Robert M. Weiss, MD
The function of the ureter is to transport urine from the kidney to the bladder. Under normal conditions, ureteral peristalsis originates with electric activity at pacemaker sites located in the proximal portion of the urinary collecting system (Bozler, 1942; Weiss et al, 1967; Constantinou, 1974; Gosling and Dixon, 1974; Tsuchida and Yamaguchi, 1977; Zhang and Lang, 1994; Lammers et al, 1996). The electric activity is then propagated distally and gives rise to the mechanical event of peristalsis, ureteral contraction, which propels the bolus of urine distally. Efficient propulsion of the urinary bolus depends on the ureter’s ability to completely coapt its walls (Woodburne and Lapides, 1972). Urine passes into the bladder through the ureterovesical junction (UVJ), which, under normal conditions, permits urine to pass from the ureter into the bladder but not from the bladder into the ureter.
Cellular Anatomy
Around the periphery of the cell are numerous cavitary structures, some of which open to the outside of the cell and are referred to as caveolae. These caveolae contain a cytoskeletal protein, caveolin, and a variety of signal transduction molecules and receptors for growth factors and cytokines (William and Lisanti, 2004). A double-layer cell membrane surrounds the cell. The inner plasma membrane surrounds the entire cell, but the outer basement membrane is absent at areas of close cell-to-cell contact, referred to as intermediate junctions.
Development of the Ureter
The ureter, a 25- to 30-cm tube extending from the renal pelvis to the bladder, arises as an outpouching from the mesonephric duct. This begins on embryonic day (E) 10.5 in mice and E28 in humans. Signals from the metanephric mesenchyme, stroma, and angioblasts induce the ureteral bud to arise from the mesonephric duct, invade the mesenchyme, and undergo branching. Formation of the ureteric bud and its subsequent branching is induced by glial cell line–derived neurotrophic factor (GDNF), derived from adjacent metanephrogenic mesenchyme (Pepicelli et al, 1997; Sainio et al, 1997; Shakya et al, 2005). GDNF signals through the c–RET receptor tyrosine kinase (Vega et al, 1996), which results in increased phosphatidylinositol-3-kinase (P13-kinase) activity and AKT/PKB phosphorylation (Tang et al, 2002). Expression of GDNF or c-RET is activated by a transcription factor present in the metanephric mesenchyme, paired box 2 (from PAX-2 gene) (Brophy et al, 2001; Clarke et al, 2006). A number of other growth factors, including transforming growth factor–β (TGF-β); hepatocyte growth factor (HGF); fibroblast growth factors (FGF 1, 2, 7, and 10); transcription factors, from Foxd1, Wnt11, and Spry1 genes; and matrix molecules, such as heparin sulphate proteoglycans, laminins, integrins, and matrix metalloproteinases (MMP9) are involved in stimulation or inhibition of growth and branching of the ureteral bud (Davies et al, 1995; Mendelsohn et al, 1999; Qiao et al, 1999; Pohl et al, 2000; Davies, 2001; Qiao et al, 2001; Takemura et al, 2002; Majumdar et al, 2003; Sakurai, 2003; Bush et al, 2004; Chen et al, 2004; Basson et al, 2006). Activin A, a member of the TGF-β family, is an endogenous inhibitor of ureteric bud outgrowth from the wolffian duct (Maeshima et al, 2006). Activin A inhibits GDNF-induced ureteral bud formation, and this is accompanied by inhibition of cell proliferation, reduced expression of PAX-2, and decreased phosphorylation of PI3-kinase and MAP kinase in the wolffian duct. The tip of each ureteral bud is capable of inducing adjacent metanephric mesenchyme to undergo a mesenchymal-to-epithelial transition (MET), with the formation of the nephron (Ekblom, 1989; Shah et al, 2004).
Programmed cell death, or apoptosis, is involved in branching of the ureteric bud and subsequent nephrogenesis. Inhibitors of caspases, which are involved in the apoptotic signaling pathway, inhibit ureteral bud branching (Araki et al, 1999). During development, the ureteral lumen is obliterated, and then it recanalizes (Russo-Gil et al, 1975; Alcaraz et al, 1991). It appears that angiotensin, acting through the angiotensin II receptor, is involved in the recanalization process (Yerkes et al, 1998) and in the inhibition of aberrant ureteral budding (Oshima et al, 2001). Knockout mice for the ATR2 gene have congenital anomalies of the kidney and urinary tract, including duplicated collecting systems with a hydronephrotic upper pole moiety, multicystic dysplastic kidneys, megaureters, and ureteropelvic junction (UPJ) obstructions. Mutant mice lacking angiotensin II type I receptors fail to develop a renal pelvis and lack ureteral peristaltic activity (Miyazaki et al, 1998). Angiotensin II, acting through angiotensin II type I receptors, also is involved in ureteric bud cell branching, a process that depends on phosphorylation of the epidermal growth factor (EGF) receptor (Iosipiv and Schroeder, 2003; Yosypiv et al, 2006).
