Abstract
The renin-angiotensin-aldosterone system (RAAS) plays a critical role in the maintenance of salt and water homeostasis by the kidneys. However, the inappropriate activation of this system results in abnormal sodium retention, potassium loss, and an increase in blood pressure. The renin-angiotensin system (RAS) is divided into the classical and nonclassical pathways. The classical pathway is comprised of angiotensin converting enzyme (ACE), angiotensin II, and angiotensin II type 1 receptor (AT 1 R). The nonclassical pathway has two components: (1) angiotensin II/angiotensin III/angiotensin IV/AT 2 R and (2) ACE2, angiotensin 1-7, and alamandine/Mas1/MrgD. The nonclassical pathway opposes the nonbeneficial effects of the classical pathway, with exceptions. Both pathways are initiated by renin, primarily synthesized in renal juxtaglomerular cells, and stored as prorenin. The synthesis of certain components of the RAAS occurs to a greater extent in some organs relative to others, such as ACE (lung), aldosterone (adrenal gland), angiotensinogen (liver), and renin (kidney). Some or all of the RAAS components are expressed in adipose tissue, blood vessel, brain, heart, kidney, and pancreas, among others, exerting autocrine/intracrine/paracrine effects. The RAAS is more active in the neonatal period and infancy than later in childhood. However, the degree of AT 1 R and AT 2 R protein expressions in the young, relative to the adult, is organ dependent. Preterm birth alters the development of the RAAS. Drugs that interfere with the RAAS, given to developing fetuses, can program the development of renal abnormalities and hypertension. In addition to genetics, epigenetics, paternal and maternal nutrition, and health-related practices affect the development of the RAAS.
Keywords
aldosterone, angiotensin, angiotensin converting enzyme, angiotensin receptor, dopamine, renin, sodium balance
- •
Components of the Renin-Angiotensin-Aldosterone System
- •
Ontogeny
The renin-angiotensin-aldosterone system (RAAS) plays a critical role in the maintenance of salt and water homeostasis by the kidneys, particularly in hypovolemic and salt-depleted states. However, the inappropriate activation of this system results in abnormal sodium retention, potassium loss, and an increase in blood pressure. In addition, excess angiotensin II (Ang II) and aldosterone can cause inflammation and oxidative stress, both of which can cause chronic kidney disease and aggravate the increase in blood pressure.
Components of the Renin-Angiotensin-Aldosterone System
Angiotensin Generation
The renin-angiotensin system (RAS) is currently divided into the classical RAS and the nonclassical RAS pathways. The classical pathway comprises angiotensin converting enzyme (ACE), Ang II, and Ang II type 1 receptor (AT 1 R) ( Fig. 11.1 ). The nonclassical pathway is composed of two components: one pathway comprises the Ang II/Ang III/Ang IV/AT 2 R, and the other pathway comprises ACE2, Ang 1-7, and alamandine/Mas1/MrgD ( Fig. 11.2 ).


Both the classical and nonclassical RAS pathways are initiated by renin (see Figs. 11.1 and 11.2 ), which is primarily synthesized in renal juxtaglomerular cells (smooth muscle cells in the walls of the afferent arteriole as it enters the glomerulus) and stored as prorenin. The principal cells and intercalated cells of the renal collecting duct also synthesize renin and prorenin, respectively. Renin is also synthesized in the connecting segment. Renin enzymatically causes the formation of Ang I (Ang 1-10) from angiotensinogen, its only substrate, with the liver as the major source. The binding of renin and prorenin to the (pro)renin receptor (PRR) activates prorenin and markedly enhances renin activity. In the classical pathway, Ang I is acted upon by ACE to form Ang II. The rate-limiting step in the activation of the RAS is the release of renin, which is the most well-regulated component of the RAS. Renal renin secretion is stimulated by three primary pathways: (1) stimulation of renal baroreceptors by a decrease in afferent arterial stretch (pressure) ; (2) stimulation of renal β 1 -adrenergic receptors, partly through increased renal sympathetic nerve activity ; and (3) a decrease in sodium and chloride delivery to, and transport by, the macula densa. Renin secretion can also be regulated by several endocrine and paracrine hormones, hypoxia, and gases (e.g., H 2 S). It should be mentioned that angiotensinogen may have Ang II-independent effects, including the regulation of body weight and the induction of atherosclerosis.
Renin-Angiotensin System Outside Juxtaglomerular Cells
The RAS has been demonstrated in tissues other than the kidney. Synthesis of certain components of the RAAS occurs to a greater extent in some organs relative to others, such as ACE in the lung, aldosterone in the adrenal glands, angiotensinogen in the liver, and renin in the kidney, which function together as an endocrine system. Some or all of the RAAS components are expressed in the adipose tissue, blood vessel, brain, heart, kidney, pancreas, and placenta, among others, exerting autocrine, intracrine, and paracrine effects. This adds complexity to the understanding of the regulatory effects of the RAAS in maintaining body homeostasis. Extraglomerular sites of prorenin synthesis include the adrenal cortex and medulla, adipocyte, brain, eye (retina and vitreous humor), heart, mast cells, ovarian follicle, chorionic villus of the placenta, renal collecting duct cell, submandibular gland, testis and male reproductive system, uterus (endometrium, decidua basalis, and decidua vera), and vascular wall, among others. The sites of synthesis of components of the RAS are species specific. The components of the RAAS can also be trapped from the circulation, renin is trapped in the brain of mice, for example. Angiotensinogen is produced in extrahepatic sites, such as the adrenal gland, brain, cardiac atrium, kidney, large intestine, lung, mesentery, ovary, spinal cord, stomach, and spleen and is also expressed in the ventricle and conducting tissue of the heart. ACE is ubiquitously expressed. However, the conversion of circulating Ang I to Ang II by ACE occurs mainly in the lung. ACE2 has been consistently identified in the human heart, kidney, and testis, and may be present in other tissues as well.
Effects of Angiotensin Converting Enzyme and Nonangiotensin-Converting Enzymes Other Than the Production of Angiotensin II
As mentioned earlier, the RAS is currently divided into the classical and the nonclassical RAS. The activation of the classical pathway results in an increase in blood pressure, directly, by vasoconstriction and extracellular volume expansion, and by an increase in renal and intestinal electrolyte transport and water flux, and indirectly by stimulation of the synthesis of mineralocorticoids, especially aldosterone. Ang II acting in brain centers (e.g., lateral paraventricular, median preoptic, lateral parabrachial, and supraoptic nuclei) can also increase salt and water intake. Inappropriate, excessive, or prolonged stimulation of the classical RAS can lead to abnormal cell growth, fibrosis, inflammation, myocardial ischemia, obesity, and oxidative stress, among others. By contrast, the nonclassical pathway is perceived to oppose the nonbeneficial effects of the classical pathway, with exceptions, to be discussed subsequently.
