Abstract
The transition from a growing fetus to a newborn infant requires many changes in fluid and electrolyte homeostasis. During gestation, the placenta controls this balance, but once the fetus is born, the kidneys will perform the task of regulating the excretion of water, electrolytes, and solutes, as well as nitrogenous waste products. This chapter will briefly review the factors that regulate the placental exchange of fluid and sodium, as well as the renal developmental changes that occur throughout the growth of the fetus and the subsequent adaptation to life outside of the womb, with a focus on the postnatal development of renal tubular transport.
Keywords
sodium transport, proximal tubule, thick ascending limb of Henle, distal convuluted tubule, collecting duct, water transport
- •
Fluid Compartments
- •
Placenta and Amniotic Fluid
- •
Developmental Changes in Renal Function and Tubular Transport
- •
Renal Tubular Sodium Transport
- •
Proximal Tubule Sodium Transport
- •
Proximal Tubule Water Transport
- •
Development of Proximal Tubule Sodium Reabsorption
- •
Development of Proximal Tubule Water Permeability
- •
Thin Descending and Thin Ascending Loops of Henle
- •
Thick Ascending Limb of Henle
- •
Distal Convoluted Tubule
- •
Collecting Duct
- •
Developmental Changes in CCD Water Transport
The transition from a growing fetus to a newborn infant requires many changes in fluid and electrolyte homeostasis. During gestation, the placenta controls this balance, but once the fetus is born, the kidneys will perform the task of regulating the excretion of water, electrolytes, and solutes, as well as nitrogenous waste products. This chapter will briefly review the factors that regulate the placental exchange of fluid and sodium, as well as the renal developmental changes that occur throughout the growth of the fetus and the subsequent adaptation to life outside of the womb, with a focus on the postnatal development of renal tubular transport.
Fluid Compartments
The total body water content of the adult human is approximately 60% of its weight and is broadly divided into the intracellular fluid (ICF) and extracellular fluid (ECF) compartments. The proportion of the total body water in these two main compartments changes dramatically throughout fetal life and in the first year of postnatal life. In early fetal life the ECF is twice the size of the ICF, whereas in the adult the ICF is twice that of the ECF. The shift in the distribution of body water is primarily due to a decrease in the ECF after the fetus is born.
Placenta and Amniotic Fluid
During gestation, the fetus is dependent on the placenta to provide its nutrition, which includes the water and sodium necessary for amniotic fluid formation in the early part of gestation. The placenta has both active and passive mechanisms for the transfer of sodium from the mother to the fetus with water following its osmotic gradient. This includes several isoforms of water channels (aquaporins [AQPs]), as well as sodium channels and sodium hydrogen exchangers (NHEs). The driving force for the movement of sodium is provided by the sodium-potassium ATPase.
The average amount of amniotic fluid in the human is approximately 40 mL at 11 weeks of gestation and increases to a maximum of approximately 920 mL at term. During the first half of gestation, this fluid comes primarily from the placenta and has an osmolality that is isotonic to the fetus. However, as the fetal kidneys begin to produce urine, the osmolality of the amniotic fluid decreases to approximately 250 to 260 mOsm/L at term.
In addition to the sodium and fluid provided to the fetus, the placenta also provides all of the nutrition necessary for fetal growth. In particular, there are specific transporters for amino acids in the placenta that are necessary for protein synthesis. Because of the diversity of amino acids, there are several classes of amino acid transporters and they all appear to be present in the placenta. The importance of these transporters is emphasized by the fact that their expression is quite diminished in models of fetal growth retardation.
After the fetal kidneys begin making urine in the 10th week of gestation, the bulk of the amniotic fluid comprises urine from the fetus ( Fig. 4.1 ). Thus the amount of amniotic fluid in the second half of gestation is determined by the urine output. Conditions in the fetus that result in a high urine output lead to polyhydramnios, whereas conditions that limit the urine output will result in oligohydramnios.

One of the kidney diseases that results in polyhydramnios is Bartter syndrome, which is a genetic defect in the thick ascending limb (TAL) of Henle (discussed later). Although most forms of Bartter syndrome are chronic and lifelong, a transient form of Bartter syndrome has been described that is caused by mutations in the gene: melanoma-associated antigen D2 (MAGED-D2) that is found on the X chromosome. A number of patients had features of Bartter syndrome that improved with age; however, mutations were also found in a number of fetuses that were associated with polyhydramnios and fetal death. It is not clear how this gene defect affects the TAL and why it is transient in nature. These findings emphasize the role of the kidney and urine output in the formation of the amniotic fluid.
