Anthony V. D’Amico, MD, PhD, Juanita M. Crook, MD, FRCPC, Clair J. Beard, MD, Theodore L. DeWeese, MD, Mark Hurwitz, MD, Irving D. Kaplan, MD Two major advances in the radiotherapeutic management of adenocarcinoma of the prostate have occurred since the early 1980s. The first advance was the generation of linear accelerators and conformal techniques capable of delivering high doses of radiation deep within the pelvis while simultaneously respecting the normal tissue tolerance of the anterior rectal wall, prostatic urethra, femoral heads, and bladder neck. The second advance occurred when image-guided techniques were introduced for use during the insertion of radioactive sources directly into the prostate gland. Eliminating the former freehand technique has vastly improved the physician’s ability to deliver high doses of radiation to the prostate gland while sparing interposed and juxtaposed normal structures. These two advances have increased the therapeutic ratio of radiation therapy (RT) in the management of prostate cancer. Specifically, decreased gastrointestinal toxicity has been documented (Dearnaley et al, 1999), and improved cancer control has been suggested (Pollack et al, 1999). The physical property that permits the photon radiation generated from a linear accelerator to penetrate deeply and spare normal tissue is the high energy of the beam. As the energy of the beam increases, the beam penetrates deeper before exerting its cytocidal effect. Whereas orthovoltage and 60Co units deposited their maximum dose within 1.25 cm below the skin surface, high-energy linear accelerators deliver the maximum dose of radiation at more than 15 cm below the skin surface. In addition, the use of multiple and conformal fields, as well as intensity-modulated and image-guided radiation therapy (Jani et al, 2003), has minimized the amount of rectum receiving the high-dose radiation volume, leading to lower rates of radiation-induced proctitis (Pollack et al, 1999). Recommendations for the treatment of clinically localized adenocarcinoma of the prostate should be made using the results of evidence-based medicine. The ideal end point on which to make therapeutic decisions is cause-specific survival. At present, follow-up is too short to make statistically meaningful statements regarding cause-specific survival for men diagnosed and treated for clinically localized disease in the prostate-specific antigen (PSA) era as a function of pretreatment prognostic factors and treatment modality. However, pretreatment prognostic factors have established roles in predicting recurrence (PSA, clinical) after external-beam RT (Pisansky et al, 1993; Zietman et al, 1994; Hanks et al, 1995; Lee et al, 1995; Zagars et al, 1995; Pisansky et al, 1997). These pretreatment factors include PSA level, biopsy Gleason grade, and 1992 American Joint Commission on Cancer Staging (AJCC) clinical stage. Combining these three factors has led to a definition of the three risk groups for patients managed with RT who have clinically localized disease. These three risk groups are as follows: Figure 104–1 shows 5-year actuarial data for 473 patients stratified using the clinical risk groups based on pretreatment PSA, biopsy Gleason grade, and 1992 AJCC clinical stage. Data (D’Amico et al, 2003) now exist to support the significant association of the pretreatment risk groups and prostate cancer–specific mortality (PCSM) following external-beam radiation as shown in Figure 104–2. Specifically, in a multi-institutional study of 2370 radiation-managed patients, the relative risk of PCSM for patients with high- or intermediate-risk disease compared with low-risk disease was 14.3 (95% confidence interval [CI]: 5.2 to 24.0; pCox < .0001) and 5.6 (95% CI: 2.0 to 9.3; pCox = .0012), respectively. For illustration, Figure 104–3 contains the relative contribution of PCSM and non-PCSM following treatment to all-cause mortality stratified by the patients’ age at the time of RT and the pretreatment risk group. These findings have been validated by others at Johns Hopkins (Hernandez et al, 2007) and the Mayo Clinic (Boorjian et al, 2008). Figure 104–2 Pretreatment risk groups and prostate cancer–specific mortality following external-beam radiation. The fraction of prostate biopsies found to contain prostate cancer is readily available information for all patients with PSA-detected or clinically palpable prostate cancer. The fraction of positive biopsies is obtained by dividing the number of positive cores by the number of cores sampled. Studies investigating the ability of the fraction of positive prostate biopsies × 100 (percentage of positive biopsies) to predict pathologic end points after RT suggest a role for this clinical factor in predicting tumor volume (Terris et al, 1995), extracapsular extension (Badalment et al, 1996; Borirakchanyavat et al, 1997), seminal vesicle invasion (D’Amico et al, 1996), lymph node involvement (Conrad et al, 1998), and percentage of Gleason grade 4 and 5 disease in the radical prostatectomy specimen (Epstein et al, 1994). The percentage of positive prostate biopsies has been shown to be an independent predictor of time to postoperative PSA failure after controlling for the established prognostic factors (D’Amico et al, 2000b). The percentage of positive prostate biopsies has also been shown to provide information in addition to the known prognostic factors for predicting PSA control after external-beam