Abstract
Renal dysplasia is a leading cause of chronic kidney disease and end stage kidney disease in children. Understanding the molecular pathogenesis of renal maldevelopment began in the 1950s with classical studies by Clifford Grobstein, who isolated and characterized primordial tissues that interact to form the metanephric (adult) kidney. Since then, studies have shown that the following four embryonic tissues develop and send reciprocal signaling to form the kidney: the nephrogenic mesenchyme, the ureteric bud, the stromal mesenchyme, and the renal vasculature. Molecular biology and gene targeting techniques have also identified many genes and signaling pathways required for proper kidney formation in animal models. Recently epigenetic mechanisms have also been described as necessary for renal development in animal models. Studies in patients with syndromic and nonsyndromic renal dysplasia and other congenital renal malformations have identified many causative genetic defects that were previously identified in animal models. This chapter first reviews the molecular control of the embryonic tissues that form the kidney and then discusses the genes known to contribute to renal dysplasia in patients.
Keywords
renal dysplasia, kidney development, metanephric mesenchyme, ureteric bud, renal vasculature, genetics, epigenetics
- •
Human Renal Dysplasia
- •
Ureteric Bud
- •
Stromal Mesenchyme
- •
Vasculature
- •
Nephrogenic Mesenchyme
Introduction
Renal dysplasia is one of the leading causes of chronic kidney disease and end-stage kidney disease in children. Elucidating the molecular pathogenesis of renal maldevelopment began in the 1950s with classical studies by Clifford Grobstein, who isolated and characterized primordial tissues that interact to form the metanephric (adult) kidney. Since then, studies have shown that four embryonic tissues develop and send reciprocal signaling to form the kidney: the nephrogenic mesenchyme, ureteric bud, stromal mesenchyme, and renal vasculature (see ). Evolving molecular biology and gene targeting techniques have also identified many genes and signaling pathways required for proper kidney formation in animal models. Epigenetic mechanisms have also been described as necessary for renal development in animal models (see ). Studies in patients with syndromic and nonsyndromic renal dysplasia and other congenital renal malformations have identified many causative genetic defects that were previously identified in animal models (see ). This chapter will first review the molecular control of the embryonic tissues that form the kidney and then discuss the genes known to contribute to renal dysplasia in patients.
Nephrogenic Mesenchyme
The development of the human kidney begins in the fifth week of gestation, and the process of new nephron formation continues until approximately 32 to 36 weeks gestational age. The process begins with reciprocal inductive signals that cause outgrowth of the ureteric bud from the mesonephric duct and, in turn, the metanephric mesenchyme (from Osr1+ cells in the intermediate mesoderm) to condense around the ureteric bud to form nephrogenic mesenchyme (nephron progenitors). These progenitor cells self-renew throughout kidney development to generate an appropriate number of nephrons and have the capacity to form the multiple epithelial cell types of the nephron from the glomerular podocytes to the distal tubule. How these progenitor cells are specified and regulated in their self-renewal or differentiation is the subject of intense study because these processes are critical for determining nephron endowment, nephron pattern, and therefore long-term kidney health.
Following formation of the nephrogenic mesenchyme around ureteric bud tips, a subset of these cells receive spatiotemporal cues to begin differentiating into epithelialized renal vesicles ( Fig. 8.1 ). The renal vesicle then goes on to sequentially form the comma-shaped body and then the S -shaped body. The lower limb of the S -shaped body differentiates into glomerular podocytes, and both endothelial and mesangial cells migrate into the cleft of the lower limb of the S -shaped body to form the immature glomerulus. Concurrently, the middle and upper limbs of the S -shaped body elongate and differentiate into nephron tubules, including proximal tubules, loops of Henle, and distal convoluted tubules. The terminal ends of the distal convoluted tubules eventually connect to the ureteric epithelia. Nephrogenesis occurs in a radial fashion, such that the most mature nephrons exist in juxtamedullary regions and the most immature nephrons in the cortex (where nephron induction occurs), until the full complement of nephrons is reached.

Nephrons continue to mature structurally (nephron elongation and growth) and functionally (transport functions) prenatally and postnatally. For example, the loops of Henle begin in the renal cortex, elongate in utero through the corticomedullary boundary in term infants, and eventually reach the inner renal medulla. As a result, the urinary concentrating capacity of newborn infants is limited by a reduced medullary tonicity gradient, due to the relatively shorter loops of Henle. In the next subsections, we will review the molecular control of the process of nephron development.
