Abstract
Before birth, the ability of the organism to conserve salt and water is not critical as the placenta controls fluid balance in the fetus. The event of birth prompts abrupt changes in the infant’s environment, rendering the newborn responsible for maintaining fluid and electrolyte homeostasis. Although nephrogenesis in full-term human infants is completed before birth, tubular development continues during the first few years of life, limiting the kidney’s ability to regulate sodium and water excretion. The renal response to arginine vasopressin (AVP) and atrial natriuretic peptide (ANP), two hormones with a major role in sodium and excretion, is developmentally regulated. Although infants have the ability to secrete AVP in response to various stimuli, the response of the immature collecting duct is significantly attenuated. Conversely, circulating ANP levels are decreased in immature mammals due to enhanced clearance. We discuss the developmental regulation of these hormones and potential clinical consequences during the neonatal period.
Keywords
arginine vasopressin, atrial natriuretic peptide, water absorption, sodium excretion, developmental regulation, aquaporins
- •
Arginine Vasopressin
- •
Atrial Natriuretic Peptide
Introduction
Total body fluid homeostasis is vital for human survival. Before birth, fetal mechanisms to conserve fluid are not essential because the placenta has a major role maintaining fluid homeostasis. At birth, the newborn becomes responsible for keeping homeostasis while facing dramatic environmental changes; therefore, the kidneys assume a central role maintaining fluid homeostasis. Despite having completed nephrogenesis by 36 weeks of postmenstrual age, renal tubular function is still immature at birth, limiting the newborn’s ability to handle water and sodium.
Understanding the developmental changes and mechanisms involved in fluid homeostasis events is crucial to manage newborns and infants. We review the role and development of two hormonal pathways that regulate the body fluid composition during the prenatal and perinatal periods: (1) arginine vasopressin (AVP) system, which has a major role modulating water homeostasis, and (2) atrial natriuretic peptide (ANP) system, which has a major role in sodium excretion.
Arginine Vasopressin
Arginine Vasopressin Synthesis and Secretion
Arginine vasopressin is synthesized in the cell bodies of magnocellular neurons that originate in the supraoptic and paraventricular nuclei of the hypothalamus and is stored in neurosecretory granules in their posterior pituitary nerve terminals ( Table 12.1 ).
Arginine Vasopressin | Atrial Natriuretic Peptide | |
---|---|---|
Stimuli |
|
|
Hormone Synthesis Site | Magnocellular cells at the supraoptic and paraventricular nuclei of the hypothalamus | Atrial cardiomyocyte |
Hormone Storage and Secretion | Neurosecretory granules at the posterior pituitary | Atrial cardiomyocyte |
Hormone Receptor | Vasopressin 2 receptor (V 2 R) | Type A natriuretic peptide (NPR-A) |
Effector Cells | Principal cell at the collecting duct (V 2 R locates on the basolateral membrane) |
|
Intracellular Signaling |
|
|
Final Effect | Insertion of AQP2 into the apical membrane of principal cells, increasing water permeability |
|
Hormone Degradation | Phosphodiesterases | Type C natriuretic peptide (NPR-C)—“clearance receptors” |
When the AVP stimuli-secretion arises, voltage-gated calcium channels in the magnocellular nerve terminals open, transient calcium influx occurs, neurosecretory granules fuse with magnocellular neuron membranes, and AVP is released into the systemic circulation. This secretion is triggered by multiple physiologic and pathophysiologic stimuli. Changes in plasma osmolality and changes in effective circulating blood volume are the main triggers for AVP secretion (see Table 12.1 ). Under normal physiologic conditions, a rise in plasma osmolality, as minimal as 2 to 5 mOsm/kg H 2 O, is sensed by the osmoreceptors in the hypothalamus and is the main stimulus of AVP secretion. Conversely, changes in effective circulating volume become important stimuli for AVP secretion when the decrease in the effective circulating blood volume is greater than 8% to 10%. Dunn et al. displayed this relationship in their experiments where male Sprague-Dawley rats were exposed to three different experimental scenarios: (1) fluid restriction (hypertonic hypovolemia—leading to both a rise in plasma osmolality and a decrease in blood volume), (2) intraperitoneal injections of hypertonic saline (hypertonic isovolemia—leading to only a rise in plasma osmolality), and (3) intraperitoneal injections of polyethylene glycol (isotonic hypovolemia—leading to a decrease in effective circulating volume only). When rats were exposed to intraperitoneal injections of hypertonic saline, a positive linear correlation was observed between a rise in plasma osmolality and a rise in AVP ( r > 0.9). Conversely, when rats were exposed to intraperitoneal polyethylene glycol an exponential, rather than linear, rise in AVP became apparent when volume had fallen by 8% to 10%. When rats were exposed to fluid restriction, an initial twofold to threefold linear rise in plasma AVP levels was observed, similar to the hypertonic isovolemia scenario, which was later followed by a 10-fold rise when additional blood volume had been lost, similar to the hypertonic hypovolemia scenario. Earlier experiments by Johnson et al. reported similar results in sheep models.