ACE acts on Ang I (Ang 1-10) to cleave off the active octapeptide, Ang II (Ang 1-8), which is a more potent vasoconstrictor than Ang I (see Fig. 11.1 ). Ang II can also be formed from Ang I by non-ACE enzymes and nonrenin enzymes, such as carboxypeptidase, cathepsin G, chymase, elastase-2, metallo-proteinase 8, and tonin. The roles of these enzymes in humans need elucidation, as tonin has not been reported in humans. Nevertheless, these non-ACE, nonrenin enzymes assume greater significance in the organs where not all components of the RAAS are expressed, providing alternate means of generation of Ang II. For example, mast cells produce renin and have chymases, which help form Ang II and may play a role in heart failure and generation of arrhythmias. Mast cell chymases limit the cardiac efficacy of ACE inhibitors. It should be noted that ACE is also known as kininase 2, which is involved in the degradation of bradykinin, a vasodepressor. Therefore, inhibition of ACE not only prevents the formation of Ang II with its vasopressor effect, but also allows the vasodepressor effect of bradykinin to persist.
ACE2 is a homolog of ACE, with about 42% sequence homology in humans. ACE2 is abundantly expressed in the kidney, specifically in the brush border of proximal tubule cells where it colocalizes with ACE. ACE2, by catalyzing the conversion of Ang I and Ang II to Ang 1-7 and alamandine (see Figs. 11.1 and 11.2 ), provides the balance between Ang II on the one hand and Ang 1-7 and alamandine, on the other, in the kidney and other tissues where these pathways may occur. In neonatal rat cardiomyocytes, aldosterone increases the expression of ACE, but the opposite is observed for ACE2. ACE2 is a carboxypeptidase and the main catabolic enzyme of Ang II, generating Ang 1-7. Ang 1-7, probably by occupying the Mas1 receptor and Mas-related gene receptor, MrgD, has natriuretic, vasodilator, and antiproliferative properties and counteracts the effects of Ang II. Ang 1-7 can also regulate lipid metabolism, decreasing cholesterol and triglyceride levels. ACE2 also decreases the levels of Ang II by converting Ang I to Ang 1-9. Thus, ACE and ACE2 exert opposing roles; a decrease in ACE2 may account for increased levels of Ang II, and the opposite occurs with ACE. Sympathetic vasoconstriction in systemic disorders of vascular regulation may be related to ACE2 deficiency, leading to a decrease in Ang 1-7. The ACE2-Ang 1-7–Mas axis plays a protective role in the pathogenesis of hypertension, regulation of renal function, and progression of renal disease, including diabetic nephropathy. A decrease in the expression or function of this system may play a critical role in the progression of cardiovascular diseases, as elucidated below. Ang 1-7 can also act as a biased AT 1 R agonist, resulting in the recruitment of β-arrestin 2, antagonizing the detrimental effects of Ang II. A homologue of ACE2, collectrin, which does not have a catalytic domain, may also be protective against hypertension.
The processing and effects of angiotensins are also species-, tissue-, and sex-specific.
Cardiovascular System
Ang I can be converted to Ang 1-9 by ACE2, chymase, and cathepsin A (aka carboxypeptidase A). The main products of Ang 1 due to ACE and chymase are Ang 1-9 and Ang II in the heart, as well as arteries, and Ang 1-9, via cathepsin A, but not Ang II in platelets. Circulating Ang 1-9 levels are increased after myocardial infarction and inhibit cardiac hypertrophy. Whereas Ang 1-9 is prothrombotic, Ang 1-7 is antithrombotic.
Adipocyte
In adipocytes, Ang 1-7 derived from Ang 1-9 is the main degradation product, and Ang III inhibits insulin-regulated amino peptidase (IRAP), which also possesses a binding site for Ang IV. White adipose tissue is the major site of angiotensinogen synthesis outside the liver, contributing to about 20% of circulating angiotensinogen. Ang II increases adipose tissue angiotensinogen expression, a case of positive feedback. ACE2, which converts Ang 1 to Ang (1-9) and Ang II to Ang (1-7), is upregulated by high-fat diet and decreases adipocyte inflammation. Mice lacking angiotensinogen or AT 2 R are protected from high-fat-diet–induced obesity and featured adipose tissue hypotrophy. Therefore, it seems that Ang II via AT 2 R and Ang (1-7) via Mas1 or MrgD counteract each other.
Kidney
In rat glomeruli, Ang I is mainly converted to Ang 1-7 via neprilysin and Ang 2-10 via aminopeptidase A (APA), in one study, and to Ang II, Ang 1-9, Ang 1-7, and Ang 3-8, enzyme(s) not determined, in another study. In mouse podocytes, the main product of Ang I is Ang 1-7, via neprilysin. Podocytes can also convert Ang II to Ang III; angiotensinogen, renin, AT 1 R, AT 2 R, and PRR are also expressed in podocytes. By contrast, human mesangial cells mainly convert Ang I to Ang II. In several animal models, renal intratubular Ang II has been found to be higher than that found in the circulation. In the mouse, components of the RAS, except ACE, are expressed in the proximal tubule; renin is also expressed in the distal convoluted tubule and cortical collecting duct, and angiotensinogen is also expressed in the thin descending and thick ascending limbs of Henle. Prorenin is expressed in the intercalated cells of the collecting duct. Thus, angiotensinogen can be converted to Ang I in the proximal and distal convoluted tubules and cortical collecting duct and, in turn, converted into Ang II by ACE from the renal vasculature or systemic circulation. However, liver-derived rather than proximal tubule–derived angiotensinogen may be the primary source of intrarenal angiotensinogen, under physiologic conditions. On normal sodium intake, angiotensinogen within the S1 and S2 segments of the proximal tubule comes from the systemic circulation, while angiotensinogen in the S3 comes from endogenous synthesis.
Blood Vessel
Vascular smooth muscles can also synthesize Ang II, in which the primary source of renin and angiotensinogen is from the plasma. However, all the other components of the RAS are expressed in the vascular wall. Ang 1 is produced from angiotensinogen via cathepsin D, rather than renin, and chymase rather than ACE converts Ang I to Ang II in injured vessels. Ang 1-7 formed in the vascular smooth muscle interacts with Mas1 in the endothelial cells. In rat mesenteric arteries, Ang I is converted by cathepsin A1 to Ang 1-7, with Ang 1-9 and Ang II as intermediates. By contrast, Ang 1 is converted by cathepsin A2 to Ang 1-9.
Renin-Angiotensin System and Brain
The existence of local brain RAS was suggested by the demonstration of renin within the brain of nephrectomized dogs. Although there are studies suggesting that brain renin represents trapped plasma renin, there are more studies that attest to the presence of a local brain RAS. In the brain, angiotensinogen is expressed more than renin, and ACE is expressed in brain areas that are important in cardiovascular regulation. The subfornical organ is important in angiotensin-mediated drinking and blood pressure regulation. Drinking and whole body metabolism may be regulated by a brain-specific isoform of renin, which negatively regulates the brain RAS. In the subfornical organ, the AT 1 R increases fluid intake while the AT 1 R increases blood pressure. In the arcuate nucleus, the AT 1 R and leptin receptor interact to regulate resting metabolic rate. In the brain, Ang II may be the primary AT 1 R agonist.