Specification of Nephrogenic Mesenchyme
The differentiation of the metanephric mesenchyme to become nephrogenic mesenchyme is marked by the expression of several growth factors, cell adhesion molecules, and transcription factors. Thus the nephrogenic mesenchyme expresses a secreted peptide growth factor, glial-derived neurotrophic factor (Gdnf), which induces ureteric bud outgrowth and branching. The differentiation of the nephrogenic mesenchyme to the renal vesicle involves changes in cell motility, which is at least partly marked by changes in the expression of transmembrane molecules, such as cadherin-11 in the nephron progenitors to cadherin-6 in the renal vesicle. High-resolution expression studies have demonstrated that the nephrogenic mesenchyme is a spatially and temporally heterogeneous population. Thus the nephrogenic mesenchyme that exists nearer to the renal capsule around the ureteric tip represent a self-renewing population of nephron progenitors, whereas those located underneath the ureteric tip appear to be committed to differentiate into renal vesicles. Moreover, nephron progenitors that are present at midgestation versus late gestation in the mouse appear to have different capacities with respect to self-renewal or differentiation. The molecular mechanisms that regulate the cessation of nephrogenesis remain ill defined.
The use of tissue-specific gene knockouts in the mouse has revealed the functional importance of several transcription factors in nephron progenitors. Conditional deletion of Eyes absent homolog 1 (Eya1), Sine oculis homeobox homolog 1 (Six1), Paired box gene 2 (Pax2), Wilms tumor 1 (Wt1), Sal-like 1 (Sall1), Sine oculis homeobox homolog 2 (Six2), or Lim homeobox 1 (Lhx1) leads to severe bilateral kidney disease (absent or small and nonfunctional) from defects in nephron progenitor specification and/or differentiation. Pax2 -mutant mice generate a metanephric mesenchyme that is incompetent to differentiate into nephrons and fail to form the mesonephric duct, which is required for ureteric bud induction. In Lhx1 and Sall1 mutants, the metanephric mesenchyme does induce the ureteric bud, but it fails to elongate and branch, and the mesenchyme is also unable to differentiate. In Wt1 mutants a defective metanephric mesenchyme forms and rapidly undergoes apoptosis. Deletion of Six2 in mice results in the formation of ectopic nephron tubules and depletion of nephron progenitors.
The balance between self-renewal and differentiation of nephron progenitors is at least partly driven by differential wingless-related integration site (Wnt) signaling (low levels promote self-renewal and high levels stimulate differentiation of nephron progenitors). Secreted Wnt9b from the ureteric bud appears to be required for the differentiation of nephron progenitors; deletion of Wnt9b results in a failure of progenitors to undergo the mesenchymal to epithelial transition that is required to form the renal vesicle. In contrast, the fibroblast growth factor (Fgf) signaling pathway promotes the survival and proliferation of nephron progenitors. Nephrogenic zone cell culture studies revealed that addition of Fgf1, 2, 9, and 20 ligands promote nephron progenitor proliferation. This is corroborated by in vivo mouse studies that show that Fgf9 and Fgf20 are critical for maintaining nephron progenitor survival, proliferation, and competence to respond to inductive signals and that deletion of the Fgf receptors, Fgfr1 and Fgfr2, in the metanephric mesenchyme leads to small and poorly functioning kidneys. Other growth factors that have been implicated in progenitor survival include transforming growth factor-β2 (Tgf-β2), leukemia inhibitory factor (Lif), epidermal growth factor (Egf), Fgf2, and bone morphogenetic protein 7 (Bmp7).
Emerging evidence has implicated epigenetic mechanisms in the regulation of nephron progenitor specification, survival, and differentiation. For example, histone deacetylases (HDACs) function by removing acetyl groups from histones, which usually results in the stimulation of gene transcription. A study has revealed that class I HDACs are highly expressed in nephron progenitors and are required for normal expression of several key genes, including Osr1, Eya1, Pax2, Wt1, and Wnt9b. MicroRNAs (miRNAs) are small noncoding RNAs that bind to specific mRNA targets to block translation and promote mRNA degradation. Conditional deletion of a specific miRNA cluster, the miR-17–92 complex, in nephron progenitors leads to a decrease in progenitor proliferation, fewer nephrons, proteinuria, and chronic kidney disease.
Nephron Induction
Nephron progenitors undergo a mesenchymal to epithelial transition to form the renal vesicle, the most immature nephron. In vitro experiments have implicated several growth factors that stimulate nephron progenitors to undergo tubuloepithelial differentiation, including Fgf2, Lif, Tgf-β2, growth/differentiation factor-11 (Gdf-11), and Wnt1/4. Moreover, transgenic mouse models have identified critical endogenous pathways necessary for this mesenchymal to epithelial transition. For example, global deletion of Wnt4 (normally expressed in renal vesicles) results in an inability of the mutant nephron progenitors to epithelialize. Conditional deletion of Fgf8 in the metanephric mesenchyme (nephrogenic and stromal) leads to a block of nephrogenesis beyond the renal vesicle stage. Interestingly, global deletion of Fgfr-like 1, a membrane-bound Fgf receptor that lacks intracellular signaling, leads to a block in nephrogenesis similar to Fgf8 conditional mutants.