Other stimuli for AVP secretion in newborns include pain, thermic stress, hypoxia, hypoglycemia, hydrocephalus, intracranial hemorrhage, and drugs (see Table 12.1 ).
Development
During fetal life, AVP is detectable in the human pituitary as early as at 11 to 12 weeks of gestational age, rapidly increasing thereafter until the second trimester of pregnancy ( Table 12.2 ). Vasopressin neurons in an adultlike organization are observed early during fetal life, with an increase in number of vasopressin neurons until the 26th week of gestational age, along with vasopressin gene expression.
Arginine Vasopressin | Atrial Natriuretic Peptide | |
---|---|---|
Is hormone secreted during fetal period? | Yes | Yes |
Is there a main renal role of this hormone during fetal period? | No | No |
What are the main stimuli for hormone secretion in the perinatal period? | Hypoxia and increased intracranial pressure | Atrial wall stretch due to decreased pulmonary vascular resistance and increased left atrial venous return |
Reasons for immature renal response to hormone at birth |
|
|
Similarly, during fetal life, a rise in plasma maternal and fetal osmolality leads to AVP secretion. In chronically catheterized sheep model, Weitzman et al. showed that pituitary AVP secretion is stimulated by hypertonic saline infusion during fetal life. Likewise, in fetal ewes, increasing maternal plasma osmolality either by water deprivation or by mannitol infusion resulted in higher fetal AVP serum concentrations. Angiotensin II is another stimulator of AVP secretion. In ovine fetuses, angiotensin II-induced fetal AVP secretion has been shown to be mediated through stimulation of angiotensin receptor 1.
In the perinatal period, the response to the hypoxia and increased intracranial pressure induced by labor has been proposed as another stimulus of AVP release (see Table 12.2 ). This is supported by studies demonstrating higher AVP levels in the human umbilical cord of infants delivered vaginally than infants delivered without labor.
Vasopressin Signal Transduction
Arginine vasopressin exerts its antidiuretic action by binding to the V 2 vasopressin receptor (V 2 R), a G-stimulatory (G s ) protein coupled receptor on the basolateral membrane of renal collecting duct principal epithelial cells (see Table 12.1 ). The V 2 R has seven transmembrane domains, with a groove for antidiuretic hormone (ADH) binding in the extracellular region and a binding site for a G S protein in its intracellular domain. Once AVP binds to V 2 R, an intracellular adenylate cyclase second messenger system gets activated. Intracellular cyclic adenosine monophosphate (cAMP) increases and activates protein kinase A, which then phosphorylates the cytoplasmic carboxy-terminal end of water channel aquaporin 2 (AQP2), resulting in the insertion of this water channel into the apical membrane of the principal cell and subsequent increased water permeability. Once cAMP is generated, it is promptly degraded by phosphodiesterase (see Table 12.1 ).
Development
Despite the documented presence of AVP during fetal life, the immature kidneys have poor response to AVP. In ovine models, AVP infusion did not change fetal plasma osmolality. In humans, Heller administered pituitary extract containing AVP to newborn infants and adult controls. In adults, a rapid increment in urine osmolality was observed, while in infants, urine concentration remained unchanged. These findings support poor response of AVP by immature kidneys.
Multiple lines of evidence suggest that this poor response of immature kidneys to AVP is related to resistance to AVP rather than to lack of receptors (see Table 12.2 ). Otrowski et al. showed that V 2 R is expressed in rats at 16 days of gestation in the developing medullary and cortical collecting duct cells. After birth, V 2 R was also observed in the cells of thick ascending limbs of loop of Henle, papillary epithelium, macula densa, and short distal nephron segments. Similarly, Rajerison and coworkers also demonstrated that AVP binding sites are present on medullopapillary membranes harvested from neonatal rat kidneys. These data suggest that V 2 R is present early in gestation, making lack of V 2 R a less likely cause of poor response of immature kidneys to AVP.