Angiotensin II and Its Metabolites
Ang II has at least three metabolites: Ang III (Ang 2-8), Ang A (Ala 1 -Ang 1-8), and Ang 1-7 (see Fig. 11.2 ). Ang III (Ang 2-8) differs from Ang II by absence of Asp 1 and by the action of APA on Ang II. Ang III, as with Ang II, participates in the classical effects on body fluid and electrolyte homeostasis, such as drinking behavior, vasopressin release, and sodium appetite in brain centers. However, Ang III has been shown to exert a natriuretic effect via its interaction with the AT 2 R. Aminopeptidase N (APN) acts on Ang III to form Ang IV (Ang 3-8). Ang IV stimulates AT 4 R and AT 2 R and inhibits AT 1 R in one study. Ang IV negatively regulates APA and thus influences the generation of Ang III. Ang IV can be cleaved to Ang 3-4 by dipeptidyl peptidase or neprilysin. Ang 3-4 can also be formed from Ang 1-7 by the action of a series of enzymes (see Fig. 11.2 ); Ang 3-4 is natriuretic by occupying AT 2 R.
Ang A differs from Ang II because of a change in the first amino acid from Asp to Ala (Ala 1 -Ang 1-8), presumably by decarboxylation of Ang II that may involve the mononuclear leucocyte aspartate decarboxylase. Although Ang A may have affinity for both AT 1 R and AT 2 R, Ang A, via AT 1 R, is mainly a vasoconstrictor and increases blood pressure, although at much higher concentrations than Ang II. Unlike Ang II, Ang A does not increase the amplitude of the calcium transient in isolated rat ventricular myocytes, acting as a biased agonist.
Ang 1-7 differs from Ang II by the absence of Phe 8 ; it is derived from Ang II by the action of ACE2, as well as prolyl carboxypeptidase, prolyl endopeptidase, and thimet oligopeptidase. In the central nervous system, Ang 1-7 may also inhibit norepinephrine synthesis and release. The physiologic effects of Ang 1-7, such as vasodilation, natriuresis, and antiangiogenesis, counteract the effects of Ang II by occupying the AT 2 R and MrdG. Ang 1-7 also inhibits Ang II-stimulated phosphorylation of mitogen-activated protein (MAP) kinase in rat renal proximal tubule cells. The ability of Ang 1-7 to counteract the effects of Ang II (e.g., cell proliferation and extracellular matrix synthesis) in rat renal mesangial cells is exerted via Mas1. However, Ang 1-7, by itself, can mimic the ability of Ang II to stimulate cell proliferation and extracellular matrix synthesis in these cells. The significance of this apparently detrimental effect of Ang 1-7 remains to be determined.
Alamandine is a heptapeptide, which is derived from Ang A by the cleavage of phenylalanine (Ala 1 -Ang 1-7) by the action of ACE2. Alamandine also can be derived from Ang 1-7 by the decarboxylation of its aspartate residue; the specific enzyme has not been definitely determined but may involve ACE. There are similarities and differences between Ang 1-7 and alamandine. As with Ang 1-7, alamandine is a vasodilator, in part by activating nitric oxide. Both alamandine and Ang 1-7 can inhibit the vasoconstriction of the rabbit aorta induced by Ang A but not that caused by Ang II. In the diseased aorta, neither Ang 1-7 nor alamandine had any effect on Ang A-induced vasoconstriction; however Ang 1-7, but not alamandine, increased Ang II-mediated vasoconstriction. However, in the human internal mammary artery, Ang 1-7 has been reported to antagonize the vasoconstrictor effect of Ang II. While alamandine reduces leptin expression in peri-renal adipocytes, Ang 1-7 increases it. Unlike Ang 1-7, which can activate both Mas1 and MrgD receptors, alamandine activates only the MrgD receptor. Alamandine can also exert is vaosodepressor effect by acting at the rostral ventrolateral medulla.
In summary, the RAS is now accepted to be present in many tissues. There are two pathways: the classical RAS comprises ACE, Ang II, and AT 1 R, whereas the non-RAS is composed of two components: Ang II/Ang III/Ang IV and AT 2 R component; and the ACE2, Ang 1-7, and alamandine component. The classical pathway is responsible for increasing water and sodium intake, sodium retention, and vasoconstriction; overactivation of the classical pathway leads to oxidative stress, inflammation, fibrosis, and cell growth, while the nonclassical pathway, in general, opposes the actions of the classical pathway. However, not all members of the nonclassical pathway oppose the classical pathway because while Ang IV, via AT 4 R, has been reported to inhibit inflammation in the heart with ischemia/reperfusion injury, it may also promote vasoconstriction, cell proliferation, and inflammation. In a model of Ang II-induced abdominal aneurysm, a medium dose but not a high dose of Ang IV exerted a beneficial effect, in part by decreasing inflammation. The RAS also could be viewed as an ACE pathway and is composed of two distinct and opposing arms: the ACE pathway, which generates Ang II, acting via the AT 1 R to subserve the biologic effects of vasoconstriction and the increase in renal sodium transport, and the ACE2 pathway, which generates an endogenous antagonist to Ang II, namely Ang 1-7 activating the oncogene Mas1 receptor protein to subserve vasodilatory and antiproliferative effects on the vasculature and decreased epithelial ion transport. In the brain, ACE2 activation also opposes the effects of Ang II exerted via the AT 1 R. The RAS has also been suggested to be divisible into five axes: (1) classic angiotensinogen/renin/ACE/Ang II/AT 1 R; (2) Ang II/APA/Ang III/AT 2 R/NO/cyclic guanosine monophosphate (cGMP); (3) Ang I/Ang II/ACE2/Ang 1-7/Mas1 receptor; (4) prorenin/renin/(pro)renin receptor (PRR or Atp6ap2)/MAP kinases ERK1/2/V-ATPase; and (5) Ang III/APN/Ang IV/IRAP/AT 4 R.
Aldosterone
Aldosterone secretion by the zona glomerulosa cells of the adrenal gland is normally regulated by Ang II and potassium, which is mediated by an increase in intracellular calcium. ACTH becomes a very important stimulus of aldosterone secretion under conditions of volume depletion. Mast cells located in the subcapsular region of the human adrenal cortex also stimulate aldosterone secretion by releasing serotonin. Extraadrenal sites of aldosterone synthesis include the adipose tissue that secretes aldosterone-releasing factors. An increase in circulating aldosterone increases local aldosterone production in hypothalamic nuclei (e.g., supraoptic nucleus and parventricular nucleus), which participates in aldosterone-induced increase in salt and water intake. The brain AT 1 R may preferentially respond to Ang III that is critical for the hypertensinogenic effect of Ang II. The RAS in cardiac myocytes plays a role in the ventricular remodeling associated with salt retention. In neurons and cardiomyocytes, the effects of aldosterone are opposed by glucocorticoids. However, under conditions of cardiac damage, corticosterone, rather than aldosterone, may be the activator of mineralocorticoid receptors. Both corticosterone and aldosterone increase cardiac contractility; however, corticosterone increases, whereas aldosterone decreases, coronary flow. Depending on the species and experimental conditions, aldosterone may have positive (rat heart) or negative (human heart) inotropic effect. Aldosterone promotes salt and water retention by stimulating sodium transport mediated by the epithelial sodium channel (ENaC) in the distal nephron. Aldosterone can also increase sodium transport in the renal proximal tubule by the stimulation of the Na + /H + exchanger types 1 and 3 (NHE1 and NHE3, respectively), as well as Na + ,K + /ATPase.