Nephron Segmentation
Shortly after renal vesicle formation, the developing nephron undergoes a series of morphologic changes to form the comma-shaped and then S -shaped body. These changes reflect the initial establishment of the proximal-distal axis required for nephron segmentation and patterning. Reciprocal repressive interactions between Wt1 and Pax2 appear vital to proximal-distal axis patterning. In the S -shaped body, Wt1 is localized to the lower limb and inhibits Pax2 expression, which together promotes a podocyte cell fate. In contrast, Pax2 is present in the upper limb and represses Wt1, stimulating these cells to become proximal and distal tubules.
Two other transcription factors important for proximal/distal axis patterning of the nephron are Lhx1 and brain-specific homeobox 1 (Brn1), both of which are expressed in the renal vesicle. Conditional deletion of Lhx1 leads to a loss of Brn1 expression. Conditional targeting of Brn1 results in failure of the loop of Henle to form and the distal convoluted tubules to terminally differentiate. These results suggest that Lhx1 acts upstream of Brn1, which is critical for distal nephron patterning. In contrast, Notch receptor signaling, mediated largely by the recombining binding protein suppressor of hairless (Rbpsuh), appears critical for proximal nephron patterning. Use of a Notch inhibitor in mouse metanephric kidney explants led to a loss of proximal cell fates, including glomeruli and proximal tubules. In vivo, conditional deletion of Notch2 or Rbpsuh leads to an absence of proximal tubules and glomerular podocytes. Finally, ectopic Notch expression in nephron progenitors results in premature differentiation into proximal nephron epithelia.
Podocyte Differentiation
Formation of the glomerulus begins at the S -shaped body stage, with the lower limb of the S -shape body committed to becoming podocytes. Initially, the immature podocytes are highly proliferative and have a columnar shape and a single-layer basement membrane. As the S -shaped body matures, the lower cleft transforms into a cup shape. This is accompanied by podocyte differentiation, forming foot processes and slit diaphragms (specialized intracellular junctions critical for proper glomerular filtration). This differentiation is associated with a transition in the glomerular basement membrane from laminin-1 to laminin-11, and from α-1 and α-2 type IV collagen chains to α-3, α-4, and α-5 type IV collagen chains. Several mouse models have shown that failure in these transitions leads to glomerular basement membrane defects.
Several transcription factors are known to be critical for podocyte differentiation, including Wt1, Lim homeobox transcription factor 1b (Lmx1b), and Mafb. Several studies using transgenic mouse models altering Wt1 expression have demonstrated its critical roles in mediating podocyte differentiation, in addition to its role in nephron progenitors. In addition, genetic deletion of Lmx1b or Mafb leads to podocyte differentiation defects after the capillary loop stage. Finally, three studies have demonstrated the importance of miRNAs in the maintenance of differentiated podocytes in the mouse by targeted ablation of Dicer (required for the production of mature miRNAs) in podocytes.
Ureteric Bud
As noted previously, the development of the mammalian kidney begins when signals from the metanephric mesenchyme elicit outgrowth of the ureteric bud from the caudal region of the nephric duct. The nephric duct itself is induced from the rostral region of the intermediate mesoderm and begins to elongate caudally. As the embryo develops, cells in the rostral portion of the nephric duct undergo apoptosis, whereas the caudal region of the nephric duct grows towards the cloaca. It is from this region of the nephric duct that the ureteric bud is induced to invade the adjacent metanephric mesenchyme. The ureteric bud elongates and branches, initially forming a T -shaped bifurcation and subsequently undergoing repetitive cycles of branching and elongation to form the collecting system of the kidney.
Development of the Nephric Duct
Genetically engineered mouse models have provided important insights into the mechanisms underlying nephric duct formation. For example, Pax2 knockout (Pax2 −/− ) mice lack kidneys and other structures derived from the intermediate mesoderm, including the ureters. Although the nephric duct starts to form in Pax2 −/− mice, it fails to extend and reach the cloaca, which results in its eventual degeneration. Because the ureteric bud cannot be induced from the nephric duct, no further kidney development occurs. Pax8, like Pax2, is expressed in precursors of the nephric duct, and these two transcription factors together specify the nephric duct lineage. S imultaneous deletion of Pax2 and Pax8 results in the failure of the intermediate mesoderm to commit to the nephric duct’s fate, and it undergoes massive apoptosis. Thus Pax2 −/− Pax8 −/− mice are incapable of forming a kidney.