On the other hand, an immature intracellular secondary messenger system has been implicated in the AVP resistance and, consequently, a reduced urine concentrating ability in neonates compared to adults (see Table 12.2 ). With regard to the development of the intracellular secondary messenger system, studies have shown that adenylyl cyclase response and cAMP generation are both markedly reduced in the neonatal period and that AVP-stimulated urine concentrating ability develops in parallel with cAMP generation. To characterize the mechanisms contributing to this low cAMP generation, we measured V 2 R-independent stimulation of adenylyl cyclase in the immature cortical collecting ducts of rabbits using direct adenylyl cyclase stimulators (NaF and foskolin). Interestingly, maximal cAMP generation was not elicited. At the same time, when isolated microperfused immature cortical collecting ducts of rabbits were exposed to a cAMP analog, 8-chlorophenylthiol-cAMP, water permeability was not increased to the levels observed with AVP in mature cortical collecting ducts. These findings suggest that the diminished AVP response is due, at least in part, to factors distal to cAMP generation.
Degradation of cAMP has a prominent role in the blunted response of immature collecting duct to AVP (see Table 12.2 ). In newborn rat renal homogenates, high levels of cAMP phosphodiesterase activity have been reported. In neonatal cortical collecting ducts, the administration of rolipram, a phosphodiesterase intravenous (IV) inhibitor, not only diminished phosphodiesterase activity, but most importantly, eliminated the blunted AVP-stimulated water permeability usually observed in immature cortical collecting ducts. Taken together, these findings suggest that phosphodiesterases have an important role inhibiting AVP response in the immature collecting duct.
High prostaglandin activity has also been implicated in the diminished response of immature collecting duct to AVP (see Table 12.2 ). In fetal lambs, high urinary levels of prostaglandin have been reported, and in piglets, inhibition of prostaglandin activity with indomethacin led to increased urine osmolality and cAMP excretion. Urinary excretion of prostaglandin E 2 (PGE 2 ) has been inconsistent in human newborns. Sulyok and colleagues reported low urinary PGE 2 levels in preterms that increase during first weeks of life, while Joppich and colleagues reported higher levels of PGE 2 in preterm than in full-term neonates. Joppich and colleagues also observed increasing urine osmolality and cAMP excretion with decreasing PGE 2 in full-terms.
However, prostaglandin interactions with AVP and cAMP are complex and may lead to both stimulatory and inhibitory water reabsorption responses by the collecting duct. PGE 2 alone stimulates cAMP generation and leads to enhanced water permeability. Conversely, in the presence of AVP, PGE 2 blunts AVP-stimulated cAMP generation and water reabsorption. Notably, in the presence of a direct adenylyl cyclase stimulator or cAMP analog, no inhibitory effect was observed. These findings suggest that PGE 2 decreases AVP-stimulated water reabsorption by blunting cAMP generation. Pretreatment of cortical collecting ducts with indomethacin, a cyclooxygenase inhibitor, resulted in AVP-mediated cAMP generation in neonatal cortical collecting ducts to levels similar to mature cortical collecting ducts. Notably, when cortical collecting ducts were incubated in pertussis toxin, an inhibitor of G inhibitor protein (G i ), similar results were reported. Finally, when immature collecting ducts were incubated with indomethacin and pertussis toxin, no additive effect was observed. Altogether, these findings suggest that prostaglandin may contribute, at least in part, to diminished AVP-stimulated water reabsorption via the activation of G i and subsequent blunting of cAMP generation on an immature cortical collecting duct.
Prostaglandin EP 3 subtype receptor has been proposed as the major site for prostaglandin inhibitory effects on cAMP generation. EP 3 receptor has been localized in the cortical collecting ducts and is coupled to G i , with PGE 2 inhibiting cAMP generation via EP 3 receptor/G i activation. EP 3 receptor expression is developmentally regulated with higher levels in the first 2 weeks of life in immature collecting ducts followed by a rapid decrease to adult levels afterwards. This could explain the observation in newborn rats that renal cortex has higher affinity for PGE 2 than the adult cortex.
In contrast, studies in chronically catheterized fetal sheep failed to show increased urine osmolality after indomethacin administration. Similarly, in vitro microperfusion of isolated rabbit tubules did not lead to maximal AVP-stimulated water reabsorption when tubules were preincubated with indomethacin. Steps distal to cAMP generation in the AVP signaling pathway, which are not mediated by prostaglandins, contributing to this diminished AVP-stimulated water reabsorption (i.e., cAMP degradation by phosphodiesterases) could potentially explain these results.