Gene Targeting of Angiotensin Synthesis: Lessons From Genetically Manipulated Rodents
Genetic ablation of angiotensinogen and ACE in separate lines of mice uniformly results in low blood pressure, abnormal renal development, renal malfunction, and low hematocrit. Tissue-specific targeted ablation helps to elucidate the paracrine and autocrine effects of tissue RAS. As indicated earlier, the main source of renal angiotensinogen is the liver; liver-specific but not renal-specific deletion of angiotensinogen decreases renal angiotensinogen and Ang II proteins. Blood pressure is decreased in both instances. However, the reduction in urinary angiotensinogen in renal- but not liver-specific deletion of angiotensinogen needs to be explained. Glial-specific ablation of angiotensinogen in mice decreases blood pressure and causes diabetes insipidus. As aforementioned, there is a report indicating that brain renin represents trapped plasma. However, ablation of the brain-specific promoter (renin-b) but not preprorenin caused hypertension, via the renin, ACE, and AT 1 R pathway in the brain. These genetic manipulations support the notion that the central nervous system contributes to the regulation of blood pressure via the RAS. Ablation of the renin gene in mice reduces aldosterone secretion in the zona glomerulosa and impairs aldosterone production. Systemic genetic ablation of many genes of the RAS (e.g., angiotensinogen, ACE) results in normal mice at birth; however, death generally ensues before 3 weeks of age. In addition to gene-targeted deletion, tissue-specific targeting of RAS overexpression has been accomplished. Transgenic mice overexpressing the human angiotensinogen and renin, specifically in renal proximal tubules, develop hypertension. The introduction of the mouse Ren-2 gene into normotensive rats creates a transgenic strain that expresses Ren-2 mRNA in the adrenal gland, heart, and kidney. This transgenic rat is a monogenic model for a form of sodium-dependent malignant hypertension. These transgenic rats are characterized by high plasma prorenin but unchanged or even suppressed concentrations of active renin, Ang 1, Ang II, and angiotensinogen, compared with transgene-negative littermates. Renal renin is suppressed while adrenal renin and corticosteroid production is increased. Transgenic mice, with selective overexpression of angiotensinogen in the renal proximal tubule, are also hypertensive despite having normal plasma angiotensinogen levels and renin activity. However, urinary angiotensinogen and Ang II concentrations are increased. Blockade of the ET A receptor in young Ren-2 transgenic rats decreases blood pressure and ameliorates end-organ damage, suggesting a potential application in the management of hypertension in newborn babies.
ACE-deficient mice have low blood pressure and severe renal disease characterized by vascular hyperplasia of the intrarenal arteries, perivascular infiltrates, paucity of renal papillae, and impaired concentrating ability, which highlight the critical role of ACE in the development of the kidneys. Inhibitors of ACE have been identified in hypoallergenic infant milk formulas containing hydrolyzed milk proteins, which could potentially affect renal function later in life. ACE inhibition in young rats has been reported to cause retardation of glomerular growth (see below). Mice with renal-specific ablation of ACE have normal serum Ang II, blood pressure, and renal architecture under basal conditions. However, there is a marked attenuation of the hypertensinogenic effect of intravenously administered Ang II and inhibition of nitric oxide synthesis with L-NAME, as well as a decrease in the expression of renal sodium transporters.
ACE2-deficient mice develop dilated cardiomyopathy, as well as severe hypertensive nephropathy in response to chronic Ang II infusion, with impairment of renal function that is related to progressive renal fibrosis and inflammation. ACE2-deficient mice develop impaired endothelium-dependent and -independent relaxation in vitro and in vivo, which is associated with a decrease in eNOS expression and nitric oxide (NO) concentration in the aorta. Lack of ACE2 also predisposes to high-fat-diet–induced nephropathy, atherosclerosis, and arterial neointima formation. A decrease in ACE2 expression has been implicated in the delayed hypertension observed in sheep treated antenatally with betamethasone, which needs further elucidation in humans, given the widespread use of antenatal steroids to enhance lung maturity in infants born prematurely. ACE2 effects extend beyond the renal and cardiovascular systems; ACE2 has been reported to be a key regulator of dietary amino acid homeostasis, innate immunity, and gut microbial ecology, which may increase a susceptibility to develop colitis. ACE2 deficiency also impairs glucose tolerance and may predispose to the development of diabetes. However, the β-cell defect of the pancreas with ACE2 deficiency is not dependent on Ang II but rather on the collectrin-like action of ACE2. There are no reports of organ/tissue-selective deletion of ACE2. However, gonadectomy prevents the increase in blood pressure and glomerular injury in diabetic male mice lacking ACE2.
Renin is no longer expressed after removal of the kidneys but prorenin is still present in the blood; renin in anephric subjects has been suggested to be “open” prorenin. Therefore, the kidney may be the only renin-secreting organ while prorenin is secreted from nonrenal tissues (see later). The consequences on blood pressure on the deletion of non-ACE genes related to the RAS have not been reported.
Gene Targeting of Angiotensin Receptors: Lessons From Genetically Manipulated Rodents
Angiotensin Receptors
The effects of the angiotensinogen metabolites, such as Ang II (Ang 1-8), Ang III (Ang 2-8), and Ang IV (Ang 3-8), Ang 1-7, Ang A (Ala1-8), alamandine (Ala1-7), and Ang 3-4 and prorenin, are mediated by their occupation of specific angiotensin receptors. There are six receptors of the RAS: AT 1 R, AT 2 R, Mas1, and MrgD, all of which are G protein–coupled receptors with seven transmembrane domains ; AT 4 R and PRR have one transmembrane domain. An AT 3 R was cloned from the rat adrenal cortex.
Ang II interacts mainly with two receptors, AT 1 R and AT 2 R. Adult human renal vasculature, glomeruli, and tubules (proximal and distal convoluted tubule, ascending limb of Henle, and collecting duct) express AT 1 R; AT 2 R is expressed in the vasculature and glomeruli, but not in the tubules. In rodents, AT 2 R is expressed in most segments of the nephron. Whereas human Ang II, Ang III, and Ang A are full agonists at the human AT 1 R, Ang IV binds to this receptor with low affinity. The conversion of Ang II to Ang III is necessary for its interaction with the AT 2 R to cause natriuresis. However, Ang III is also a full agonist of AT 2 R; Ang IV, Ang A, Ang 1-9, Ang 1-7, and Ang 3-4 are AT 2 R agonists (see Fig. 11.3 ). The human AT 1 R gene (AGTR1) is located in chromosome 3q24. The human AT 2 R gene (AGTR2) is located in chromosome Xq23.