RT. Specifically, 473 men treated using three-dimensional conformal external-beam RT at the Joint Center for Radiation Therapy between 1989 and 1998 had detected PSA or clinically palpable prostate cancer. Figure 104–1 illustrates the ability of the previously described risk group system (D’Amico et al, 1998b) that was based on pretreatment PSA level, biopsy Gleason grade, and 1992 AJCC clinical stage to stratify patients according to PSA outcome. Specifically, 5 years after RT, 91%, 62%, and 43% of low-, intermediate-, and high-risk patients, respectively, had not experienced PSA failure as defined by the American Society for Therapeutic Radiology and Oncology (ASTRO) consensus panel (Consensus statement, 1997). Figure 104–4 illustrates the clinically relevant stratification provided by the percentage of positive biopsies in the previously defined intermediate-risk group on the basis of pretreatment PSA level, biopsy Gleason grade, and the 1992 AJCC clinical stage. Specifically, patients in the intermediate-risk subgroups who also had less than 34% of the positive biopsies improved their risk stratification for PSA outcome by one category to low risk. Conversely, patients with more than 50% of the positive biopsies performed less well than expected and were comparable with the high-risk patients. With longer follow-up, the impact of the percentage of prostate positive biopsies (PPBs) on prostate cancer–specific mortality in low-risk and favorable intermediate-risk patients has become available (D’Amico et al, 2004). Specifically, from a series of 421 patients with low-risk (PSA ≤ 10 ng/mL and biopsy Gleason score ≤ 6) or favorable intermediate-risk (PSA > 10 to 15 ng/mL or biopsy Gleason score 7 but not both factors) disease who underwent three-dimensional conformal radiation therapy (3DCRT) to a median dose of 70.4 Gy, a significant association between PCSM and the percentage of positive prostate biopsies at diagnosis was documented. In particular, the relative risk of PCSM following 3DCRT for patients with 50% or more as compared with less than 50% PPB was 10.4 (95% CI: 1.2 to 87; pCox = .03), 6.1 (95% CI: 1.3 to 28.6; pCox = .02), and 12.5 (95% CI: 1.5 to 107; pCox = .02) in men with a PSA of 10 or less and Gleason score 6 or less, PSA 10 or less and Gleason score 7 or less, and PSA 15 or less and Gleason score 6 or less, respectively. By 5 years after 3DCRT, up to 10% as compared with 2% or less (plog rank ≤ .01) of these patients experienced PCSM if they had 50% or more as compared with less than 50% PPB as shown in Figures 104-5, 104-6, and 104-7. For the purpose of illustration, Figure 104–8 contains the relative contributions of PCSM and non-PCSM following treatment to all-cause mortality stratified by the % PPB (<50% vs. 50% or more) and the PSA level and biopsy Gleason score at diagnosis. Studies (D’Amico et al, 2005; Palma et al, 2008) have documented that a pretreatment PSA velocity greater than 2 ng/mL/year is associated with an increased risk of biochemical recurrence, metastasis, and cancer-specific death following treatment with RT or RT and hormonal therapy. In particular, in men with otherwise low-risk disease undergoing external beam RT, a pretreatment PSA velocity greater than 2 ng/mL/year increased the risk of death from prostate cancer x-fold, which translated into 19% [95% CI: 2 to 39%] as compared with 0% of men dying of prostate cancer within 7 years following treatment with RT if the PSA velocity was more than as compared with 2 ng/mL/year or less, respectively. As a result such men planning to undergo RT should be considered for RT and hormonal therapy because of the known survival benefit when adding hormonal therapy to RT in men with locally advanced (Pilepich et al, 1995, 1998, 2001; Bolla et al, 2002) or localized high-risk disease (D’Amico et al, 2008). Key Points: Pretreatment Prognostic Factors Serum PSA has become widely accepted as a surrogate end point for monitoring the success of definitive treatment for localized prostate cancer. However, the definition of biochemical failure after RT remains controversial. Unlike the situation after radical prostatectomy, the patient successfully treated with radiotherapy still has a prostate gland and therefore is not expected to achieve an undetectable PSA reading. However, an “acceptable” PSA level after successful radiotherapy is markedly lower than the range of normal for an age-matched unirradiated population because of marked atrophy and a reduction in the size and number of nonmalignant acini (Grignon and Sakr, 1995). Ablation of normal prostatic epithelium appears to be dose dependent. In a randomized trial comparing 70 and 78 Gy, Pollack and colleagues (2002b) found that with 78 Gy, 80% of patients achieved a nadir of less than 0.5 ng/mL, compared with 67% who received 70 Gy (P = .02). Although higher nadirs are associated with decreased disease-free survival, it is probably not necessary to ablate all the normal epithelium in order to achieve cure. Five-year freedom from biochemical failure is in the range of 80% to 90% for nadirs less than 0.5 ng/mL (Zincke et al, 1994; Lee et al, 1996; Crook et al, 1998; Critz et al, 1999; Kestin et al, 1999; DeWitt et al, 2003) but drops to 29% to 60% for nadirs of 0.6 to 1.0. However, even for PSA nadirs above 1.0 ng/mL, up to one third of patients remain free of recurrence at 5 years (DeWitt et al, 2003). Nadir PSA is a significant predictor of