Aquaporins: Water Channels
Aquaporins are 26 to 34 kD glycosylated proteins sharing 50% to 85% homology containing six transmembrane-spanning domains organized in homotetramers. There are several aquaporin isoforms that differ in their water transport properties, solute selectivity, and tissue expression; and at least seven AQP isoforms are expressed at different sites in the kidney. AQP2 is expressed in the principal cells of the collecting tubules. It is the main target for AVP-mediated water transport (see Table 12.1 ). AQP3 and AQP4 are expressed in the basolateral membrane of collecting duct principal cells in the cortical and medullary collecting duct, respectively, and have a role in the movement of water from the principal cell to the plasma. Water enters the principal cell via apical AQP2 and leaves through AQP3 and AQP4 located on the basolateral membrane. AQP1 is constitutively expressed on the apical and basolateral membranes of water-permeable proximal tubular cell and descending loop of Henle. It is also expressed in the descending vasa recta capillaries. Lack of AQP1 in the collecting duct suggests that it does not participate in the AVP-mediated water reabsorption. AQP7 is expressed on the apical membrane of the distal portion of the proximal tubule (the S3 segment), suggesting that AQP7 has a role in proximal tubule water absorption. AQP6 is expressed in the type A intercalated cells of the collecting duct, exclusively in the intracellular space with no expression on the plasma membrane. Yasui and colleagues proposed that AQP6 may have a role in vesicle acidification; however, studies are required to further elucidate its role. AQP8 is expressed in low amounts in proximal tubules and collecting duct principal cells, but its functions remain to be elucidated.
Development
AQP2 expression has also been implicated as a contributor to the limited urine concentration ability during the first year of life (see Table 12.2 ). In rats, AQP2 expression was low at birth but steadily increased to maximal expression at 10 weeks of age in parallel with increased urine concentrating ability. When immature rats were water deprived, AQP2 expression increased to adult levels, but this was not accompanied by a proportional increase in urine osmolality. In preterm and full-term newborns, urinary excretion of AQP2, a marker of AVP activation in the collecting duct, was similar to adults’ levels and was not correlated with changes in urine concentrating ability. These findings suggest that although AQP2 expression may contribute to the reduced urine concentrating ability in the neonate, it is probably not a major limiting factor. The expression of AQP1, AQP3, and AQP4 increases progressively during fetal life, but they do not have major changes in expression after birth; thus, these AQPs do not seem to limit water reabsorption by the neonatal kidneys.
Aquaporins also seem to play an important role in the transcellular solute-free water flow through the placenta. Four different types of AQPs have been identified (AQPs 1, 3, 8, and 9) in the placenta. Aquaporin 3, primarily expressed in the apical membranes of syncytiotrophoblasts, appears to have an important role regulating placental water flux during gestation, with its expression changing throughout gestation. This is supported by studies in mouse models showing increased AQP3 expression along with placental permeability to water early in fetal life followed by a decrease in AQP3 expression and placental water permeability at the end of gestation. Aquaporin 1, primarily expressed in the fetal chorioamnion, has been implicated in the water flux between the fetus and the amniotic fluid. Supporting this role is the observation that there is significant polyhydramnios in AQP1 knockout mouse models. Aquaporin 1 also has a variable expression during gestation with higher expression early in fetal life, followed by a decrease and rise again near term. Aquaporin 8 is expressed in trophoblast epithelial cells, and AQP9 is expressed in the amnion and allantoin; their roles in placental water exchange remain to be elucidated.
Medullary Interstitium Tonicity
Finally, relatively low interstitial sodium chloride and urea concentration and anatomically shorter loops of Henle have been implicated in the reduced urinary concentrating ability observed in immature kidneys (see Table 12.2 ). Immature salt reabsorption machinery in the thick ascending limb of Henle and urea recycling mechanisms lead to low medullary tonicity, which limits water movement across collecting ducts. Likewise, low dietary protein intake may have a limiting role in urea generation. Shorter loops of Henle limit the performance of the countercurrent system. With renal growth after birth, loop length increases progressively.
Atrial Natriuretic Peptide
Atrial Natriuretic Peptide Synthesis and Secretion
Atrial natriuretic peptide (ANP) is mostly synthesized in the atrial cardiomyocytes as a 151-amino acid pre-pro-hormone (pre-pro-ANP) and stored as a 126-amino acid prohormone in secretory granules. ANP is also synthesized in cardiac ventricles and hypothalamus but at lower levels than in atrial cardiomyocytes. To become active ANP, pro-ANP undergoes a proteolytic cleavage by corin, a transmembrane trypsinlike serine protease, which is also very abundant in myocytes.
Atrial natriuretic peptide is continuously secreted in the atria, but its synthesis and secretion are modulated by stretch-mediated and neurohumoral-mediated factors (see Table 12.1 ). Atrial wall stretching, caused by changes in central venous return and atrial chamber dilation, is the main stimulus for ANP secretion. This is supported by early studies by Lang and coworkers showing that increasing atrial pressure of isolated rat heart led to ANP secretion. Similarly, they showed that ANP plasma levels increased in anesthetized rats exposed to volume expansion. Likewise, anesthetized dogs with atrial distention secondary to mitral valve obstruction also had increased ANP levels. In humans, a rise in central venous pressure (i.e., right atrial pressure) of 1 mm Hg leads to a 10 to 15 pg/mL increase in circulating ANP.
