaluation of individuals with newly recognized hematuria can be limited to ultrasound of the kidneys and urinary tract in most instances. In the absence of tumor or structural anomalies of the urinary tract, a diagnosis other than AS is unlikely.
When AS is suspected, confirmation of the diagnosis may be achieved through immunohistochemical methods and molecular genetic analysis. Monospecific antibodies directed against collagen IV α(alpha) chains are available making it possible to reliably evaluate renal basement membranes for expression of α(alpha)3(IV), α(alpha)4(IV), and α(alpha)5(IV) chains [133, 134]. Since the α(alpha)5(IV) chain is normally expressed in the epidermal basement membrane (EBM), examination of skin biopsies by immunofluorescence for expression of α(alpha)5(IV) is an additional tool for making a diagnosis of AS. Absence of the α(alpha)5(IV) chain from EBM is diagnostic of XLAS [135]. Given a male patient with a positive family history and clinical features characteristic of AS, examination of skin for α(alpha)5(IV) expression may obviate the necessity for kidney biopsy. However, a normal result does not exclude the diagnosis of AS, since in about 20 % of XLAS kindreds affected males express α(alpha)5(IV) in their renal and epidermal basement membranes. Females with XLAS frequently express α(alpha)5(IV) mosaically in the skin. While clearly mosaic expression of α(alpha)5(IV) is diagnostic of XLAS in a female, a normal result does not exclude the diagnosis.
Renal expression of type IV collagen α(alpha) chains can serve to confirm a diagnosis of AS and can in addition differentiate XLAS and ARAS. In most males with XLAS, renal basement membranes are devoid of the α(alpha)3, α(alpha)4, and α(alpha)5(IV) chains, while females frequently show mosaic expression of these chains. In most males and females with ARAS, the GBM, Bowman’s capsule, and distal TBM show no expression of the α(alpha)3(IV) and α(alpha)4(IV) chains, while α5(IV) is expressed in Bowman’s capsule and distal TBM but not GBM [114].
Genetic analysis provides the only means for reliably diagnosing the carrier state in asymptomatic female members of XLAS kindreds and for making a prenatal diagnosis of AS. There are also clinical situations in which a firm diagnosis of AS cannot be established or in which it is not possible to determine the mode of transmission, despite careful evaluation of the pedigree and application of the full range of histological methods. In these situations genetic analysis has the potential to provide information essential for determining prognosis and guiding genetic counseling. Genetic counseling based upon erroneous determination of the mode of inheritance may have unintended consequences [136].
Molecular genetic analysis of the COL4A3, COL4A4, and COL4A5 genes has become widely but not universally available, and the expense of such testing may limit access. Direct sequencing of these genes offers mutation detection rates of about 90 %. When clinical suspicion of XLAS is high, direct sequencing of COL4A5 can obviate the need for renal or skin biopsy. If clinical features and family history are not particularly suggestive of AS, kidney biopsy may be the most informative diagnostic study.
Treatment
Transplantation
Since AS does not recur in renal allografts, kidney transplantation corrects the AS nephropathy. Graft survival rates in patients with familial nephritis are comparable to those in patients with other diagnoses [137]. However, some AS patients develop anti-GBM nephritis in the renal allograft, usually resulting in graft loss. Data from several transplant centers indicates an incidence of 3–4 % in transplanted AS patients [138–140]. These patients are usually male, always deaf, and likely to have reached ESRD before the age of 30 [141]. This profile describes the majority of AS patients presenting for renal transplantation, so its predictive value is rather limited. However, AS patients with normal hearing or late progression to ESRD appear to be at very low risk for the development of posttransplant anti-GBM nephritis. Females with XLAS also appear to be in a low-risk category.
The onset of posttransplant anti-GBM nephritis was within the first year following transplantation in approximately 75 % of cases. Three-quarters of the allografts failed irreversibly, usually within a few weeks to months after diagnosis. Treatment with plasmapheresis and cyclophosphamide has been of limited benefit. Anti-GBM nephritis has recurred in at least 7 of 8 patients who underwent retransplantation. Posttransplant anti-GBM nephritis may recur despite an interval of many years between transplants and in the absence of detectable circulating anti-GBM antibodies prior to retransplantation.