Interestingly, Pax2 and Pax8 regulate the expression of a third gene that is indispensable for nephric duct development, the transcription factor Gata3. Gata3 −/− mice form multiple disorganized nephric ducts that extend toward the surface ectoderm (rather than a single nephric duct that elongates towards the cloaca). The absence of Gata3 results in increased proliferation of nephric duct cells that also lack expression of Ret, a receptor tyrosine kinase for Gdnf. Studies in Axolotl (Ambystoma mexicanum) demonstrated that Gdnf is chemoattractant for nephric duct cells that fail to migrate properly when Gdnf expression is disrupted. As expected, genetic deletion of Gdnf − , Ret, and retinaldehyde dehydrogenase 2 −/− ( Raldh2, an upstream regulator of Gata3 ) in mice results in nephric ducts that fail to fuse with the cloaca and ultimately urinary tract obstruction and hydronephrosis. The downstream molecular mechanisms activated by Ret/Gdnf signaling and its effects on kidney morphogenesis will be discussed later in this section.
The transcription factor genes hepatocyte nuclear factor-1β ( HNF1β; also known as vHNF1 ) and Lhx1 are other important regulators of nephric duct development. In HNF1β mutants, the nephric duct forms initially but subsequently degenerates. Because HNF1β is necessary for maintenance of Pax2 and Lhx1 expression, the phenotype is likely due to reduced levels of these genes in the nephric duct. Lhx1 is necessary for the differentiation of the intermediate mesoderm, as well as the formation and extension of the nephric duct towards the urogenital sinus. Therefore Lhx1 -deficient mice exhibit renal agenesis and lack reproductive tract structures.
In summary, the concerted action between Pax2, Pax8, HNF1β, and Lhx1 is necessary to specify the nephric duct lineage. Subsequently, downstream effectors such as Gata3, Gdnf, Ret, and Raldh2 act in parallel to regulate the elongation of the nephric duct and its correct insertion in the cloaca. Dysregulation of any step in this molecular network can lead to urinary tract defects, such as absent kidneys, urinary tract obstruction, and hydronephrosis.
Ureteric Bud Outgrowth
After specification and elongation, the nephric duct reaches the cloaca as a simple cuboidal epithelium. Shortly afterwards, the epithelium becomes pseudostratified and will soon give rise to the ureteric bud. At this point, the expression of Ret and its coreceptor Gfrα-1 becomes restricted to the caudal portion of the nephric duct, and Gdnf expression is restricted to the nephrogenic mesenchyme. The binding of Gdnf to Gfrα-1 (facilitated by heparan sulphate glycosaminoglycans) activates Ret, which in turn elicits a number of intracellular pathways that promote cell adhesion, proliferation, and migration. Among these are phosphatidylinositol 3-kinase (PI3K)/AKT, p38 mitogen-activated protein kinase (MAPK), RAS/extracellular signal-related kinase (ERK), and phospholipase Cγ (PLCγ)/Ca + (see ). A critical step in the Ret/Gdnf signaling pathway is the activation (via PI3K) of the ETS transcription factor genes, Etv4 and Etv5, which drive the expression of a number of transcripts required for ureteric bud outgrowth.
An elegant study by Chi et al. elucidated the cellular basis of Ret/Gdnf-mediated ureteric bud outgrowth. Using time-lapse imaging and chimeric embryos containing both Ret +/+ and Ret −/− cells, they demonstrated that Ret +/+ cells contribute preferentially to form the ureteric bud tip, whereas the Ret −/− cells form the ureteric trunk. This preferential positioning of Ret +/+ cells in the nascent ureteric bud tip facilitates their response to Gdnf signals to proliferate and branch and prevents an indiscriminate ureteric bud induction site. A similar scenario occurs in chimeric embryos containing Etv4- or Etv5- deficient cells, confirming a critical role for these ETS transcription factors in ureteric bud outgrowth and branching.
A complex signaling network is necessary for controlling the correct spatiotemporal execution of ureteric bud outgrowth and its precise positioning on the nephric duct ( Fig. 8.2 ). In this regard, Six1, Pax2, Sall1, Hox11, and Eya1 act in concert to positively modulate the expression of Gdnf in the nephrogenic mesenchyme (see ). Mutations in these genes result in failure of ureteric bud outgrowth, as would be expected. Conversely, Gdnf/Ret feedback inhibitors, such as Sprouty1 (Spry1), Foxc1, Bmp4, and Slit2 (and its receptor Robo2), restrict Gdnf/Ret-mediated ureteric bud outgrowth. If mutated, these aforementioned genes lead to the development of multiple ureters and multiplex kidneys due to the formation of ectopic ureteric buds.