Hypoxia is another potent stimulus for ANP secretion (see Table 12.1 ). In isolated hearts from rabbits and rats exposed to hypoxia, increased ANP has been documented, with longer hypoxia periods leading to higher ANP levels. Once tissues are reoxygenated, ANP levels return to baseline. In healthy humans exposed to moderate acute hypoxemia by being exposed to a gas mixture containing 10% oxygen, 4.5% CO 2 , and 85.5% N 2 resulted in an increase in ANP from 17.7 +/− 3.4 to 27.2 +/− 1.7 pg/mL. Also, in animal models and humans, myocardial infarction induced cardiac ischemia leads to significant increment in ANP release. The mechanisms implicated in this hypoxia-induced response include alpha- and beta-adrenergic as well as endothelin stimulation. Studies in Langendorff-perfused rat hearts exposed to hypoxia showed that treatment with phentolamine and propranolol reduced atrial natriuretic factor (ANF) release by half, suggesting a partial contribution of alpha- and beta-adrenergic stimulation to hypoxia-induced ANP release. On the other hand, supporting endothelin-mediated ANP release in the setting of ischemia, Skvorak and coworkers showed that isolated rat atria exposed to anoxia present significant increments in ANP secretion, fully reversible after normal oxygen levels are achieved. Moreover, treatment with an endothelin-1 receptor agonist, BQ123, blocks ANP secretion in this scenario.
Vasoconstrictor hormones such as angiotensin II and vasopressin have also been implicated as stimuli for ANP secretion (see Table 12.1 ). However, it is yet unclear whether they directly affect ANP secretion or indirectly affect it by causing vasoconstriction and increased venous return. ANP secretion is stimulated by angiotensin II in isolated rabbit hearts and in atrial appendages excised from fresh rat hearts but not in rat heart-lung circuits. In vivo experiments in anesthetized rats that received angiotensin II led to changes in cardiac atrial pressure and increments in ANP release. In addition, other experiments in rats exposed to angiotensin at doses that do not affect atrial pressures also led to increased ANP levels. Experiments with AVP in vitro have shown contradictory results. Rat atria incubated in AVP showed ANP secretion. However, in isolated rat hearts, ANP secretion was inhibited by AVP. In isolated rat heart-lung circuit, no increments in ANP secretion were observed when exposed to AVP or angiotensin.
As with other hormonal secretory processes, the ANP secretory process involves four steps: (1) signal transduction at the cell membrane, (2) integration of several intracellular messengers, (3) hormone packing, trafficking, and release, and (4) extracellular fluid transport.
Once atrial cardiomyocytes receive the initial stimuli by atrial stretch or endothelin 1 (or other potential neurohumoral factors) via the stretch activated ion channels and/or endothelin receptor (other neuroendocrine hormone receptors), respectively, G proteins serve as transducers of ANP secretion (see Table 12.1 ). There are two different G proteins serving as transducers in the atrial myocytes: (1) Gi/o-mediated, pertussis toxin sensitive and (2) Gq-mediated, pertussis toxin insensitive. Atrial stretch caused by central venous return changes, as seen with extracellular fluid volume expansion, is regulated by Gi/o-mediated stretch-stimulated, pertussis toxin sensitive mechanism, while ANP secretion at basal state and stimulated by neuroendocrine factors is regulated by the Gq-mediated, pertussis toxin insensitive protein.
Calcium and cyclic nucleotides play important roles in the secretion processes of multiple hormones. For ANP secretion, the role of calcium seems to be complex. Calcium channel agonists increase ANP secretion in atrial myocyte cultures, but they inhibit ANP secretion in isolated rat hearts and rabbit atria. Inhibition of calcium release from the sarcoplasmic reticulum, either with ryanodine or thapsigargin, inhibited stretch-stimulated ANP secretion in atrial myocyte cultures and isolated rat atria, but not the ANP basal secretion. Calcium depletion decreases endothelin-mediated ANP secretion in myocytes cultures. Taken together, these findings suggest that calcium may have a positive modulatory role under conditions of stretch-mediated or neurohumoral-mediated stimulation. The cyclic nucleotides (cAMP and cGMP) seem to have an inhibitory effect on ANP secretion. This is supported by evidence showing that cAMP stimulators, such as forskolin, phosphodiesterase inhibitors, and adrenomedullin, as well as cGMP generation inhibit ANP secretion.