The current understanding that AT 2 R expression is higher in fetal life than in newborns or adults has been challenged by recent observations in rats, wherein AT 2 R protein expression is lower and AT 1 R expression is higher in the fetal and neonatal brain and kidneys than in adults, but the expression of AT 2 R is higher in the fetal and neonatal liver compared with adults.
Occupation of the AT 1 R by Ang II triggers the generation of various second messengers via heterotrimeric G proteins, mainly G q/11 . Phospholipase C (PLC) β1 is activated, leading to the formation of 1,4,5-inositol trisphosphate (IP 3 ) and diacyl glycerol (DAG) from the hydrolysis of phosphatidylinositol-4,5-bisphosphonate. IP 3 activates IP 3 receptors in the endoplasmic reticulum, releasing Ca 2+ . Ca 2+ released from the endoplasmic reticulum causes the Ca 2+ -sensing stromal interaction molecule protein to interact with Orai1 in the plasma membrane. This interaction, together with the activation of IP 3 receptors at the plasma membrane, allows the influx of extracellular calcium. The increase in intracellular calcium and the stimulation of protein kinase C (PKC) by DAG lead to vasoconstriction. Activation of the AT 1 R stimulates growth factor pathways, such as tyrosine phosphorylation and PLCγ activation, leading to the activation of downstream proteins, including MAP kinases, and signal transducers and activators of transcription (STAT protein). These cellular proliferative pathways, mediated by the AT 1 R, have been implicated in the proliferative changes seen in cardiovascular and renal diseases. AT 1 R signaling may also be positively affected by reactive oxygen species (ROS) and reactive nitrogen species, but NO may decrease AT 1 R signaling by cysteine modification of the nuclear transcription factor, NFκB.
The AT 1 R and AT 2 R are differentiated based on their affinity for various non-peptide antagonists. The human AT 2 R has 34% sequence homology with the human AT 1 R but activates second messenger systems with opposite effects via various signal transduction systems, mainly G i and G o proteins. Stimulation of the AT 2 R leads to the activation of various phosphatases, resulting in the inactivation of extracellular signal-regulated kinase (ERK), the opening of K + channels, and the inhibition of T-type Ca 2+ channels. The AT 2 R has a higher affinity for Ang III than Ang II; indeed, Ang III may be the preferred ligand for the AT 2 R, exerting its natriuretic effect in the kidney. Whereas AT 1 R causes increased ion transport, vasoconstriction, inflammation, and immunity and decreased longevity, AT 2 R mediates antiproliferative effects, apoptosis, differentiation, and possibly vasodilatation, offering therapeutic targets for the treatment of cardiovascular diseases. An increase in the expression of AT 1 R and a decrease in the expression of AT 2 R are associated with increased hypertensive renal injury in rodents.
Gene Targeting of Angiotensin Receptors
Additional support of the importance of the RAS in the kidney in the regulation of blood pressure comes from renal cross-transplantation studies in mice with or without the AT 1 R gene. The deletion of AT 1 R gene in the renal proximal tubule of mice also decreases basal blood pressure and response to exogenous Ang II.
Other Angiotensin Receptors
In addition to AT 1 R and AT 2 R, there are three other angiotensin receptors (i.e., AT 4 R, Mas1, and MrgD) and possibly a fourth one, AT 3 R.
The AT 3 R represents an angiotensin-binding site identified in a mouse neuroblastoma cell line. The AT 3 R has a high affinity for Ang II but a low affinity for Ang III. An AT 3 R was cloned from the rat adrenal cortex. However, its existence in humans has not been shown.
The AT 4 R is an angiotensin-binding site with a high affinity for Ang IV. Unlike the AT 1 R and AT 2 R, the AT 4 R is a class II receptor and has one transmembrane domain and is not coupled to heterotrimeric G proteins. AT 4 R has been identified as IRAP. Human IRAP is in chromosome 5q21. However, IRAP may not be the only receptor for Ang IV.
Mas1 receptor, encoded by MAS1, has been identified as the receptor for Ang 1-7. The human MAS1 is located at chromosome 6q25.3-q26. As aforementioned, Ang 1-7 can also activate the MrgD receptor (see below). Ang 1-7 has kinin-like effects that are transduced by the Mas1 receptor, apparently because of heterodimerizaton of Mas1 with the bradykinin 2 receptor (B 2 R). The ability of Ang 1-7 to inhibit noradrenergic neurotransmission and stimulate nitric oxide production may be due to stimulation of B 2 R. Ang 1-7 may also stimulate the production of prostaglandins, via Mas1.
Human MRGD is a single copy gene located in chromosome 11q13.3. As aforementioned, it belongs to the G protein-coupled receptor family with seven transmembrane domains. MrgD is a receptor for both alamandine and Ang 1-7. Alamandine increases NO production whereas Ang 1-7 increases arachidonic acid synthesis. MrgD couples to Gq/11 and pertussis toxin (PTX)-sensitive Gi/o proteins. MrgD-deficient mice have been generated, but their blood pressure phenotype has not been reported. However, in the two-kidney, one-clipped kidney model, the intravenous infusion of alamandine initially increases blood pressure, via the AT 1 R, and subsequently decreases blood pressure, via MrgD. By contrast, alamandine increases the blood pressure in normotensive rats. The hypotensive effect of alamandine injected into the caudal ventrolateral medulla may be via the Mas1 and AT 2 R.
(Pro)Renin Receptor
The discovery of the PRR adds another layer of complexity to the understanding of the scope and extent of the RAAS. PRR is a receptor for both renin and prorenin. Circulating levels of prorenin are 10 times higher than levels of renin. The PRR, so called because it binds to renin and prorenin, regulates intracellular profibrotic and cyclooxygenase genes independent of Ang II. The binding affinity of prorenin for the PRR is two to three times the affinity of renin for the PRR. The PRR has catalytic (generation of Ang II) and non-catalytic signal transduction effects (which lead to hypertension and glomerulosclerosis). Furthermore, the myriad of intracellular signaling pathways mediated by this receptor may hold the key to the mechanisms underlying important developmental processes and the progression of diseases, such as hypertension and diabetes. For example, ablation of the prorenin ATP6ap2 gene results in embryonic lethality. By associating with vacuolar H + -ATPase, the PRR is essential for the Wnt/β catenin signaling molecular pathways, now known to be responsible for neural and renal embryonic development (see Fig. 11.1 ).