outcome, but no absolute nadir threshold can or should be used to define cure (DeWitt et al, 2003; Ray et al, 2004). The 1996 ASTRO Consensus Conference (Consensus statement, 1997) proposed a standardized definition of biochemical failure after radiotherapy. Although there is no distinct PSA threshold that defines successful treatment, PSA stability following the nadir is important. The panel concluded that three consecutive increases in PSA is a reasonable definition of biochemical failure, recommending that the PSA determinations be 3 to 4 months apart in the first 2 years after radiotherapy and every 6 months thereafter. The requirement for three readings and the temporal spacing between the readings are necessary to avoid overcalling failure on the basis of temporary instability related to biologic noise or fluctuations in PSA of benign etiology (Crook et al, 1997a). It must be emphasized that establishing that a patient meets the criteria for biochemical failure is not justification for intervention. However, for the purposes of clinical trials and the reporting of data, a standard definition of failure is required. For these purposes, the date of failure should be backdated to midway between the PSA nadir and the date of the first increase. Although the ASTRO definition provided uniformity in reporting of results, it is not a perfect solution to the problem of defining biochemical failure after radiotherapy. Waiting for three rises delays the establishment of failure by 18 months or more (Cherullo et al, 2002). This has no consequences for the individual patient because no individual requires intervention before the third rise, which defines the change in status. Furthermore, biochemical failure is not equivalent to clinical failure and is not of itself an indication for additional treatment. However, when reporting results, waiting for three rises and then backdating failure to midway between the nadir and the first rise tends to flatten the tail of the survival curve, underestimating late failures (Coen et al, 2003). Long follow-up minimizes this effect and short follow-up overestimates treatment success (Horwitz et al, 2003) because outcome cannot be predicted when the median follow-up is shorter than the median time to failure. One means of overcoming this shortcoming in the ASTRO definition is to backdate the follow-up time for all patients with one or two PSA rises (Coen et al, 2003; Thames et al, 2003). These patients can be censored (as disease free) midway between their nadir and first rise at the same time as they would be censored as a failure should they go on to have a third rise. In this way, the actuarial calculation does not benefit from extended follow-up for these patients whose status is uncertain. An alternative definition of biochemical failure that is particularly useful when few PSA values are available, as occurs when a patient has been lost to follow-up for a period of time or when PSAs are checked only annually, is any PSA value that is more than 2 ng/mL above the nadir (Coen et al, 2003). Serum PSA declines slowly after completion of radiotherapy. The time to nadir has been shown to be inversely proportional to disease-free survival (Ray et al, 2004). The median time to nadir in patients who remain with no evidence of disease (NED) is 22 to 34 months (Hanlon et al, 2002; Pollack et al, 2002a). In a series of 615 men with a median follow-up of 64 months, Hanlon and colleagues (2002) reported that a lower nadir (P < .0001) and longer time to nadir are independent predictors of freedom from distant metastases (P = .0002). Lee and colleagues (1996) reported that 75% of men whose PSA reached a nadir in less than 12 months had distant metastases by 5 years as compared with 25% of those whose PSA took more than 12 months to reach a nadir (P < .001). Kestin and colleagues (1999) found that 92% of men whose PSA reached a nadir at 36 months or longer remained disease free as compared with 30% of those who reached a nadir in less than 12 months. The slow decline in PSA and the long time to PSA nadir are explained by the fact that, unlike other ablative treatment modalities such as surgery and cryotherapy, radiotherapy does not cause immediate cell death. The double-stranded DNA chromosomal breaks caused by radiotherapy are not immediately fatal but do not permit successful cell reproduction. Postmitotic cell death is not expressed until a cell tries to reproduce. Given the long doubling time of most prostate cancers, a fatally damaged cell may survive 18 to 24 months before unsuccessfully attempting cell division and dying. The level of PSA nadir achieved to some extent reflects the type of failure (Table 104–1). The median PSA nadir for NED patients in most series treated with external-beam radiotherapy is 0.4 to 0.5 ng/mL (Zietman et al, 1996; Critz et al, 1999; Crook et al, 2000), whereas for those exhibiting local failure it is often greater than 1.0 ng/mL and for distant failure greater than 2 ng/mL. A study by Hanlon and colleagues (2004) (n = 615, follow-up median 64 months) reported that freedom from distant metastases was 96% for a nadir less than 1 ng/mL, 89% for nadirs 1.1 to 2 ng/mL, and 61% for nadirs greater than 2 ng/mL. The postnadir doubling time of the PSA also correlates with the type of failure, with distant failures having shorter PSA doubling times of 3 to 6 months (Zagars et al, 1993; Hancock et al, 1995; Lee et al, 1997) and local