The target(s) of anti-GBM antibodies in some of these patients has been determined, with variable results. Most patients with XLAS exhibit antibodies that target the carboxyterminal noncollagenous domain of the α(alpha)5(IV) chain, but anti-α(alpha)3(IV) antibodies have also been described [103, 138, 142–145]. Antibodies against α(alpha)3(IV) have been observed in ARAS patients with posttransplant anti-GBM nephritis [144].
It has been proposed that mutations in the COL4A5 gene that prevent expression of an immunogenic gene product, thereby preventing the establishment of tolerance for α(alpha)5(IV), might be associated with an increased risk for the development of posttransplant anti-GBM nephritis [146]. Of 14 AS patients with posttransplant anti-GBM nephritis examined for a COL4A5 mutation, seven, or 50 %, had complete or partial deletions, compared with a deletion frequency of about 10 % in the general AS population (discussed in [141]). Although it is possible that COL4A5 deletions confer an increased risk for posttransplant anti-GBM nephritis, AS males with COL4A5 deletions have been transplanted successfully [147], indicating that other factors, presently unknown, must influence the initiation and elaboration of the immune response to the allograft. At this time it appears that the only way to determine whether a previously untransplanted AS patient will develop posttransplant anti-GBM nephritis is to perform the transplant, although as noted previously certain patients are at very low risk.
Females who are heterozygous for COL4A5 mutations would not be expected to be at risk for the development of posttransplant anti-GBM nephritis, since the product of the normal COL4A5 allele would allow establishment of immunologic tolerance for α(alpha)5(IV). Nevertheless, posttransplant anti-GBM nephritis has been reported in two females with AS [100, 138]. Both of these women were found to have ARAS, due to COL4A3 mutations [99, 100].
Renoprotective Therapies
Interventions aimed at preventing or slowing the inexorable decline in renal function typical of male AS are currently under investigation. Ramipril therapy initiated prior to the development of proteinuria delayed the onset of proteinuria and renal failure and lengthened survival in a murine model of ARAS [131, 132]. Early angiotensin-converting enzyme inhibition in dogs with XLAS had no effect on the onset of proteinuria, but did delay ESRD [148]. Prospective studies of angiotensin inhibition in AS have involved small numbers of patients with proteinuria serving as their own controls, with relatively short follow-up, and have produced mixed results [149–152]. Recently, retrospective analysis of data in the European Alport Registry showed that early angiotensin blockade was associated with delayed onset of ESRD, with a median interval of 18 years compared to patients who received no therapy [153].
In an uncontrolled study of eight AS males, cyclosporine appeared to suppress proteinuria and stabilize renal function, over several years of observation [154, 155]. However, other investigators found that patient responses to cyclosporine are variable and that cyclosporine may accelerate interstitial fibrosis [156, 157]. In dogs with XLAS, cyclosporine failed to suppress proteinuria, but treated animals did exhibit a delay in progression to ESRD [158]. At this time, it is the author’s view that the efficacy of cyclosporine as a treatment for AS has not been demonstrated with the degree of certainty needed to overcome concerns about its nephrotoxicity.
There has been interest in gene transfer approaches aimed at delivering a normal copy of the COL4A5 gene into glomeruli as a treatment for AS [159–162]. While attractive in theory, it has yet to be demonstrated that such treatment can be accomplished in an experimental model, let alone patients.
A number of other therapies have been shown to improve outcomes in animal models of AS, including inhibitors of TGF-β1 [126], matrix metalloproteinases [163], vasopeptidase A [164], or HMG-CoA reductase [165]. chemokine receptor 1 blockade [166], BMP-7 [129], stem cells [167–170], and irradiation [171]. None of these approaches has been prospectively studied in human AS populations. In comparison with angiotensin blockade, which has been examined extensively in children with chronic renal disease [172, 173], these therapeutic approaches have little or no track record in pediatric populations and would be costlier, less accessible, and probably riskier forms of treatment.