Whole body overexpression of human PRR in mice causes slowly progressive glomerulosclerosis that is independent of tissue Ang II. Inducible renal-tubular specific knockout of PRR in mice does not change basal blood pressure. However, the absence of renal tubular PRR promotes sodium wasting and attenuates the hypertensive response to Ang II. PRR, via direct prorenin/renin stimulation of PKA/Akt-dependent pathways, stimulates ENaC activity in the collecting duct. A similar renal tubular-specific deletion of PRR in another strain of mice has no effect on the RAS but the mice have renal concentration defect and distal renal tubular acidosis, caused by impaired V-ATPase activity. Low concentrations (nM) of prorenin increase ENaC activity that is not affected by AT 1 R blockade in cultured mouse medullary collecting ducts cells and microdissected renal collecting ducts. The specificity of these studies has been questioned because the concentrations of prorenin used is much higher than the EC50 for renin. Nevertheless, renal collecting duct-selective silencing of PRR or renin similarly blunts the Ang II-mediated increase in urinary renin activity and the increase in ENaC, relative to control floxed mice. The silencing of PRR in the subfornical organ of the brain attenuates Ang II-induced hypertension in human renin-angiotensinogen double-transgenic mice and prevents deoxycorticosterone acetate/salt-induced hypertension by inhibiting brain Ang II formation. The intracerebroventricular infusion of a PRR antagonist also prevents prorenin-induced hypertension in C57Bl6/J mice. The hypertension mediated by brain PRR also may be related to an increased sodium appetite.
Physiologic Effects of Angiotensin II
Via the Ang II Type 1 Receptor
Ang II exerts most of its effects via the AT 1 R. Ang II has pleiotropic actions, including direct and indirect vasopressor effects. In response to sodium depletion, hypotension, or hypovolemia, Ang II synthesis is increased, which causes immediate vasoconstriction of arteries and veins, and activates the sympathetic nervous system, increasing peripheral vascular resistance and venous return, respectively, and raising blood pressure. The effect of Ang II on blood pressure secondary to increased ion transport by the renal tubule and intestines is more gradual. Ang II increases sodium and chloride reabsorption directly in several segments of the nephron. In the proximal tubule, low concentrations of Ang II play a central role in ion transport by increasing the activity of renal tubular luminal NHE3, Na-glucose cotransporter, sodium phosphate cotransporter (NaPi-II), and basolateral Na + ,K + -ATPase and Na + -HCO3 − cotransporter. AT 1 R stimulates NHE3 but not NHE2 activity in brush-border membranes of renal proximal tubules. High concentrations of Ang II can inhibit proximal tubule sodium transport via the stimulation of PLA2- and cytochrome P450–dependent metabolites of arachidonic acid. Ang II also affects ion transport in the medullary thick ascending limb of the loop of Henle in a biphasic manner. In this nephron segment, low concentrations of Ang II increase sodium, potassium, and chloride transport by stimulating NHE3 and Na + ,K + , 2Cl − cotransporter activities. The inositol 1,4,5-trisphosphate receptor-binding protein released with inositol 1,4,5-trisphosphate is important in the stimulatory effect of Ang II on ion transport in renal proximal tubules. Ang II stimulates NHE1 activity in the macula densa. Ang II also stimulates ENaC activity in the collecting duct and NHE2 in the distal convoluted tubule, but not in the proximal tubule. Ang II also stimulates water transport in the collecting duct by regulating aquaporin II. All of these effects are mediated by the AT 1 R.
Via the Ang II Type 2 Receptor
The role of the AT 2 R in influencing sodium transport is not well established. AT 2 R inhibits sodium transport. As mentioned previously, the natriuresis mediated by the AT 2 R occurs because of its interaction with Ang III. AT 2 R is coupled to the sodium/hydrogen exchanger type 6 (NHE6), but NHE6 is not involved in renal tubular sodium reabsorption. Ang 1-7 has been reported to inhibit Na + ,K + -ATPase in pig outer cortical nephrons and Na + -HCO 3 − exchanger in mouse renal proximal tubules. However, an increase in sodium transport via the AT 2 R in rat proximal tubules has also been reported. These discrepancies may be related to the condition of the animal. For example, AT 2 R inhibits Na + /K + -ATPase activity in renal proximal tubules of obese, but not lean, rats and during AT 1 R blockade. In human renal proximal tubule cells, the relatively low expression of AT 2 R is increased with its heterodimerization with the dopamine D 1 receptor (D 1 R). This results in a cooperative increase in cyclic adenosine monophosphate (cAMP) and cGMP production, protein phosphatase 2A activation, Na + /K + -ATPase internalization, and sodium transport inhibition. The ability of Ang II to increase NO production via the AT 2 R has been shown to be the mechanism by which Ang II can decrease sodium transport in rat thick ascending limb and renal proximal tubule cells.
Indirect Effects
Ang II indirectly increases sodium transport, partly by stimulating the synthesis of aldosterone in the zona glomerulosa of the adrenal cortex. Aldosterone increases ENaC activity by inducing the transcription of α-ENaC and redistribution of α-ENaC in the connecting tubule and collecting duct from a cytoplasmic to an apical location. However, Ang II can stimulate the expression of α, β, and γ ENaC independent of aldosterone. Aldosterone also activates Na + /K + -ATPase in the basolateral membrane of the principal cells of the collecting duct. Aldosterone, similar to Ang II, can act in an autocrine and paracrine manner. Aldosterone has been reported to be produced by aldosterone-producing cells other than the adrenal glomerulosa, such as neuronal glial cells and cardiac myocytes. Aldosterone can also stimulate NHE1 activity in the renal proximal tubule of spontaneously hypertensive (SHR), but not normotensive Wistar-Kyoto rats. Aldosterone and Ang II may act in ligand-independent ways to affect cell signaling, cell–cell communication, and growth. Some components of the RAS may have effects opposite to those of aldosterone and among the products of the RAS and their receptors. Although the AT 1 R can be stimulated by stretch, independent of Ang II, Ang II can have intracellular effects independent of the AT 1 R. The effects of the Ang II metabolites on sodium transport are described in the Ang II metabolites section.
Regulators of the Intrarenal Renin-Angiotensin System
The renal RAS is under tight control by a complex network of both positive and negative regulators. The positive regulators include Wnt/β-catenin and prostaglandin E2 receptor 4 pathways. The negative regulators included fibroblast growth factor 23/Klotho, vitamin D receptor, and liver X receptor.
Ontogeny
Development of the Renin-Angiotensin System: Structure of the Kidney and Urinary Tract
Postnatal Changes in Renin-Angiotensin-Aldosterone System Structure and Function in Humans
Studies in Humans
In humans, Ang-related genes are activated at stage 11 of the developing embryo, which corresponds to 23 to 24 days of gestation. AT 1 R and AT 2 R are expressed very early (24 days of gestation), indicating that Ang II may play a role in organogenesis. Whereas the AT 1 R is expressed in the glomeruli, the AT 2 R is found in the undifferentiated mesonephros that surrounds the primitive tubules and glomeruli. The AT 2 R is maximally expressed at about 8 weeks of gestation followed by decreasing but persistent expression until about 20 weeks of gestation. At stages 12 to 13, which corresponds to 25 to 30 days of gestation, angiotensinogen is expressed in the proximal part of the primitive tubule, and renin is expressed in glomeruli and juxtaglomerular arterioles. ACE is detected in the mesonephric tubules at stage 14. By 30 to 35 days, all components of the RAAS are expressed in the human embryonic mesonephros. Expression of these proteins in the future collecting system occurs later, at about 8 weeks of gestation.