failures having longer PSA doubling times of 11 to 13 months (Zagars et al, 1993; Hancock et al, 1995). D’Amico and coauthors (2003) reported that a PSA doubling time of less than 3 months is associated with prostate cancer mortality. Kuban and colleagues (2004) (n = 4839) found that PSA doubling time (<10 months vs. >10 months) and time to PSA failure (<2 years vs. >2 years) were the most significant factors in predicting distant metastasis-free survival after PSA failure. In any multivariate analysis of prognostic factors, if PSA nadir is included in the model with pretreatment PSA, Gleason score, and T stage, it becomes the strongest independent predictor of outcome (Ben-Josef et al, 1998; Crook et al, 1998; Preston et al, 1999). This is not surprising considering the close temporal relationship between the PSA nadir and the definition of biochemical recurrence. Although it is common practice to mix pretreatment prognostic factors in the same multivariate model with postradiotherapy PSA nadir, the latter should more correctly be considered a means of evaluating the response to radiotherapy. In the presence of residual benign epithelium or before the full effect of radiotherapy has been expressed, PSA may fluctuate or show several consecutive increases. A spike or bounce can be defined as a rise greater than 0.2 ng/mL followed by a durable decline (Merrick et al, 2003b). This phenomenon is especially common after brachytherapy, where it is reported to occur in 24% to 35% of men (Critz et al, 2000; Smathers et al, 2001; Merrick et al, 2002a, 2003b; Reed et al, 2003). Spikes have been reported to be more common in younger men and after implantation with 125I rather than 103Pd (33% vs. 17%) (Merrick et al, 2003a). Spikes can start any time from 9 to 30 months after brachytherapy (Reed et al, 2003) and may reach a maximum PSA value up to 10 ng/mL, although the majority shows a cumulative rise of not more than 2 to 3 ng/mL. Spikes may last as long as 12 to 18 months, and double peaks can be seen. Biopsies performed to investigate the rising PSA may show residual cancer with treatment effect (indeterminate) (Reed et al, 2003). Currently, there is no reliable way to discern whether a rising PSA in the first 3 years after brachytherapy represents treatment failure. Many meet the ASTRO definition of biochemical failure. Only patience and careful follow-up demonstrate the subsequent spontaneous PSA decline to low levels. In early reports, little was known about the rate of histologic clearance of irradiated tumor and failures were declared as early as 6 months after completion of radiotherapy (Scardino and Wheeler, 1985). It is now recognized that the time for histologic resolution of tumor parallels the time to serum PSA nadir and for the same reasons. Radiation causes postmitotic cell death, and fatally damaged cells may survive a limited number of cell divisions (Mostofi et al, 1992, 1993). Biopsies taken before histologic resolution is complete show a moderate to marked radiation effect (Crook et al, 1997b). Ultimate viability of these cells cannot be predicted. Crook and colleagues (1995) have determined that the optimal time to biopsy is 30 to 36 months after radiotherapy. Gleason scoring of irradiated prostate cancer should be performed only if the histologic evidence of radiation effect is absent or minimal. Gleason’s original work was based on surgically obtained material that had not been exposed to prior radiotherapy or hormonal therapy. It is based on gland architecture, which is known to be markedly altered by radiotherapy. Inappropriate application of the Gleason scoring system to disintegrating malignant glands showing a marked RT effect (Siders and Lee, 1992; Grignon and Sakr, 1995) results in a false-positive biopsy and perhaps unnecessary “salvage” therapy. Radiation atypia in benign glands can be severe enough to mimic malignancy (Bostwick et al, 1982; Grignon and Sakr, 1995; Cheng et al, 1999). Anticytokeratin monoclonal antibody for high-molecular-weight keratin labels the basal cell layer of benign glands and therefore helps to distinguish radiation atypia in benign glands from residual tumor where the basal cell layer would be absent (Brawer et al, 1989). Scoring systems for the degree of radiation effect have been proposed (Dhom and Degro, 1982; Bocking and Auffermann, 1987) on the basis of cytoplasmic and nuclear changes (Table 104–2) and can be helpful in interpreting the significance of residual tumor in the biopsy (Crook et al, 1997a). Their importance is to emphasize the need to differentiate between biopsies showing no or minimal radiation effect and those showing marked treatment effect (Zelefsky et al, 2004). Failure to do so dilutes the prognostic significance of biopsy status and may lead to inappropriate salvage therapy. Table 104–2 Grading Scheme for Cytoplasmic and Nuclear Radiation Effect RT, radiation therapy. Immunohistochemical stains for markers of cellular proliferation can also be useful in interpretation of the significance of residual tumor. The two nuclear proteins expressed in proliferating cells for which there is the most clinical experience are proliferative cell nuclear antigen or PCNA (Crook et al, 1994) and Ki-67 (Ljung et al, 1996). PCNA is expressed both at DNA replication for cell division and during cellular repair. It is not formalin stable and is unreliable if the duration of formalin fixation is not closely monitored (Wiatrowska