Calcineurin, a Ca2+–dependent serine/threonine phosphatase also appears to be an essential signaling molecule in urinary tract development. Mutant mice in which calcineurin function is removed are noted to have reduced proliferation of smooth muscle and mesenchymal cells in the developing urinary tract, with abnormal development of the renal pelvis and ureter with resultant defective pyeloureteral peristalsis (Chang et al, 2004).
Electric Activity
The electric properties of all excitable tissues depend on the distribution of ions on both the inside and the outside of the cell membrane and on the relative permeability of the cell membrane to these ions (Hodgkin, 1958). The ionic basis for electric activity in ureteral smooth muscle has not been fully described; however, many of its properties resemble those in other excitable tissues.
Resting Potential
When a ureteral muscle cell is in a nonexcited or resting state, the electric potential difference across the cell membrane, transmembrane potential, is referred to as the resting membrane potential (RMP). The RMP is determined primarily by the distribution of potassium ions (K+) across the cell membrane and by the permeability of the membrane to K+ (Hendrickx et al, 1975). In the resting state, the K+ concentration on the inside of the cell is greater than that on the outside of the cell, that is, [K+]i > [K+]o, and the membrane is preferentially permeable to K+. Because of the tendency for the positively charged K+ ions to diffuse from the inside of the cell, where they are more concentrated, to the outside of the cell, where they are less concentrated, an electric gradient is created, with the inside of the cell membrane being more negative than the outside (Fig. 59–1A). The electric gradient that is formed tends to oppose the further movement of K+ outward across the cell membrane along its concentration gradient, and an equilibrium is reached.
where Ek is the potential difference attributable to the concentration difference of K+ across the cell membrane, R is the molar gas constant, T is the absolute temperature, n is the number of moles of K+, and F is the faraday constant (Nernst, 1908). However, in the ureter and in other smooth muscles, the RMP is considerably less than the K+ equilibrium potential, with values of −33 to −70 mV, the inside of the cell being negative relative to the outside (Kuriyama et al, 1967). Studies from single isolated ureteral cells show spontaneous transient hyperpolarizations, with the RMP transiently becoming more negative (Imaizumi et al, 1989). This phenomenon appears to be due to spontaneous release of calcium ions (Ca2+) from the sarcoplasmic reticulum, with activation of tetraethylammonium (TEA) and charbydotoxin-sensitive Ca2+-dependent K+ channels (IK(Ca)). Although the low resting potential of ureteral cells may be explained in part by a relatively small resting K+ conductance (Imaizumi et al, 1989), it also may be due to the contribution of other ions.
One such ion that could account for the relatively low RMP of the ureter and other smooth muscles is the sodium ion (Na+) (Kuriyama, 1963). In the resting state, the Na+ concentration on the outside of the cell membrane is greater than that on the inside, that is, [Na+]o > [Na+]i. If the resting membrane were somewhat permeable to Na+, both the concentration and the electric gradient would support an inward movement of Na+ across the cell membrane, with a resultant decrease in the electronegativity of the inner surface of the cell membrane (Fig. 59–1B).
If such an inward movement of Na+ went unchecked, the RMP would be expected to decrease to a level lower than that actually observed, and the concentration gradient for Na+ might become reversed. In order to maintain a steady-state ion distribution across the cell membrane with [K+]o < [K+]i and [Na+]o > [Na+]I, and to prevent the transmembrane potential from becoming lower than the measured ureteral RMP, an active mechanism capable of extruding Na+ from within the cell against a concentration and electrochemical gradient is required (Fig. 59–1C). Such an outward Na+ pump that is coupled with an inward movement of K+ derives its energy requirements from the dephosphorylation of ATP (Casteels, 1970). Na+/Ca2+ exchange also may play a role in Na+ extrusion, especially when the Na+ pump is inhibited (Aickin, 1987; Aickin et al, 1987; Lamont et al, 1998).
The dynamic processes illustrated in Figure 59–1 enable the ureter in its resting state to maintain a relatively low RMP. In addition to the mechanisms described, the distribution of chloride ions (Cl−) across the cell membrane and the relative permeability of the membrane to Cl− may be factors in the maintenance of the RMP in the ureter and other smooth muscles (Kuriyama, 1963; Washizu, 1966).