Thin Basement Membrane Nephropathy
TBMN, like AS, is an inherited disorder of glomerular basement membranes that is characterized clinically by persistent microscopic hematuria and episodic gross hematuria. TBMN differs clinically from AS in several important respects: (1) it is only rarely associated with extrarenal abnormalities; (2) proteinuria, hypertension, and progression to ESRD are unusual; (3) gender differences in expression of TBMN are not apparent; and (4) transmission is autosomal dominant in nature. TBMN and early AS may be difficult to distinguish histologically, since diffuse GBM attenuation is characteristic of both. However, in TBMN the GBM remains attenuated for the patient’s lifetime, rather than undergoing the progressive thickening and multilamellation of the lamina densa that are pathognomonic of AS.
Clinical Features
Individuals with TBMN typically exhibit persistent microhematuria that is first detected in childhood. In some patients the microhematuria is intermittent and may not be detected until adulthood. Episodic gross hematuria, often in association with upper respiratory infections, is not unusual. The hematuria of TBMN appears to be lifelong. It has been estimated that 20–25 % of patients referred to a pediatric nephrologist for evaluation of persistent hematuria will prove to have thin GBM on renal biopsy [174].
Overt proteinuria and hypertension are unusual in TBMN but have been described [48, 175–177]. Some of these patients exhibit focal global glomerulosclerosis on renal biopsy [48, 49]. In occasional adult patients with Alport syndrome, the predominant GBM abnormality is attenuation rather than thickening and multilamellation. Other glomerular disorders such as IgA nephropathy may occur in patients with TBMN, altering the expected natural history and histopathology of the condition.
Pathology
Light and immunofluorescence microscopies are unremarkable in typical cases of TBMN. Most patients exhibit diffuse thinning of lamina densa and of the GBM as a whole. GBM thickness is age and sex dependent in the normal population. Both the lamina densa and the GBM increase rapidly in thickness between birth and age 2 years, followed by gradual thickening throughout childhood, adolescence, and into adulthood [178]. GBM thickness of adult men (373 + 42 nm) exceeds that of adult women (326 + 45 nm) [179]. Thus, the age and sex of the patient must be taken into account when evaluating GBM width.
The definition of “thin” GBM in the literature is imprecise, in part because of the use of different techniques to measure GBM width. When an EM laboratory’s normal values for GBM width are similar to those of Steffes et al. [179], a cutoff value of 250 nm will accurately separate adults with normal GBM from those with thin GBM. Where the normal values are significantly higher, a cutoff value of 330 nm is appropriate [180]. For children, the cutoff is in the range of 200–250 nm (250 nm is within 2SD of the mean at age 11) [178, 181]. The intraglomerular variability in GBM width is small in thin GBM disease [176]. In a patient with persistent hematuria, marked variability in GBM width within a glomerulus should raise suspicion for Alport syndrome, although focal lamina densa splitting has been described in TBMN.
Genetics and Pathophysiology
TBMN is usually transmitted as an autosomal dominant condition. A negative family history may not be reliable, since patients eventually diagnosed as having TBMN are frequently unaware that they have relatives with hematuria [182]. The first clue to the genetic basis of TBMN was provided by Lemmink and coworkers, who described a large Dutch TBMN kindred in which the disease locus was first mapped to chromosome 2 in the region of the COL4A3 and COL4A4 genes, and then affected individuals were found to be heterozygous for a missense mutation in COL4A4 [183]. Since this landmark work, heterozygous mutations in COL4A3 or COL4A4 have been described in numerous TBMN families [94, 184–186]. However, linkage to the COL4A3 and COL4A4 genes has been excluded in other TBMN families, indicating that TBMN is a genetically heterogeneous condition [187]. No other genetic loci for TBMN have been identified thus far.