et al, 1997). On the other hand, Ki-67 is expressed exclusively in proliferating cells, reaches a maximum in the mitotic phase, and is formalin stable. Positive immunohistochemical staining for either of these markers in residual tumor is strongly associated with subsequent failure (Crook et al, 2000). Although TRUS guidance is recommended for postradiotherapy prostate biopsies to ensure adequate and systematic sampling, TRUS alone is of limited diagnostic use because the increase in fibrosis alters the echogenic characteristics of the irradiated prostate (Crook et al, 1993; Svetec et al, 1998). Surviving malignant cells may be in the form of minimal scattered microscopic foci. False-negative rates approaching 20% have been reported (Crook et al, 2000). Key Points: Post-Treatment Prognostic Factors The question of whether the absence or presence of local control of a treated tumor is related to the subsequent development of metastatic disease was explored experimentally as far back as 1970. Mice with sarcomas of the extremity developed fewer pulmonary metastases if their affected limbs were amputated early in the course of disease (Suit et al, 1970). Given that RT is a local treatment modality, one of the goals of treatment became local control, with the expectation that only patients who achieved such control could be expected to be cured of their cancer. This principle was first examined in prostate cancer in the 1980s, when a relationship between treatment planning technique and cancer control was demonstrated in the Patterns of Care Studies (Leibel et al, 1984; Hanks et al, 1988). Patients’ treatment records were reviewed from 163 randomly selected radiation oncology departments located across the United States. Tumor control was better in patients who received higher doses of radiation to larger fields, at the expense of increased complications. Fully 40% of patients’ records indicated departure from current cancer guidelines for diagnostic evaluation and treatment. These studies sparked an intense interest in and ongoing effort to improve outcomes in men with prostate cancer by providing the best treatment planning and delivery systems possible (Leibel et al, 1994). Although external-beam RT has been used for decades to treat prostate cancer, it is still an evolving specialty. Until the 1970s, radiation oncologists had to treat cancers without precise knowledge of their location within the body. Knowledge of normal anatomy, routes of spread of a particular cancer, and limited information from diagnostic radiology were used for treatment planning (Asbell et al, 1980). Radiation oncologists became adept at designing their portals on the basis of skeletal anatomy. For the treatment of prostate cancer, the radiation portals were centered on the pubic symphysis and femoral heads. One group designed a rotating platform treatment; men with prostate cancer stood on a small mechanical platform that rotated 360 degrees while the radiation beam was aimed at the level of their pants pockets. This was considered advanced for its time. Later, oncologists learned to use additional tools for treatment planning. The location of the prostate was inferred indirectly by introducing a contrast-filled Foley catheter and rectal tube into the patient. The prostate was assumed to be located in the space between these two organs, as shown in Figure 104–9. As recently as the mid-1980s, this technique was considered state of the art. With the advent of computed tomography (CT) scanners, a direct method to localize the prostate within the bony pelvis became available, and optimization of radiation with both physical and biologic end points was finally possible (Mohan et al, 1992; Niemierko et al, 1992). Clinicians knew as early as 1980 that they were making significant errors in field placement without CT data, but limitations in computer hardware and software allowed no solution to the problem (Rosenman et al, 1991; Fraass, 1993). Fortunately, the dramatic and rapid improvement in computer availability, lowered costs, improved graphics, and rapid computational power has changed forever the field of radiation oncology. Men with prostate cancer are the direct beneficiaries of the new technologies, and prostate cancer is one disease for which the treatment of today bears little resemblance to that used as recently as the late 1980s (Fraass, 1995). The 1990s began the era of three-dimensional tumor visualization and treatment planning. For the first time, the internal organs—the prostate and surrounding structures—could be identified with increased precision, and the beam’s eye view, a technique allowing visualization of the regional anatomy from the perspective of the beam apertures, enabled the design of plans that could more accurately treat the prostate target while minimizing dose to the surrounding normal tissues. The result is loosely described as conformal radiation therapy (CRT) because the radiation beams conform to the shape of the treatment target (Fraass, 1995). The ability to deliver CRT requires special hardware, software, and training. In the United States, there are approximately 1500 radiation facilities, only 20% of which are associated with a university or residency training program (D’Amico and Hanks, 1999). Today’s residency programs all educate their radiation oncology residents in the art of CRT, and each year more practices offer this treatment as a standard therapy. One of