Glucocorticoids, mineralocorticoids, and prostaglandins have also been implicated in the stimulus of ANP secretion (see Table 12.1 ). Glucocorticoids and mineralocorticoids upregulate ANP gene expression in rat myocytes increasing ANP production. On the other hand, PGF2 alpha stimulates synthesis and secretion of ANP, which is mediated by stretch-induced ANP secretion. This is supported by experiments in rats and rat atrial myocyte cultures showing that atrial stretch and PGF2 alpha led to ANP secretion, but the effect of the two together was not greater than the effect of the individual stimuli. Also, prostaglandin synthesis inhibition with indomethacin led to reduction of basal ANP secretion as well as hypoxia/endothelin-1 stimulated ANP secretion. Taken together, these findings suggest an important role of prostaglandins, glucocorticoids, and mineralocorticoids mediating ANP synthesis and secretion.
Development
During pregnancy, ANP has been proposed to play a major role in the regulation of vascular tone, with maternal ANP levels steadily increasing at 12 weeks of gestation followed by a decline postpartum. In the human placenta, significant ANP expression has been demonstrated in extravillous trophoblasts and, to a lesser degree, in decidual cells, suggesting local placental synthesis. However, in mouse placenta, ANP synthesis remains controversial. Importantly, ANP does not seem to cross placenta, as evidenced by experiments in pregnant rats, where no active or passive transport of radiolabeled ANP across the placenta was observed.
Atrial natriuretic peptide is secreted during fetal life, with ANP mRNA being detected as early as 8.5 days of gestation in rat embryos (see Table 12.2 ). Importantly, in humans and rats, ANP is synthesized in the ventricular cardiomyocytes, while it is synthesized in the atrial cardiomyocytes in ovine fetus. These findings are important when extrapolating animal data to humans where ANP release stimulating mechanisms may not be the same in different species. ANP secretion does not seem to be related to maternal ANP concentrations. Another important aspect of fetal ANP secretion is that fetal ANP levels during pregnancy are higher than maternal levels. The cause for higher fetal than maternal ANP levels is not clear.
Mid- and late-gestation animals can increase ANP levels in response to various stimuli, including angiotensin II infusion, AVP infusion, indomethacin treatment, acute fetal volume expansion, hypertonicity, and fetal hypoxia. However, ANP probably has a small role in renal homeostasis and fluid balance during intrauterine life, which is chiefly ensured by the placenta. In general, supraphysiologic ANP doses infused to fetal animals leads to lower arterial blood pressure and a moderate diuretic effect, but there is not a significant increase in natriuresis.
At birth, loss of the placental circulation produces dramatic hemodynamic changes such as decreased pulmonary vascular resistance and increased left atrial venous return, leading to atrial distension stimulating ANP secretion from myocardial cells (see Table 12.2 ). In humans, plasma ANP levels are elevated during the first days of life, with peak levels on days 1 to 2 of life, which then decrease by the second week of life. This pattern parallels the postnatal diuresis period, leading to extracellular space contraction seen in infants, which is then followed by the sodium retention period for somatic growth.
Atrial Natriuretic Peptide Signal Transduction
Atrial natriuretic peptide exerts its action in the kidney via activation of a type A natriuretic peptide receptor (NPR-A) (see Table 12.1 ). This receptor has (a) on the extracellular aspect: a glycosylated domain where ANP binds, (b) single transmembrane segment, (c) on the intracellular aspect: a noncatalytic, kinaselike, ATP-binding domain, a hinge region, and a guanylyl cyclase domain (the latter is the catalytic effector of the receptor). Two peptide receptor chains, dimerized via a disulfide bridge, are required to activate the function of NPR-A. When ANP binds the extracellular domain, the intracellular kinaselike domain undergoes a conformational change leading to relaxation of guanylyl cyclase tonic inhibition, increasing cytosolic cyclic guanosine monophosphate (cGMP) production. cGMP in turn activates cGMP-gated channels, cGMP-dependent phosphodiesterases, and the cGMP-dependent protein kinases cGKI and cGKII, as will be discussed below (see Table 12.1 ).
In addition to the kidneys, NPR-A is also expressed in the heart, lungs, adrenal glands, adipose tissue, and eyes. In the kidneys, the NPR-A receptor is expressed throughout the nephron (glomerular cells and tubular epithelial cells). In the glomerulus, NPR-A receptors are located on the surface of the podocytes and mesangial cells. Glomerular podocytes respond to ANP stimulation by upregulating their cGMP synthesis (see Table 12.1 ). However, the role of ANP-induced cGMP production in podocytes remains unclear. Experiments in isolated rat glomeruli suggest that F-actin filaments may be reorganized, leading to cellular relaxation and modulation in filtration characteristics. In mesangial cells, ANP-induced cGMP production, via NPR-A, increases hyperpolarization leading to cellular relaxation and increases ultrafiltration associated with increased glomerular hydrostatic pressure and filtration rate (see Table 12.1 ). ANP also increases glomerular filtration rate and glomerular permeability by promoting vasodilatation of the afferent arteriole and vasoconstriction of the efferent arteriole and by causing a direct, reversible increment of the radius and number of large pores, respectively.