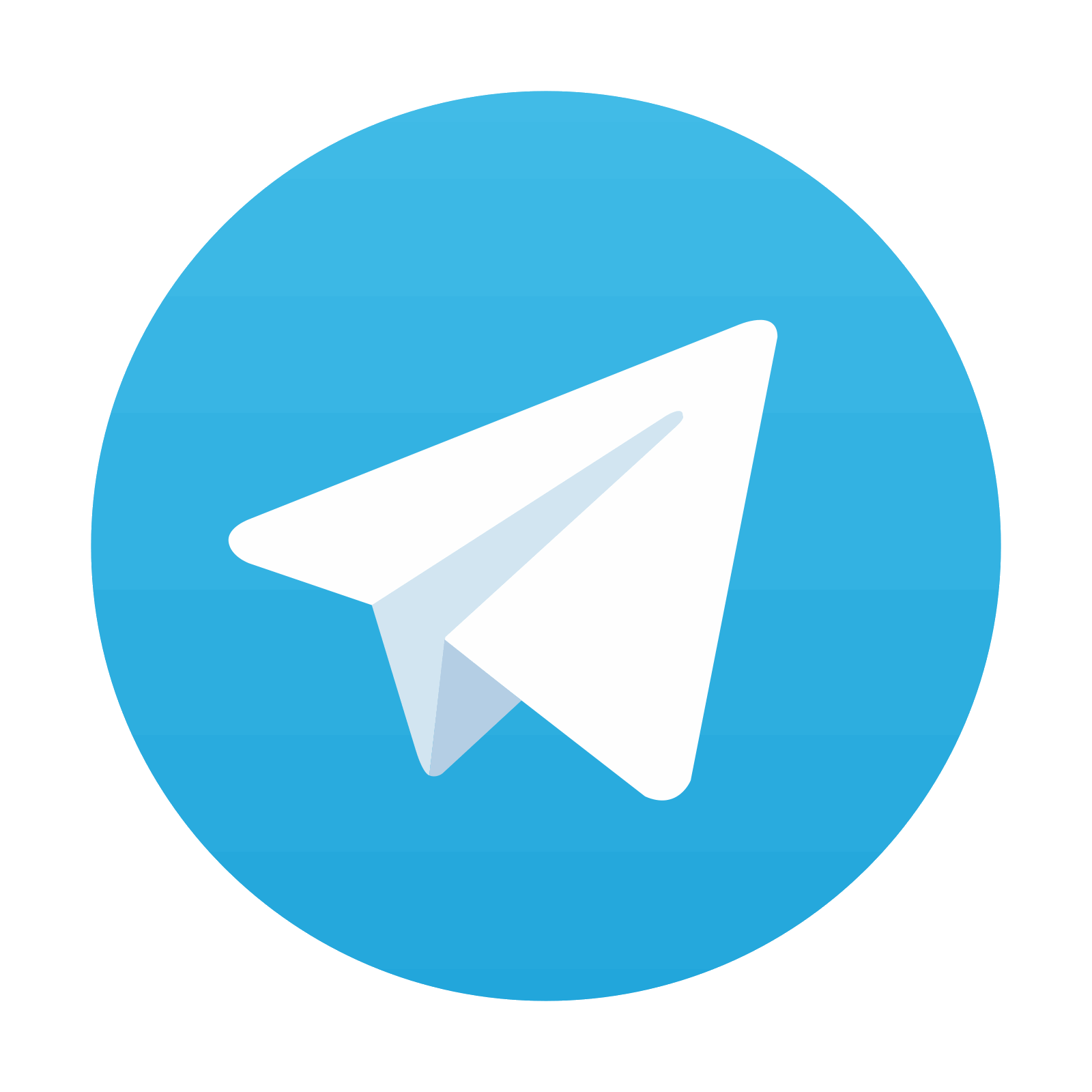
Stay updated, free articles. Join our Telegram channel
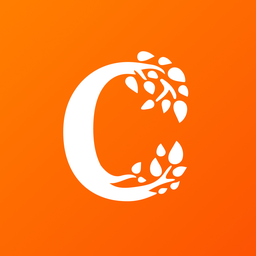
Full access? Get Clinical Tree
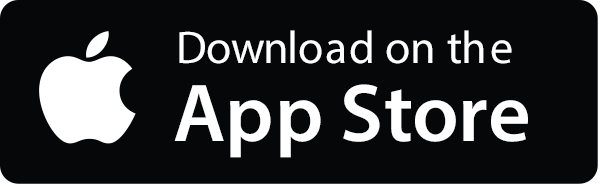
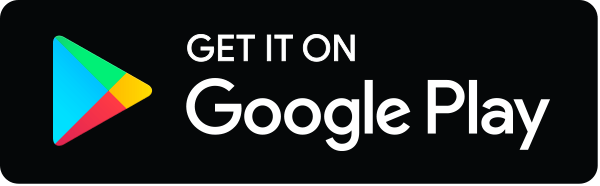