the goals of conformal prostate radiation is to lower the dose to the surrounding normal tissues, such as the rectum and bladder, while simultaneously increasing the dose delivered to the prostate itself (Burman et al, 1991; Niemierko et al, 1992). All radiation oncologists agree that CT data allow more accurate placement of the radiation fields. This was first demonstrated by Pilepich and colleagues (1982), who found a 53% error rate in field placement and portal size in patients simulated without CT data. In theory, better pretreatment visualization and localization of the prostate eliminate the need to enlarge the radiation portal to account for anatomic and geometric uncertainties. It follows that smaller radiation portals allow less irradiation of nontarget structures such as the bladder or rectum. However, some data suggest that the geometric relationships between the prostate and the contiguous normal tissues vary within the same patient owing to physiologic motion of the pelvis in a random fashion that can be difficult to categorize (Ten Haken et al, 1991; Beard et al, 1996; Zelefsky et al, 1999b). The proximity of the prostate to the radiation-sensitive bladder, rectum, neurovascular bundles, and erectile tissue of the penis has always been a concern; patients who undergo external-beam radiation are at risk for gastrointestinal, genitourinary, and sexual side effects. The percentage of patients who experience side effects and the severity of the side effects differ somewhat from series to series, depending on the morbidity scale used and also on whether the assessment is physician based or patient based (Table 104–3) (Pilepich et al, 1987; Lawton et al, 1991; Austin-Seymour et al, 1994; Beard et al, 1997, 1998). In general, prospective patient-based assessments yield higher percentages of patients experiencing side effects, irrespective of the type of treatment studied (Beard et al, 1997; Talcott et al, 1998). If there is still some debate about whether complication rates have decreased as a result of conformal treatment, it is probably because clinicians increased their doses as treatment portals became smaller. Also, although normal tissue complication rates have been established to be related to radiation dose and volume, they are also influenced by other dosimetric and clinical factors such as coexisting disease in individual patients that cannot be fully controlled or described in clinical studies (Pollack et al, 2003). Several authors (Sandler et al, 1992, 1995; Leibel et al, 1994; Schultheiss et al, 1995, 1997) have compared the complication rates in their own prostate cancer patients receiving conventional versus conformal radiation. Most authors use the Radiation Therapy Oncology Group (RTOG) late-radiation morbidity scales, as shown in Table 104–4. Table 104–4 RTOG Late Gastrointestinal and Genitourinary Morbidity Scales RTOG, Radiation Therapy Oncology Group. A prospective randomized trial was performed in the University Hospital of Rotterdam and the Daniel den Hoed Cancer Center of the Netherlands comparing the effect of 66 Gy delivered by either conventional therapy or CRT in a group of 266 patients (Koper et al, 1999). All patients were evaluated for gastrointestinal and genitourinary outcomes. A statistically significant reduction was seen in the proportion of patients experiencing grade 2 rectal toxicity in the conformal arm of the study (19% vs. 32%) (P = .02). Most of the benefit with conformal RT was due to a low incidence of anal side effects (8% compared with 16% experienced by the control group). Interestingly, no difference was seen in the percentages of patients who experienced bladder symptoms, which were minimal in both arms of the trial. A second prospective randomized trial comparing conformal and conventional radiation was performed at the Royal Marsden Hospital (Dearnaley et al, 1999). In that trial, 225 men were randomly assigned to receive 64 Gy of one or the other form of radiation. Only 5% of the conformal group developed grade 2 proctitis and bleeding, compared with 15% of the conventional group (P = .01). As in the Dutch study, no difference was seen between the groups in the development of grade 1 or 2 bladder symptoms. Sexual function after conformal RT has been evaluated by several groups. At the Joint Center for Radiation Therapy and the Dana-Farber Cancer Institute (DFCI), patients participating in a prospective multi-institutional outcomes trial were divided into three groups on the basis of their RT treatment technique (Beard et al, 1997). As shown in Table 104–5, fewer patients reported sexual side effects after treatment with conformal radiation than with small-field or whole-pelvis treatment. None of the values were statistically significant, but this may have been due in part to the small sample size studied. Table 104–5 Prospective Multi-Institutional Outcomes Data on Sexual Symptoms over Time in Patients Treated with Conventional Whole-Pelvis Irradiation, Conventional Small-Field Irradiation, and Conformal Irradiation without Dose Escalation New data exist on the relationship between dose and morbidity. For prostate cancer, most of the progress has been made in the area of rectal complications. Predictors of bladder complications have yet to be identified, perhaps because they manifest many years after treatment (Gardner et al, 2002). Most “late” rectal complications, however, are seen within 2 years and almost always