Mutations that disrupt Gdnf/Ret signaling also cause renal developmental abnormalities. Ret −/− , GFRα-1 −/ , − and Gdnf −/− mice have absent kidneys due to failure of ureteric bud formation. The same kidney phenotypes occur in Etv4/5 −/− mice and mice lacking heparan sulfate 2-sulfotransferase (HS2ST), a key enzyme in heparan sulfate biosynthesis. Moreover, Ret mutations in the critical tyrosine residues Y 1062 (activates MAPK and PI3K pathways) or Y 1015 (activates PLCγ/Ca + ) result in absent kidneys or nonfunctional kidneys with megaureters, respectively.
Although Gdnf/Ret signaling is critical to the induction of ureteric bud outgrowth, other signaling pathways also have roles. This is supported by the observation that occasionally Ret −/− , Gfrα-1 −/− , and Gdnf −/− mice form some kidney tissue, albeit nonfunctional. Subsequent studies have demonstrated that Fgf signaling (likely Fgf10) can compensate for the combined loss of Ret, Gdnf, and Spry. This occurs because Fgfs can activate the same downstream signaling pathways stimulated by Gdnf/Ret (e.g., PI3K-AKT and RAS/MAPK), which are normally negatively regulated by Spry.
Ureteric Bud Branching Morphogenesis and Collecting Duct Elongation
Many of the regulators of bud outgrowth are also critical for ureteric bud branching morphogenesis, including Gdnf, Ret, and Etv4/5. Wnt signaling also plays a critical role in ureteric bud branching and collecting duct elongation. During embryonic kidney development, Wnt11 is present in the tips of the branching ureteric epithelium. Wnt11 functions as a positive feedback regulator of Gdnf/Ret signaling; Wnt11 deletion leads to a reduction in Gdnf expression and in the number of ureteric tips, resulting in development of small and poorly functioning kidneys. Wnt9b −/− mice exhibit a defective pattern of branching, in addition to nephron induction defects (outlined earlier). In addition, deletion of β-catenin in the ureteric lineage results in either absent or very small kidneys, due to dysregulation of hierarchical circuitry, involving Emx2, Lim1, Pax2, Ret, and Wnt11, all of which are required for appropriate ureteric branching.
Members of the Bmp family regulate ureteric bud branching and collecting duct morphogenesis, via activation of type I Bmp receptors (also known as Alk). Bmp4 is expressed in the mesenchymal cells surrounding the nephric duct and ureteric bud trunks. Genetic studies have demonstrated that Bmp4 antagonizes Gdnf/Ret signaling and ureteric outgrowth, preventing ectopic budding, and later regulates collecting duct elongation. Bmp4 −/− mice have a wide array of kidney and urinary tract defects, including dilated ureters, duplicated collecting system, and small or abnormally patterned kidneys. Mice with deletion of Alk3 have similar kidney and urinary tract defects.
The Fgf signaling pathway also regulates ureteric branching morphogenesis. Fgf7 and Fgf10, expressed in renal stroma, bind to and activate their cognate receptor, Fgfr2, expressed in the ureteric lineage. Global deletion of Fgf7, Fgf10, and the IIIb isoform (epithelial isoform) of Fgfr2 developing nephrons leads to small kidneys due to presumed ureteric branching defects. Conditional deletion of Fgfr2 in the ureteric lineage confirms that the small kidneys result from ureteric branching defects, due to changes in expression of other downstream genes critical for ureteric branching morphogenesis, such as Gdnf, Ret, Wnt11, and Etv4/5.
Stromal Mesenchyme
Origin and Development
Renal stromal progenitors likely originate from the Osr1+ cells of intermediate mesoderm that are also precursors of nephrogenic mesenchyme. Stromal progenitors are identified by their spindle-shaped appearance and the expression of the transcription factor Foxd1. At E10.5 in the mouse, stromal progenitors surround the nephrogenic mesenchyme, but as metanephric kidney development progresses, the stromal population expands and is distributed in the capsular, cortical, and medullary regions. Lineage tracing studies have shown that the renal stromal population gives rise to interstitial fibroblasts, pericytes, erythropoietin-producing cells, vascular smooth muscle cells, mesangial cells, and subsets of peritubular capillary endothelia of the kidney ( Fig. 8.3 ). Many of the signaling pathways that regulate the commitment of stromal progenitors towards these different lineages are yet to be defined.