ACE has a role in fetal growth and development. ACE inhibitor-induced fetopathy, consisting of oligohydramnios, intrauterine growth restriction (IUGR), hypocalvaria, renal dysplasia, anuria, and death, has been described in mothers exposed to ACE inhibitors during the second and third trimesters of pregnancy. These effects were initially believed to arise from a decrease in organ perfusion. More recent evidence indicates that ACE inhibition has teratogenic effects during development. Fetuses exposed to ACE inhibitors during the first trimester have an increased risk of congenital malformations, with an incidence of 7.1%, compared with those with no maternal exposure to antihypertensive medications during the same time period. The congenital anomalies are major and include cardiovascular defects, such as atrial septal defect, patent ductus arteriosus, ventricular septal defect, and pulmonary stenosis; skeletal malformations, including polydactyly, upper limb defects, and craniofacial anomalies; gastrointestinal malformations, such as pyloric stenosis and intestinal atresia; central nervous system malformations, such as hydrocephalus, spina bifida, and microcephaly; and genitourinary malformations, including renal malformations. Angiotensin receptor blockers (ARBs) have also been reported to be fetotoxic. Inhibition of the activity of the RAAS in pregnancy may have effects on the fetus that manifest later on in life, such as hypertension, which are addressed later.
The expression patterns of the RAS components in the embryonic kidney are similar in rodents and humans. In rodents, mutations of genes encoding renin, angiotensinogen, ACE, or the AT 1 R are associated with skull ossification defects, as well as autosomal recessive renal tubular dysgenesis. AT 1 R-deficient mice do not develop renal pelves. AT 2 R-deficient mice have congenital abnormalities of the kidney and urinary tract, such as multicystic dysplastic and aplastic kidneys. The more dramatic phenotypes seen in mice with deficient AT 2 R compared with humans with similar defects are attributed to the fact that human nephrogenesis is completed in utero, but morphogenesis of rodent nephrons continues for up to 10 days after birth. However, the phenotypes of AT 1 R gene (Agtr1/AGTR1) deficiency are similar in rodents and humans. As indicated earlier, mutations in Ang-related genes in humans are associated with renal tubular dysgenesis characterized by early onset and persistent fetal anuria leading to oligohydramnios and the Potter sequence. Whereas increased renin production is noted in the kidneys of patients with mutations in the genes encoding angiotensinogen, ACE, or AT 1 R, the effect of defects in the human renin gene (REN) on renin production is variable with loss-of-function mutation associated with absence of renin production, and REN missense mutations are associated with increased renin production. These studies demonstrate the importance of the RAAS in the development and maturation of the kidneys and collecting systems. The importance of the PRR in embryonic development has been discussed previously.
Postnatal Changes in Renin-Angiotensin-Aldosterone System Structure and Function in Humans
The RAAS is more active in the neonatal period and infancy than later in childhood. Plasma renin activity and plasma aldosterone levels are markedly increased in preterm human infants in the first 3 weeks of life. Although fetal ACE levels remain stable during gestation in rats, serum ACE activity has been reported by some to be higher in late fetal than in early neonatal life in humans, lambs, and guinea pigs. By contrast, renal ACE activity may increase with age, at least in pigs and horses. A similar pattern may be found in humans, based on the measurement of urine ACE isoforms. One study reported that plasma Ang II levels are similar among human infants with normal and low birth weights. However, at 7 days of life, plasma Ang II levels are markedly increased in very low birth weight infants. Small for gestational age boys (8–13 years) may have increased circulating Ang II and ACE activity relative to those with birth weights appropriate for gestational age. High serum aldosterone levels are seen at 2 hours of age and gradually decrease over the first year of life.
Unconventional Behavior of Renin-Angiotensin System Components
Although the canonical scheme of activation of the RAS components from renin to Ang II and its effects on Ang receptors is well recognized, recent evidence of other effects merits discussion. Both Ang I and Ang II may lead to effects that are independent of, or even antagonistic to, the accepted effects of the RAS. ACE may also function as a “receptor” that initiates intracellular signaling and influences gene expression. AT 1 R and AT 2 R have been shown to form heterodimers with other 7-transmembrane receptors and to influence signal transduction pathways with physiologic and pathologic consequences. For example, the high glucose-induced dimerization between adiponectin and AT 1 R leads to tubulointerstitial injury.
Intracellular Ang II affects cell communication, cell growth, and gene expression via the AT 1 R, but also has RAS-related independent effects.
Fetal Programming for Hypertension: Failure of Renoprotection?
The association of low birth weight with the development of hypertension later in life has been validated epidemiologically and, more recently, has been demonstrated experimentally in animal models. A suboptimal fetal environment may lead to maladaptive responses, including failure of renal autoregulation and the development of hypertension. A reduction in nephron number during development may contribute to a reduction in the glomerular filtration rate, but this is not always borne out in experimental studies. Multiple factors may contribute to the development of hypertension, but this chapter is restricted to the role of renal mechanisms. The RAAS may play a more important role than other factors because it is expressed early and is associated with nephrogenesis. Blockade of the AT 1 R during the nephrogenic period after birth in rats led to a decrease in nephron number, a reduction in renal function, and hypertension. The consequences of impairment of the RAS in utero and during development have been discussed earlier. Protein-restricted diets have also been shown to increase ACE levels in pregnant ewes. A general stimulation of all components of the systemic RAS, in response to protein restriction, is blocked by treatment with an ACE inhibitor or an ARB. Thus, the adverse environment in utero, which programs the fetus to develop hypertension, could be critically linked to abnormalities in the RAS. There are sex differences in fetal programming that may be related to hormonal milieu, which affect the RAS, with testosterone having a permissive effect and estrogen having a protective effect. A high fructose diet in pregnant Sprague-Dawley rats also increases blood pressure and aggravates the increase in blood pressure caused by a high-salt diet in male offspring that may involve the RAS. Other aspects of fetal programming and the effect on blood pressure have been recently reviewed.