within 4 years of treatment (Pollack et al, 2002b) and are more easily evaluated. In the M.D. Anderson Cancer Center trial, the 5-year freedom from a complication above grade 2 was 26% in the 78-Gy arm and 12% for those in the 70-Gy arm, nicely demonstrating the relationship that can exist between dose and complications. When further analysis was performed to identify factors other than total dose that might be used to predict for rectal toxicity, it was shown that the risk of complications increased from 25% to 46% when the rectal volume exposed to 70 Gy increased from less than 25% to greater than 25% (Pollack et al, 2002a). A more detailed analysis followed and showed that it was the proportion of the rectum receiving greater than 70 Gy that was more significant than the absolute rectal volume (Huang et al, 2002b), but the basic premise remained the same. Unlike that after radical prostatectomy, sexual dysfunction after RT increases slowly with time (Talcott et al, 1998). Zelefsky and colleagues (1999a) from Memorial Sloan-Kettering Cancer Center reported that 39% of 542 previously potent patients given 64.8 to 81 Gy of conformal radiation became impotent within 5 years of treatment. A retrospective evaluation of 287 men from the University of Chicago yielded similar results, with 53% of previously potent men reporting impotence by 60 months after conformal therapy (Mantz et al, 1999). As with other studies of sexual function after radiation, the men most likely to remain potent after treatment were those with good pretreatment sexual function and little or no comorbid illness and those who did not receive androgen therapy. Key Point: Treatment—Cancer Control and Quality of Life Zelefsky and colleagues (1998) reported their results for the first 743 men with prostate cancer treated with prospective dose-escalation conformal radiation. Patients were divided into favorable (stage T1 or T2, PSA < 10 ng/mL, Gleason grade < 6), intermediate (having one unfavorable feature), and unfavorable (having two unfavorable features) groups. For these three groups, the 5-year PSA freedom-from-relapse rates were 85%, 65%, and 35%, respectively. Almost identical data were obtained by Fukunaga-Johnson and colleagues (1997) at the University of Michigan. In that series, 707 patients received conformal radiation at doses of up to 80 Gy, with most patients receiving more than 69 Gy. Patients with favorable features (defined as stage T1 or T2, Gleason grade 7, and PSA < 10 ng/mL) enjoyed a 75% 5-year freedom from PSA relapse, compared with a 37% rate in those with unfavorable features (T3 or T4, Gleason grade > 7, or PSA > 10 ng/mL). Researchers at the Fox Chase Cancer Center in Philadelphia modeled the effect of dose levels less than 74 Gy against those greater than 74 Gy using pairs of patients matched by stage, pretreatment PSA level, and Gleason grade (Hanks et al, 1999). When PSA was discounted, delivered dose greater than 74 Gy correlated significantly with freedom from local disease progression and freedom from distant metastases and increased cause-specific and overall survival. The results were still significant for freedom from disease progression and freedom from distant metastases when PSA was included as an independent variable. Six hundred eighteen patients who received conformal radiation were divided into groups on the basis of their pretreatment tumor characteristics (Gleason grade, clinical stage, and PSA level) and their total radiation dose (76 Gy vs. more than 76 Gy). Six subgroups were created. Patients with favorable tumors (Gleason grade 6, stage T1 or T2a) who also had a PSA less than 10 ng/mL derived no benefit from dose escalation because all patients in this group did well. Patients with unfavorable tumors (Gleason grade 7 to 10, stage T2b or T3) who had a PSA greater than 20 ng/mL also derived no benefit from dose escalation. In this cohort, both arms fared poorly, with freedom-from-relapse rates that were less than 30%. All other patients demonstrated statistically significant improved freedom-from-relapse rates with high-dose conformal treatment, as shown in Figure 104–10. (Modified from Hanks G, Hanlon A, Pinover W, et al. Survival advantage for prostate cancer patients treated with high-dose three-dimensional conformal radiotherapy. Cancer J Sci Am 1999;5:152–8.) It has long been understood that increased doses of external RT are associated with better cancer control rates for patients with prostate cancer. For many years, an isocenter dose of 70 Gy was considered the maximum dose that could be safely delivered. With the advent of CRT, several groups looked at higher doses in the setting of a randomized trial. At Massachusetts General Hospital, a trial randomizing between 67.2 Gy (conventional arm) and 75.6 Gy (proton boost arm) was undertaken in patients with locally advanced disease. The study showed a significant increase in local control with the higher dose, with 8-year rates of 19% and 84% (P = .0014) for patients in the high- and low-dose arms, respectively (Shipley et al, 1995). Disease-free survival was also better for a subset of patients with Gleason grade 8 to 10 cancer, but no differences in overall or cancer-specific survival were noted. A number of CRT and intensity-modulated radiotherapy (IMRT) dose escalation trials are under way or have completed accrual (Pollack), but only the M.D. Anderson Cancer Center trial has reached maturity and been