Decreased fetal renal function and urine output is associated with oligohydramnios. Severe forms of this sequence are known as Potter sequence and have characteristic facies, as well as clubfeet. The critical issue in these patients is that the lack of amniotic fluid leads to pulmonary hypoplasia, and many of these infants die from respiratory failure. These infants will generally not survive due to their respiratory failure.
Developmental Changes in Renal Function and Tubular Transport
As seen in Fig. 4.1 , the fetal kidney begins to function around the 10th to 11th week of gestation. At term, the kidney has developed its full complement of glomeruli that it will have as an adult. However, the function of the neonatal kidney continues to undergo development in terms of its ability to filter the blood (glomerular filtration rate [GFR]) and modify the filtered fluid (tubular function).
The GFR of a term neonate is only approximately 30% of the normal adult rate when factored for body surface area. The GFR increases during the first 12 to 18 months of life when the GFR is approximately the same as the adult when factored for body surface area. The glomerular ultrafiltrate is then modified by the renal tubules to eventually form the final urine. The tubules reabsorb a number of solutes, including sodium, bicarbonate, glucose, and amino acids, and also secrete a number of solutes, including potassium, acid, and ammonium. The excretion rate of the primary nitrogenous waste product, urea, is determined primarily by the filtration rate, which obligates a high rate of filtration to excrete urea and remain in nitrogen balance. This high filtration rate then obligates the renal tubules to reabsorb a large amount of sodium and fluid to remain in fluid balance.
The coordination between the GFR and tubule reabsorption rate is termed “glomerular tubular balance.” As will be discussed later, the neonatal tubules have much lower transport rates than the adult renal tubules. Because the developing neonate has a low GFR, the developing renal tubular reabsorption capacity is still able to meet the demand of the GFR. Thus the developing neonate is able to maintain glomerular tubular balance.
Renal Tubular Sodium Transport
The mature adult kidneys filter approximately 150 L of blood per day so that they can excrete nitrogenous waste in the form of urea. The renal tubules then reabsorb approximately 99% of the filtered load of fluid and solutes so that the final urine volume is on the order of 1 to 2 L per day. The key regulator of the reabsorbed fluid volume is sodium transport by the renal tubules.
Although sodium reabsorption occurs via a number of different transporters throughout the nephron, all of the active transport of sodium in the renal tubules is dependent on the sodium-potassium ATPase. This enzyme is located on the basolateral membrane of the cells throughout the nephron and uses energy from adenosine triphosphate (ATP) to maintain a low intracellular sodium concentration. There is an inwardly directed electrochemical gradient for sodium to enter the cell through the luminal membrane. The entry of sodium into the cell is driven by this low intracellular sodium concentration, and the energy of this electrochemical gradient can also be linked to other solutes for transport. As will be seen, the mechanism for entry of sodium through the luminal membrane varies throughout the different segments of the nephron.
Proximal Tubule Sodium Transport
In the proximal tubule the key transporter for luminal sodium entry into the cell is the sodium-hydrogen exchanger ( Fig. 4.2 ). The primary isoform of this transporter in the adult kidney is NHE3. By linking the sodium entry to proton secretion, this transporter will increase the intracellular pH of the proximal tubule so there will be a gradient for sodium and bicarbonate exit through the basolateral membrane via the sodium-bicarbonate cotransporter (NBC).

In the luminal fluid of the proximal tubule, the proton combines with the filtered bicarbonate to form carbonic acid that is then converted to water and carbon dioxide in the presence of carbonic anhydrase. Once the water and carbon dioxide enter the cell, they are converted back to carbonic acid that ionizes into protons and bicarbonate. The proton can then be secreted again, and the bicarbonate is transported through the basolateral membrane and into the bloodstream.
Because the processes involved in the reabsorption of bicarbonate have finite rates, the entire process exhibits saturation kinetics and has a transport maximum. When the filtered load of bicarbonate is below the transport maximum, all of the filtered bicarbonate will be reabsorbed. If the filtered load exceeds the transport maximum, some of the filtered bicarbonate will be excreted. Because bicarbonate is an anion, it must be excreted with a cation such as sodium or potassium. Thus bicarbonaturia can lead to volume depletion due to the sodium loss and also to hypokalemia.