In tubular cells, ANP inhibits the basolateral Na-K ATPase through type II cGMP-dependent protein kinase (PKGII) induced phosphorylation. In proximal tubule cells, ANP inhibits Na reabsorption without altering glomerular filtration rate (GFR) by inhibiting Na-dependent transport systems (see Table 12.1 ). Potassium, chloride, and organic acid transporters in the proximal tubule are also regulated by ANP. In the thick ascending loop cells, ANP inhibits chloride transport in a cGMP-dependent manner by directly inhibiting Na-K-2Cl (NKCC) cotransporter activity, and this, in turn, leads to decreased urine concentrating capacity and increased urine flow (see Table 12.1 ). Medullary collecting duct cells are the main site of ANP action. In these cells, ANP promotes sodium excretion by inhibiting cyclic nucleotide-gated (CNG) cation channels including the epithelial sodium channel designated ENaC (see Table 12.1 ). Furthermore, ANP has been shown to inhibit water permeability by 40% to 50% in non–AVP-stimulated renal collecting ducts.
NPR-A stimulation also regulates blood pressure by inducing vasodilatation, increased endothelial permeability, and inhibition of the sympathetic system. This is supported by evidence showing that targeted deletions of ANP or NPR-A gene in transgenic animals induce severe arterial hypertension, while hypotension is observed with overexpression of these genes.
ANP is also ligand for NPR-C, “clearance receptor.” This receptor has (1) on the extracellular aspect, a domain where ANP binds, (2) a single transmembrane segment, and (3) on the intracellular aspect, a 37-amino acid long domain. NPR-C is expressed in kidneys, heart, brain, endothelium, adrenal glands, and smooth muscle cells similar or greater than NPR-A. However, unlike NPR-A, NPR-C has no guanyl cyclase activity. On the contrary, NPR-C activation leads to decreased cAMP levels. As a “clearance receptor,” NPR-C’s main function is to internalize and degrade ANP, as well as other natriuretic peptides, to modulate their activity in various tissues (see Table 12.1 ). This is supported by evidence in the NPR-C knockout mouse which has hypotension and reduced urinary concentration ability as a result of increased bioavailability of ANP.
Development
Natriuretic peptide receptors are present in the placenta as evidenced by expression of NPR-A in human uterine tissues, including decidua, chorion, and myometrium, and in the placenta. Notably, natriuretic peptide receptors in the human placenta are more significantly expressed on its fetal components than on the maternal microvillous membranes. NPR-C is downregulated in fetoplacental artery endothelial cells by the end of gestation, which is mediated, at least in part, by increased placental basic fibroblast growth factor secretion. This concerted downregulation by the end of gestation leads to increases in local ANP and cGMP concentrations, which may be critical for maintaining adequate blood flow in the placental and uterine tissues.
Renal response to ANP is still largely immature at birth. This is supported by evidence showing that natriuresis after ANP infusion is less efficient in young than adult sheep and rats. Two proposed mechanisms for this immature response are (1) lower ANP levels due to increased circulating ANP clearance and (2) attenuated renal response to ANP (see Table 12.2 ). In young and adult rats, ANP infusion leads to dose-dependent increments in urine sodium and cGMP excretion. However, in young rats, ANP levels after infusion are lower than in adults, implying that ANP gets cleared faster in immature rats. Enhanced NPR-C and neutral endopeptidase activity have been proposed as main contributors of ANP clearance in young rats, after experiments demonstrating that ANP levels in young rats doubled those of adult rats following NPR-C and neutral endopeptidases inhibition. On the other hand, attenuated renal response to ANP has been demonstrated by multiple experiments. In rats, expression of renal ANP receptors is low at birth, increasing thereafter to reach adult levels at the end of the fifth week of life. In addition, although intracellular cGMP production has been found to be higher in immature rats, active extracellular transport of cGMP is attenuated in immature rats. This correlates with a parallel maturation of the signal transduction pathways, as demonstrated by a progressive increase in ANP-induced renal cGMP synthesis during the first weeks of life.