published. In this trial, 301 patients were randomized; 150 received the standard CRT dose of 70 Gy at isocenter, and the other 150 received the higher dose of 78 Gy at isocenter (Pollack et al, 2002b). The 6-year rates of freedom from failure were significantly higher for those randomly assigned to the 78-Gy arm (70% vs. 64%, P = .03). The greatest benefit was observed for patients with a pretreatment PSA greater than 10 ng/mL. These intermediate- to high-risk patients had 6-year freedom-from-failure rates of 62% and 43% when treated with 78 and 70 Gy, respectively (P = .12). There was also a borderline reduction in distant metastases for patients in this group who received the higher dose (2% vs. 12%, P = .056). Although CRT was a major technological advance, it represented advancement of treatment planning processes that had been in place since the 1980s. Real progress in the delivery of radiation came with the advent of IMRT. IMRT is an exciting form of conformal RT that uses extremely advanced software, specialized personnel, and hardware adaptations to linear accelerators (Burman et al, 1997). At present, its availability is limited to a small number of radiation centers because of its complexity, but in time it may replace CRT in popularity and availability. The goal of IMRT treatment planning and delivery is to maximize treatment to the target (e.g., the prostate) while minimizing treatment to the surrounding tissues to a degree that is not possible with conformal therapy (Oh et al, 1999). The term IMRT is now applied to a family of methodologies that use different methods of treatment planning and delivery in order to achieve a precise, nonuniform beam fluence (Jani et al, 2003). IMRT uses a new planning approach referred to as inverse treatment planning. This approach gives equal attention to the areas where radiation dose is to be minimized and the areas that are to receive high-dose treatment. Inverse planning uses a mathematic approach to convert a desired dose distribution to the target volume and normal organs into a clinically applicable treatment plan. The planning computer, using a mathematic optimization technique known as simulated annealing, determines the optimized beam arrangement. The second component of IMRT is computer-controlled modulation of the intensity of the radiation beam during treatment. The outcome is a set of radiation beams with changing intensities across the field, as shown in Figure 104–11. With this technology, the prostate can receive a daily dose of 180 cGy, whereas the majority of the adjacent bladder receives less than half of this dose. An example of an intensity-modulated beam is shown in Figure 104–12. The tight distribution of radiation around the prostate with avoidance of most of the rectum and bladder is seen on inspection of the figure. IMRT for prostate cancer generally uses five or seven radiation ports or fields per day, as shown in Figure 104–13. Prostate cancer is currently the single most common tumor site treated with IMRT worldwide (Guerrero Urbano et al, 2004
Historical Perspective
Localized Disease
Pretreatment Prognostic Factors
Pretreatment Risk Groups and Prostate Cancer–Specific Mortality
Percentage of Positive Prostate Biopsies and Intermediate-Risk Patients
Percentage of Positive Prostate Biopsies and Prostate Cancer–Specific Mortality in Low-Risk and Favorable Intermediate-Risk Patients
Pretreatment PSA Velocity and the Risk of Prostate Cancer–Specific Mortality
Post-Treatment Prognostic Factors
Localized Disease—Evaluating the Response to Radiation
PSA Follow-up—Definition of Failure
Time to Nadir
Significance of Nadir Value and Doubling Time
Benign Bounce Phenomenon
Postradiation Therapy Biopsy
Timing
Interpretation
Markers of Cellular Proliferation
Sampling Error
Treatment: Cancer Control and Quality of Life
GRADE
GENITOURINARY
GASTROINTESTINAL
2
Moderate frequency; generalized telangiectasia; intermittent macroscopic hematuria
More than two antidiarrheals per week; regular non-narcotic for pain; occasional blood transfusion; occasional steroids; occasional dilation; intermittent use of pads
3
Severe frequency and dysuria; severe generalized telangiectasia; frequent hematuria; reduction in bladder capacity (<150 mL)
More than two antidiarrheals per day; regular narcotic for pain; frequent blood transfusions; steroid enemas; hyperbaric oxygen for ulceration; regular dilation; daily use of pads
4
Necrosis; contracted bladder (<100 mL); severe hemorrhagic cystitis
Perforation; life-threatening bleeding; surgical repair
5
Fatal toxicity
Fatal toxicity
Tumor Control after Conformal Radiation Therapy
Intensity Modulation
Stay updated, free articles. Join our Telegram channel
Full access? Get Clinical Tree
Radiation Therapy for Prostate Cancer
• Low risk: more than 85% 5-year PSA failure-free survival, 1992 AJCC clinical stage T1c-T2a and PSA level of 10 ng/mL or lower, and biopsy Gleason grade of 6 or lower
• Prostate cancer–specific mortality can be estimated on the basis of the pretreatment PSA level, biopsy Gleason score, and clinical T category.
(The scores for cytoplasmic and nuclear changes are added together. A score of 5-6 represents marked treatment effect, 3-4 is moderate, and 0-2 minimal.)
• The level of PSA nadir achieved following radiation therapy reflects the pattern of failure. Specifically, a nadir greater than 2 ng/mL is associated with distant failure.
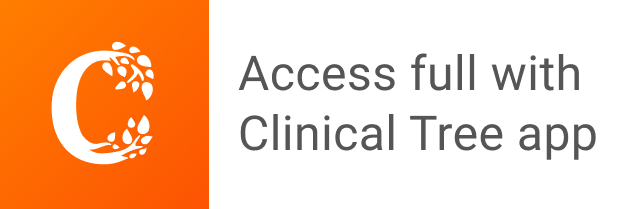