Other sodium-coupled transporters on the luminal membrane include the sodium-glucose cotransporters (SGLT1 and SGLT2), sodium-phosphate cotransporters (NaPi2a), and sodium–amino acid cotransporters. (The transport of amino acids is very complex and is beyond the scope of this chapter. However, the simplistic view of the sodium–amino acid cotransporter is adequate for this discussion. Please see references and for further details.) These cotransporters account for only a small portion of the sodium that is reabsorbed but are responsible for reabsorbing almost the entire filtered load of glucose and amino acids. The amount of phosphate that is reabsorbed is dependent on the diet and can comprise a very wide range of the filtered load. Thus there is almost no glucose or amino acids in the final urine, but there can be considerable amounts of phosphate, depending on the dietary intake of phosphate.
Active, transcellular transport of sodium chloride occurs via the action of parallel transporters, NHE3, and the chloride-hydroxyl exchanger (see Fig. 4.2 ). As discussed previously, the sodium-hydrogen exchanger raises the intracellular pH of the proximal tubule. This leads to a pH gradient that can be used to exchange intracellular base (hydroxyl ions) for luminal chloride ions. The hydrogen ion that was secreted is then combined with the hydroxyl ion to form water while the sodium and chloride exit the cell via the basolateral membrane and into the bloodstream.
The actions of these transporters for the reabsorption of bicarbonate, glucose, and amino acids in the early proximal tubule will cause the luminal fluid to have a low bicarbonate concentration and a high chloride concentration ( Fig. 4.3 ). Thus the luminal fluid in the distal portion of the proximal tubule has a very low bicarbonate concentration, almost no glucose or amino acids, and a high concentration of chloride. The distal portion of the proximal tubule then uses the chloride concentration gradient to reabsorb salt and water by passive paracellular transport. The rate of reabsorption in this part of the nephron is dependent on the concentration gradient that was generated, as well as the paracellular permeability to chloride.

The expression of the intercellular junction proteins known as claudins determines the paracellular permeability of the proximal tubule. As will be discussed in the development of the proximal tubule transport, the expression of these claudins changes during development and has a direct impact on the rate of transport of sodium chloride.
Proximal Tubule Water Transport
The proximal tubule reabsorbs the bulk of the filtered load of sodium, chloride, and water in an isotonic fashion. The osmolality of the luminal fluid throughout the proximal tubule does not differ very much from the osmolality of the blood. This isotonicity is maintained because of the high expression of AQP1, the membrane water channel, in the luminal and basolateral membranes of the proximal tubule. Thus the water permeability of the proximal tubule is very high, and the osmotic movement of water can occur with a very small solute concentration gradient. As will be discussed later, other water channels are expressed throughout the nephron segments so that salt and water transport can be separated, but in the proximal tubule, there is no separation of salt and water.
Development of Proximal Tubule Sodium Reabsorption
The plasma bicarbonate concentration of neonates is lower than that of older children and adults ( Fig. 4.4 ). This was initially thought to be due to backleak of bicarbonate across the tubule epithelium that would lead to an excess excretion of bicarbonate. However, direct measurement of the epithelial permeability to bicarbonate demonstrated a lower permeability in neonatal rabbit proximal tubules compared to adult rabbit proximal tubules. Thus, it was clear that active transport of bicarbonate was lower in the neonatal tubule.

As the proximal tubule matures, all of the components of bicarbonate transport increase. The development of the Na + ,K + -ATPase activity parallels that of the basolateral surface area and provides the required energy for the secondary active secretion of protons through the luminal membrane. Thus the increase in the Na + ,K + -ATPase provides the necessary energy for transporting bicarbonate, as well as other filtered solutes such as glucose, phosphate, and amino acids ( Fig. 4.5 ).

The development of the NHE is more complex. The primary isoform of the luminal sodium-proton exchanger in the adult kidney is NHE3. Although the expression of NHE3 in neonatal rabbit proximal tubules was found to be very low, experiments demonstrated that there was sodium-hydrogen exchange activity that could not be explained by the negligible amount of NHE3 expressed. The isoform that was subsequently found to be responsible for this exchange activity in the neonatal tubules was NHE8. This isoform is also expressed in adult proximal tubules but is in intracellular compartments, whereas it is in the luminal membrane in the neonatal tubules. Thus there is evidence for an isoform switch for the sodium-proton exchanger throughout development.
Developmental expression of NHE3 and NHE8 is under hormonal control. A number of studies demonstrated that the expression of NHE3 is stimulated by glucocorticoids. Treatment with glucocorticoids also will cause the luminal expression of NHE8 to decrease. Thus glucocorticoids are probably one of the primary regulators of the development of transport of bicarbonate and sodium in the proximal tubule.