Stromal Genetics (and Epigenetics) Implicated in Renal Development
Studies have shown that stromal progenitors are critical in patterning interstitial and vascular tissues and have nonautonomous effects on developing nephron progenitors and ureteric bud lineages. Mouse models that ablate expression of genes such as Foxd1, Hox10, and Ctnb1 in the stromal compartment reveal their critical roles in kidney development. Mice with global deletion of Foxd1 develop an abnormal renal capsule due to mispatterning of capsular stromal cells, resulting in fused kidneys that are unable to detach from the peritoneum and are trapped in the pelvic region. Mutant mice that lack the expression of Hox10 paralogs ( Hoxa10, Hoxc10, and Hoxd10 ) in renal stroma also develop severally malformed kidneys with impaired differentiation of capsular stromal cells that fail to integrate into the developing metanephric anlagen. Deletion of Ctnb1, encoding for β-catenin, an effector of canonical Wnt signaling, in Foxd1+ stroma progenitors impairs the development of renal medulla; conversely, expression of constitutively active β-catenin in the renal stromal compartment results in expansion of the stroma with disrupted nephrogenesis and ureteric branching morphogenesis.
Many studies have established that stroma directly signals to and regulates the size of the nephrogenic mesenchyme. Diphtheria toxin-mediated ablation of Foxd1+ stromal cells results in the increase in size of the nephron progenitor pool. Other studies have shown that the stromally expressed transcription factors Foxd1, Pbx1, Pod1, and Sall1 and atypical cadherin Fat4 regulate size of the cap (nephrogenic) mesenchyme. Mice with germline deletion of Foxd1, Pbx1, and Pod1 or with conditional deletion of Sall1 or Fat4 in the cortical stroma have an increase in the size of nephron progenitor pool. Interestingly, Fat4-mediated crosstalk with nephron progenitors is restricted to cortical stroma because deletion of Fat4 specifically in the medullary stroma does not affect the size of the nephron progenitor pool. The downstream mechanisms and targets of Fat4 in stromal cells remain controversial. In contrast, Decorin, a proteoglycan that is expressed in cortical stroma, appears to regulate the size of nephron progenitor pool. Decorin levels are increased in Foxd1 – and Sall1 -deficient stromal progenitors, which may lead to sequestration of Bmp4, which is required for the differentiation of nephron progenitors (thereby increasing the pool of self-renewing progenitors). Notably, concomitant deletion of Decorin partially rescues the nephron progenitor expansion defects in Foxd1 mutants. Studies have determined that miRNAs in stromal cells also regulate the size of nephron progenitor pool. Deletion of Dicer (required for the production of mature miRNAs) in Foxd1+ stromal cells leads to expansion of nephron progenitors and disrupts nephrogenesis (presumably due to upregulation of yet to be identified miRNA target genes).
As mentioned, studies have also identified signaling molecules emanating from the renal stroma that affect ureteric morphogenesis. Ablation of Foxd1+ stromal progenitors with diphtheria toxin not only results in nephron progenitor expansion but also in ureteric bud branching defects suggesting the role of stromal-ureteric bud crosstalk during kidney development. In vitro studies have shown that stromal cell production of retinoic acid, renin, and angiotensinogen triggers pathways that modulate the Gdnf/Ret/Wnt11 axis, which is critical for ureteric bud branching. Elegant in vivo studies by the Mendelsohn group showed how simultaneous knockout of two retinoic acid receptors, Rara and Rarb2, led to impaired Ret expression and subsequent ureteric branching defects, providing strong evidence that stromal signals crosstalk with the ureteric lineage.
Vasculature
A highly intricate network of blood vessels traverses through the kidney to ensure efficient filtration and tubular transport, as well as sufficient nutrient supply for metabolic demands of renal cells. Both angiogenesis (new vessels sprouting from existing vessels) and vasculogenesis (de novo vessel formation) contribute to the development of renal vasculature. Endothelial cells and mural cells (vascular smooth muscle cells and pericytes) constitute the main components of renal blood vessels. Renal blood vessels exhibit some features that are common to or in other cases distinct from the vascular beds of other organs; for example, fenestrated endothelia of glomerular capillaries are supported by mesangial cells that are specialized pericytes, whereas renal peritubular capillaries are surrounded by typical pericytes that enfold capillaries and venules throughout the body. Renal arteriolar endothelia are lined by layers of vascular smooth muscle cells and pericytes but also contact renin cells, specialized smooth muscle cells, at juxtaglomerular locations.