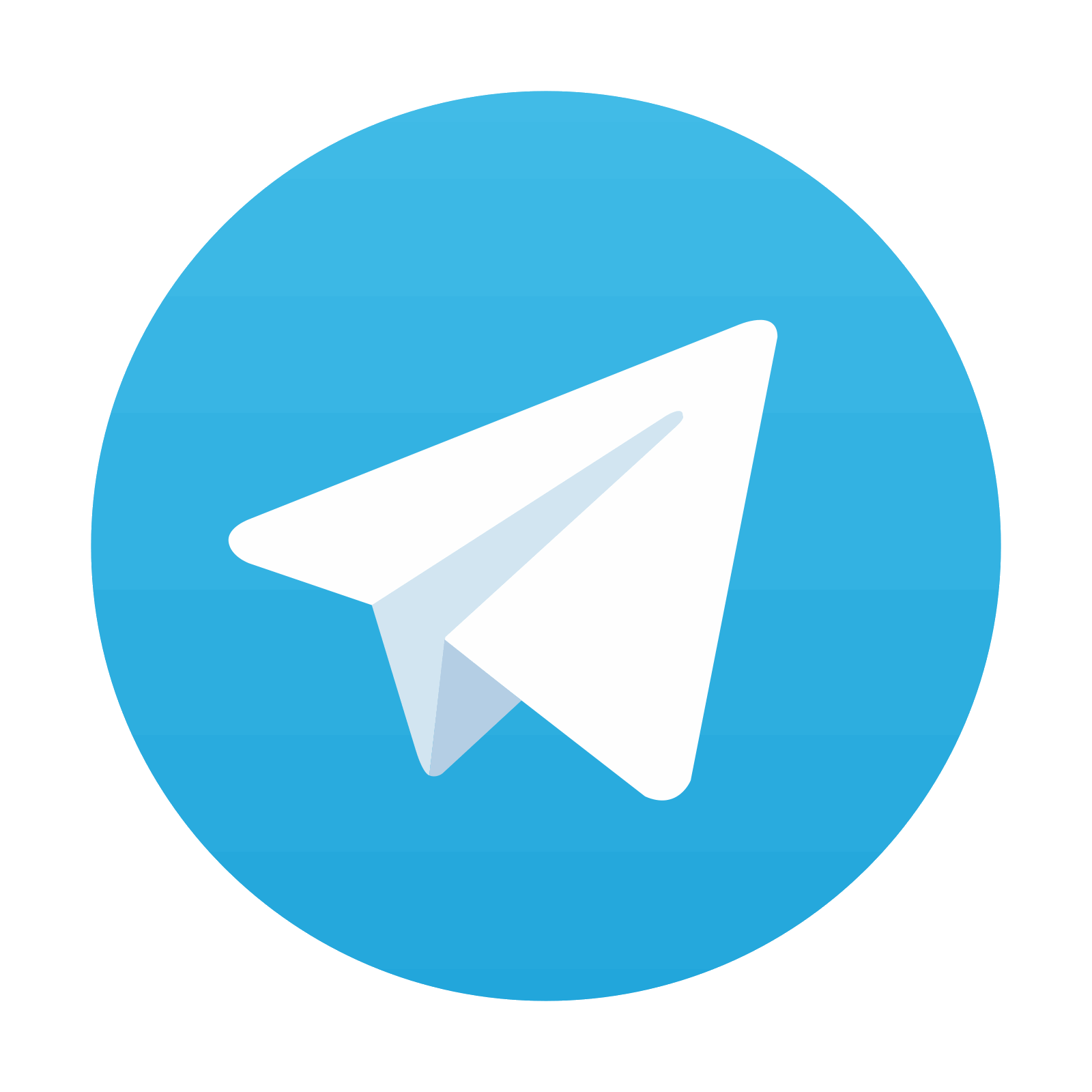
Stay updated, free articles. Join our Telegram channel
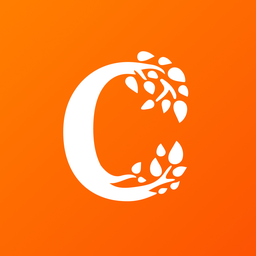
Full access? Get Clinical Tree
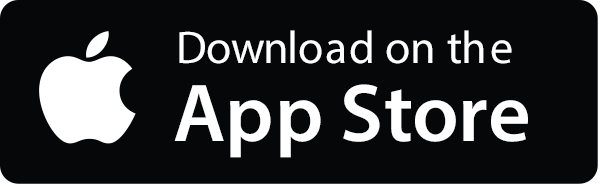
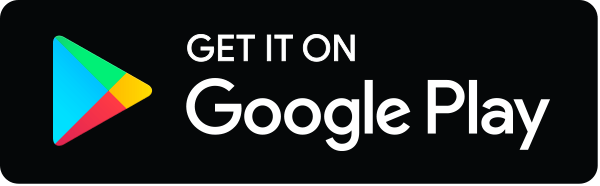