In addition to glucocorticoids, thyroid hormone has been shown to have a number of effects on the development of proximal tubular transport. Although thyroid hormone had some effect on the maturational increase in the expression of NHE3 and the decreased expression of NHE8, there were more significant effects on the paracellular transport of sodium chloride. The proximal straight tubules from hypothyroid neonatal rabbits were found to have a very low chloride permeability. Proximal straight tubules from hypothyroid animals that were treated with thyroid hormone had a chloride permeability that was not different from control animals. Thus the passive, paracellular transport in the proximal tubule also undergoes significant changes that appear to be affected by thyroid hormone.
The paracellular permeability properties of an epithelium are determined by the intercellular junction proteins known as claudins. The claudins expressed in the neonatal proximal tubule are different from the adult tubule, which leads to the very low permeability to chloride in the neonatal proximal tubule. Claudins 6, 9, and 13 were shown to have high expression in the neonatal tubules but not in the adult tubules. These claudins were shown in a cell culture model to have effects on chloride permeability. Thus the mechanism of sodium chloride transport in the late proximal tubule has significant maturational changes that are stimulated in part by thyroid hormone.
Development of Proximal Tubule Water Permeability
The active transport of sodium, chloride, and other solutes generates a small osmotic gradient across the proximal tubule. The high water permeability of this epithelium allows for high water transport rates in the presence of the low osmotic gradient. The high water permeability is due in part to the expression of AQP1 that is present in both the luminal and basolateral membranes of the tubule.
Expression of AQP1 in the proximal tubule of the neonatal rabbit kidney was found to be much lower than that of the adult proximal tubule. In addition, water permeability measurements of vesicles made from proximal tubule luminal and basolateral membranes showed that the water permeability of the membranes was much lower in the neonatal tissue than the adult kidney tissue. Thus it appeared that the water permeability of the neonatal proximal tubule should be much lower than the adult. However, direct measurements in isolated perfused rabbit proximal tubules showed that there was no difference in the water permeability between tubules from neonatal rabbits and adult rabbits. This was explained by the fact that the intracellular compartment in the neonatal tubules was much smaller than that of the adult tubules and provided less resistance to the movement of water through the epithelium. Thus, although the expression of AQP1 was lower in the neonatal proximal tubule, the epithelial water permeability remained high enough so that water could be reabsorbed.
Thin Descending and Thin Ascending Loops of Henle
Although transport of salt and water in the thin descending and ascending portions of the loop of Henle is primarily passive in nature, it is complex and its contribution to the medullary hypertonicity is incompletely understood. The osmolality of the medullary interstitium is higher than that of the luminal fluid so that there is an osmotic gradient for water reabsorption as the thin descending limb goes further into the medulla. Some, but not all, of the thin descending limbs of Henle express AQP1, so there is high water permeability in these segments. As water is reabsorbed, solute in the lumen becomes more concentrated so that when the fluid begins to ascend to the cortex, there is a gradient for passive sodium reabsorption in the thin ascending limb of the loop of Henle. Thus the reabsorption of water and sodium in the thin portion of the loop of Henle is passive and there is no separation of salt from water.
The developing fetus has only short thin limbs of Henle. Shortly before birth, they begin to form the long loops of Henle that will eventually contribute to medullary hypertonicity. The molecular signals controlling this process have only recently been studied. Defects in this process could contribute to the medullary hypoplasia seen in many forms of renal dysplasia.
Thick Ascending Limb of Henle
The TAL of Henle actively transports sodium from the lumen to the bloodstream in a way that also causes many other ions to be reabsorbed ( Fig. 4.6 ). As in the proximal tubule, the primary active transporter is the sodium-potassium ATPase located in the basolateral membrane of the cell that maintains a very low intracellular sodium concentration. The sodium-potassium-2 chloride cotransporter (NKCC2) uses this sodium concentration gradient to transport sodium, potassium, and chloride ions into the cell. This transporter is electroneutral and thus does not directly affect the luminal membrane electric potential.