Origin and Development of Renal Endothelial Cells
Most of the renal endothelial cells are thought to be derived from hemangioblasts, common precursors for blood cells and endothelial cells, that express the transcription factor stem cell leukemia (Scl). Lineage tracing studies have shown that Scl+ cells are precursors to endothelial cells that line arteries, veins, arterioles, glomerular, and many peritubular capillaries of the kidney. A conditional knockout mouse model shows that G protein–coupled sphingosine-1-phosphate receptor signaling in Scl+ cells is essential for the proper development of the renal vasculature. Another group discovered that Foxd1+ stromal progenitors give rise to endothelial cells that line subsets of peritubular capillaries. One marker of Foxd1+ stromal-derived endothelial precursors is CD146, a coreceptor for vascular endothelial growth factor receptor 2.
Renal Vascular Mural Cell Development, Genetics, and Epigenetics
Mural cells include vascular smooth muscle cells, pericytes, and renin-producing cells that surround the renal endothelia. Lineage tracing studies have established that mural cells originate from the Foxd1+ stromal progenitor population and that much of the endothelial cell network develops within the stroma; moreover, diphtheria toxin–mediated deletion of Foxd1+ stromal progenitors during embryonic stages leads to severe vascular mispatterning. Other studies have shown that expression of transcription factor genes Foxd1 and Pbx1 in stromal progenitors is critical for the normal development of renal vasculature. Mutant mouse models lacking Foxd1 or Pbx1 expression show severe vascular patterning defects in which renal artery branching occurs in a random pattern in contrast to the stereotypical fashion observed in controls; this marked disruption in spatiotemporal vascular morphogenesis in Foxd1 and Pbx1 mutants leads to kidney failure resulting in perinatal lethality. Interestingly, Pbx1 mutants exhibit ectopic expression of platelet-derived growth factor receptor beta (Pdgfrβ), leading to premature differentiation of stromal progenitors and vascular defects, all of which can be at least partially rescued by a simultaneous one allele loss of Pdgfβ. The downstream targets of Foxd1 in stromal progenitors and mural cells are yet to be identified.
Foxd1+ stromal cells are also precursors of mesangial cells, a specialized pericyte population that attaches to glomerular endothelial cells that (together with podocytes) form an assembly critical for glomerular filtration. The Notch signaling pathway is essential for mesangial cell formation by regulating their specification, proliferation, and survival. Foxd1-cre mediated deletion of RBPJ, a well-established downstream target of Notch 1 and Notch 2 signaling, results in the failure of differentiation of stromal progenitors into mesangial cells. Another pathway essential for mesangial cell formation is Pdgfβ signaling. Glomerular endothelial cells secrete Pdgfβ that binds to its receptor, Pdgfrβ, expressed by mesangial cells; disruption of Pdgfβ/Pdgfrβ signaling leads to the failure of mesangial precursors to migrate to their proper position within glomeruli. As expected, the failure of mesangial cell formation in mutant mice with disrupted Notch and Pdgf signaling results in perinatal lethality due to kidney failure. Finally, conditional deletion of Dicer in the Foxd1+ stromal population leads to an absence of mesangial cells, showing that miRNAs are also critical for mesangial cell development from the stroma.
Renin-secreting cells are specialized vascular smooth muscle cells localized in the juxtaglomerular apparatus; these cells regulate blood pressure by secreting renin and also originate from Foxd1+ stromal progenitors. Although renin is also expressed in collecting ducts, conditional knockout studies have shown that deletion of the renin gene in collecting duct epithelium is dispensable for renal development; however, deletion of renin in Foxd1+ progenitors disrupts renal vasculature development resulting in hypertrophic arteries and arterioles with marked increased expression of smooth muscle actin.
Studies have also shown that tubular epithelial cells communicate with renal vascular cells and regulate their development. Wnt7b, a growth factor that is secreted by the developing ureteric bud, regulates proliferation of medullary peritubular capillary mural cells and ultimately vessel lumen diameter. Deletion of vascular endothelial growth factor a (Vegfa) in renal epithelial cells leads to reduction in the density of peritubular capillaries resulting in small kidneys.
Signaling pathways, such as angiopoietin, ephrin, and Tgf-β1, that are known to regulate vasculature development in other organ systems likely play crucial roles in the development of renal vasculature, and future studies will define their functions specifically in the endothelial and mural cells of the kidney. Identification of specific miRNAs involved in the regulation of renal vasculature is of significant interest based upon the aforementioned studies showing that stromal-specific Dicer deletion leads to progressive loss of mesangial cells. The identification and detailed understanding of the molecular networks that regulate the development of renal vasculature are crucial in defining the role of renal maldevelopment in pediatric and adult kidney disease and for the success of regenerative efforts to build a kidney.