Clinical Aspects
As discussed earlier, the development of the kidney occurs during the first 35 days postconception in humans. The integrity of the RAAS is essential for normal development, and Ang II is essential for normal structural development of the kidney and collecting system. The excretory function of the kidney begins soon after clamping of the umbilical cord at birth. It follows that the neonate is exquisitely sensitive to stressors, which activate the RAAS and lead to profound oligoanuric states. Disruption of the production or action of Ang II by genetic manipulation or pharmacologic blockade results in renal tubular agenesis and anemia in fetuses. More recently, the recognition of ACE2 as an important player in vasodilation by generation of alternative metabolites of Ang II has led to the discovery that blunting of the ACE2 effect is responsible for the postural orthostatic tachycardia syndrome—a syndrome of widespread vasoconstriction. This is probably the first human illness that is directly related to ACE2 and is applicable to the wider field of disordered vasoregulation, extending to fetal and neonatal life. The recognition of the importance of the PRR in hypertension has paved the way for the use of new antihypertensive drugs based on their ability to block the PRR. Ischemia and asphyxia generate renin by sympathetic stimulation. Drugs, such as furosemide, that increase distal delivery of sodium and water, decrease NKCC2 activity and should decrease renin secretion. However, decreased cotransporter activity impairs tubuloglomerular feedback and in the face of continued ion and water excretion may result in hypovolemia and activation of the RAS, which may result in oliguria. Indomethacin, and to a lesser extent ibuprofen, which is used to treat patent ductus arteriosus in neonates, could lead to decreased renal blood flow and renal insufficiency. Congenital abnormalities in steroid synthesis that lead to deficiencies in aldosterone production cause profound salt wasting. In type 1 pseudohypoaldosteronism, mutations in ENaC cause profound neonatal salt wasting. To counter the heightened activity of the RAAS in neonates, adenosine receptor antagonists may offer new avenues of therapy. The association between maternal ACE inhibition and renal abnormalities in fetuses has been described earlier in this chapter. Recent observations of increased fetal renin levels in hydronephrosis lend credence to the developmental significance of an intact RAAS in developing embryos. Preterm birth alters the development of the RAAS that can result in deleterious consequences. However, ACE2 may have a protective role in the heart and kidney.
In summary, the RAAS is an important developmental and physiologic system, which is involved in many aspects of renal function and blood pressure regulation. Moreover, drugs that interfere with the RAAS, given to developing fetuses, can program the development of renal abnormalities and hypertension. In addition to genetics, epigenetics, paternal and maternal nutrition, and health-related activities affect the development of the RAAS. A better understanding of these mechanisms would translate to better care for premature and full-term newborns with renal dysregulation, hyponatremia, and oligoanuric renal failure.
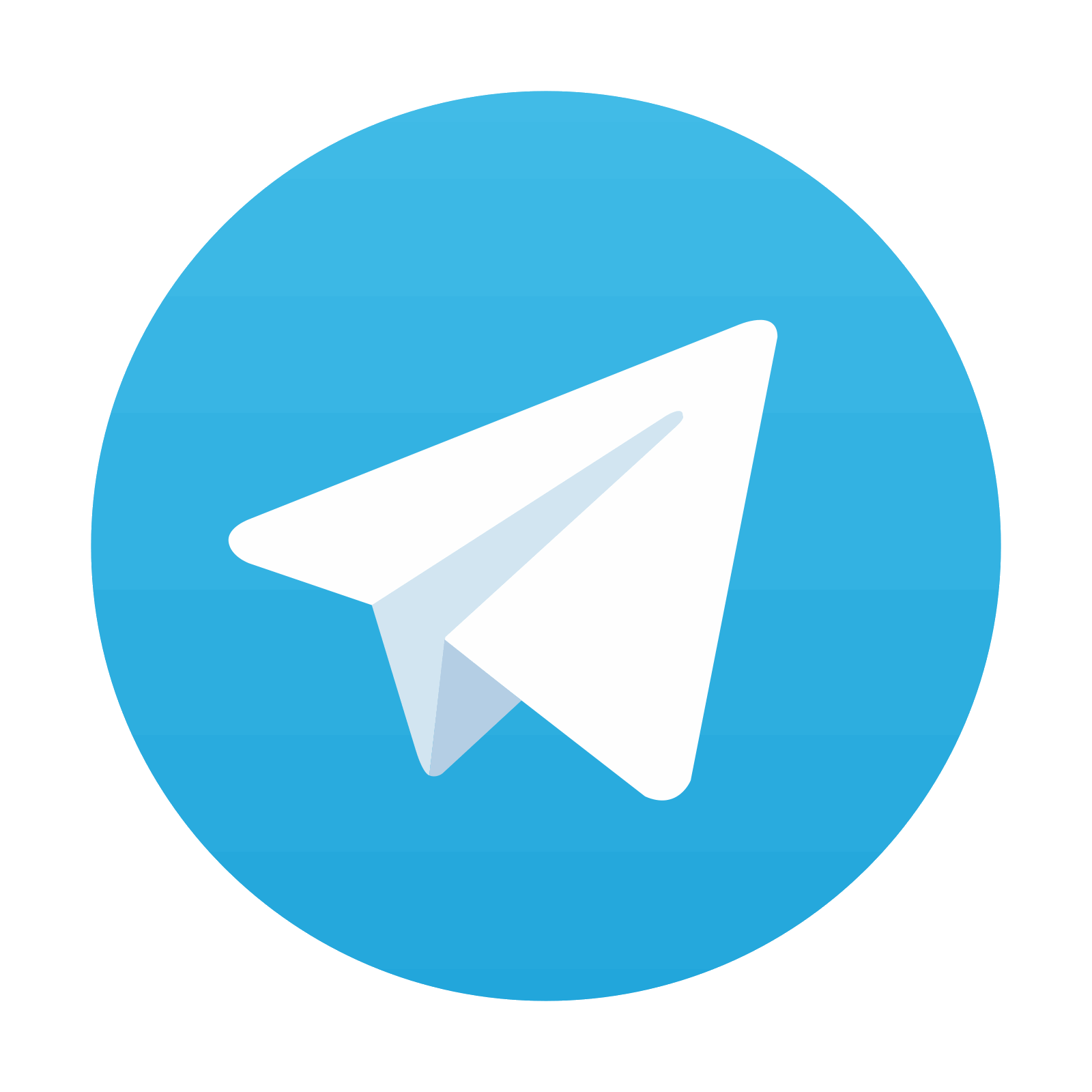
Stay updated, free articles. Join our Telegram channel
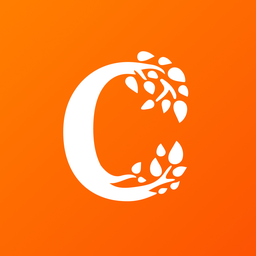
Full access? Get Clinical Tree
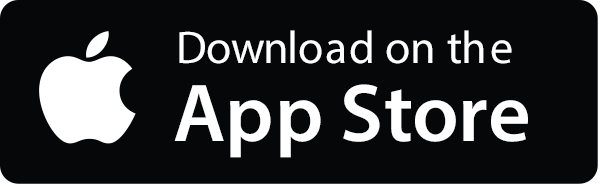
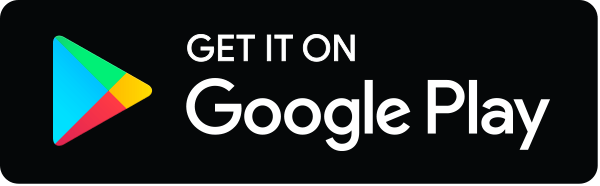