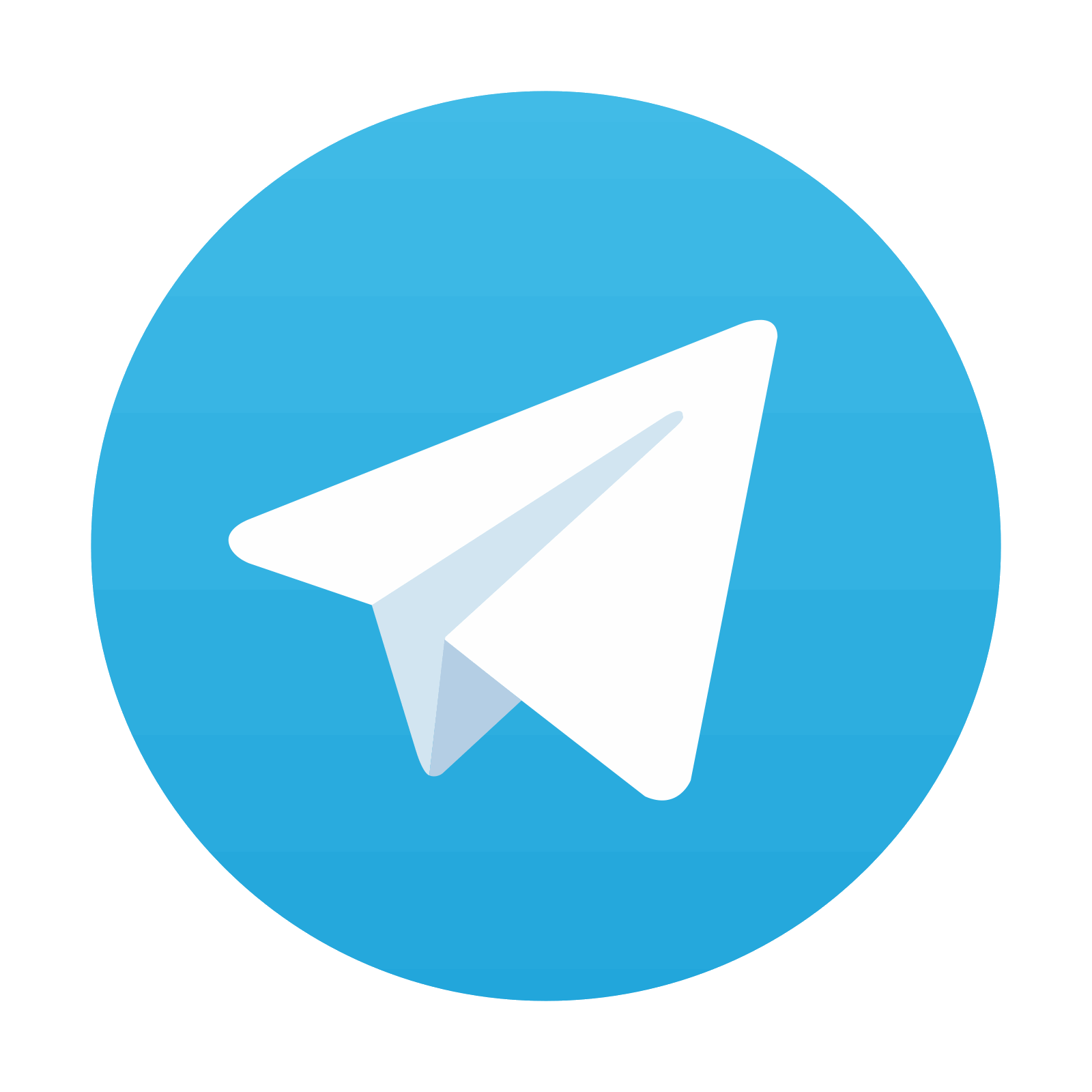
Stay updated, free articles. Join our Telegram channel
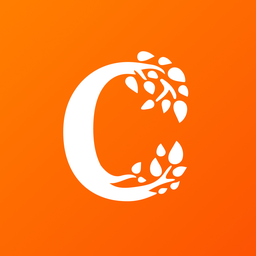
Full access? Get Clinical Tree
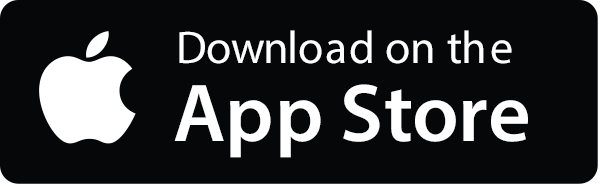
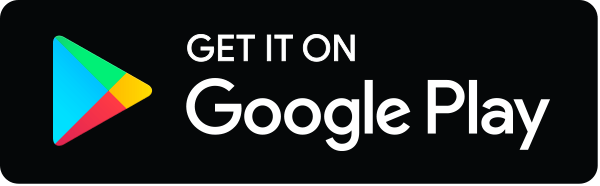
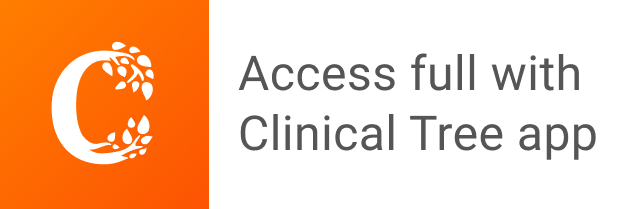