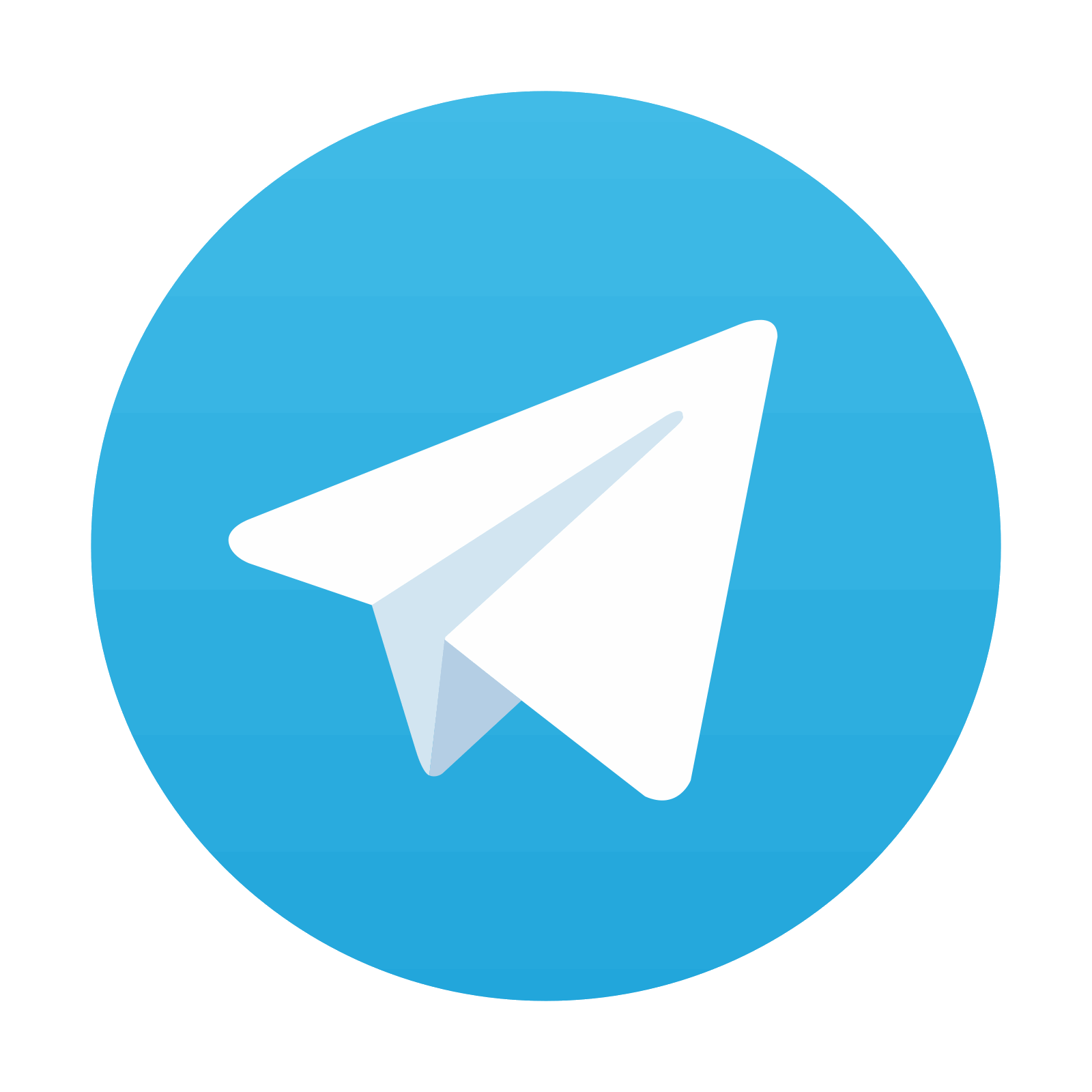
Stay updated, free articles. Join our Telegram channel
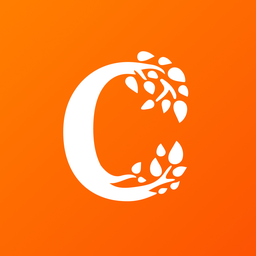
Full access? Get Clinical Tree
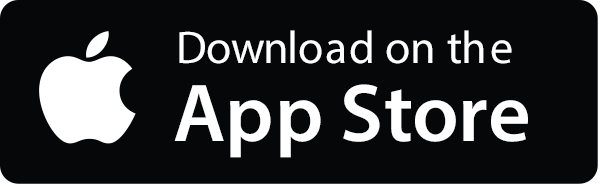
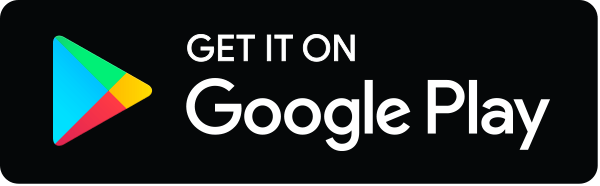