Action Potential
The changes that occur are diagrammatically depicted in Figure 59–2. If a stimulus is very weak, as shown by arrow a, the transmembrane potential may remain unchanged. A slightly stronger, yet subthreshold, stimulus may result in an abortive displacement of the transmembrane potential, but not to such a degree that an action potential is generated (arrow b). If the stimulus is strong enough to decrease the transmembrane potential to the threshold potential, the cell becomes excited and develops an action potential (arrow c). The action potential, which is the primary event in the conduction of the peristaltic impulse, has the capability to act as the stimulus for excitation of adjacent quiescent cells and, through a complicated chain of events, gives rise to the ureteral contraction.
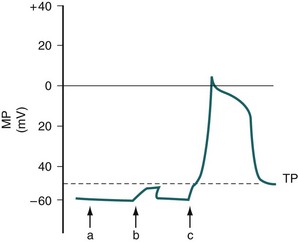
(From Weiss RM. Ureteral function. Urology 1978;12:114.)
When the ureteral cell is excited, its membrane loses its preferential permeability to K+ and becomes more permeable to Ca2+ ions that move inward across the cell membrane, primarily through fast L-type Ca2+ channels and give rise to the upstroke of the action potential (Fig. 59–3A) (Kobayashi, 1965; Kuriyama and Tomita, 1970; Imaizumi et al, 1989; Lang, 1989, 1990; Sui and Kao, 1997a, b; Smith et al, 2002). This channel is inhibited by the calcium channel blocker nifedipine and by cadmium ions (Cd2+), and it is potentiated by barium ions (Ba2+). As the positively charged Ca2+ ions move inward across the cell membrane, the inside of the membrane becomes less negative relative to the outside and may even become positive at the peak of the action potential, a state referred to as overshoot. Na+ ions also may play a role in the upstroke of the ureteral action potential (Kobayashi, 1964, 1965; Muraki et al, 1991). The rate of rise of the upstroke of the ureteral action potential is relatively slow, 1.2 ± 0.06 V/sec in the cat (Kobayashi, 1969). This compares with a 610 V/sec rate of rise in dog cardiac Purkinje fibers (Draper and Weidmann, 1951) and a 740 V/sec rate of rise in skeletal muscle (Ferroni and Blanchi, 1965). The slow rate of upstroke rise of the ureteral action potential accounts for the slow conduction velocity in the ureter.
After reaching the peak of its action potential, the ureter maintains its potential for a period of time (plateau of the action potential) before the transmembrane potential returns to its resting level (repolarization) (Kuriyama et al, 1967). The plateau phase of the guinea pig action potential is superimposed with multiple oscillations, a phenomenon not observed in the rat, rabbit, or cat (Fig. 59–4) (Bozler, 1938). The plateau phase appears to depend on the persistence of an inward Ca2+ current and on Na+ influx through a voltage-dependent Na+ channel (see Fig. 59–3A) (Kuriyama and Tomita, 1970; Imaizumi et al, 1989; Sui and Kao, 1997b). Also involved in the plateau formation is the maintenance of depolarization by an inward calcium-dependent chloride current (ICl(Ca)) which is countered by outward voltage-gated (I70) and Ca2+-activated K+ currents (I(KCa)) (Smith et al, 2002). There are species differences in the ionic currents involved in the formation of the action potential, with the Ca2+-activated chloride current being present in the rat but not in the guinea pig ureter. The inward Cl− current can be inhibited by niflumic acid and by Ba2+ (Smith et al, 2002). The oscillations on the plateau of the guinea pig action potential appear to depend on the repetitive activation of an inward Ca2+ current (Kuriyama and Tomita, 1970) and of a Ca2+-dependent outward K+ current (Imaizumi et al, 1989). Prolongation of the inward calcium current and the duration of the action potential correlate with an increased force of contraction (Burdyga and Wray, 1999b).
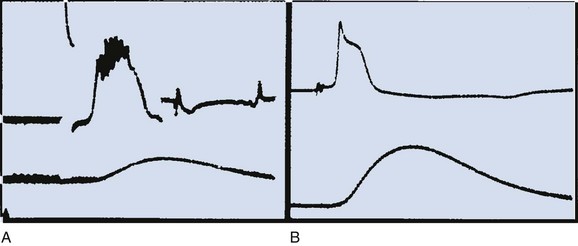
(A and B, From Weiss RM. Ureteral function. Urology 1978;12:114.)
The activation of a Ca2+-dependent K+ current that is involved in repolarization is mainly due to Ca2+ release from the endoplasmic reticulum, which is triggered by the influx of extracellular Ca2+ through voltage-dependent Ca2+ channels. The increase in intracellular Ca2+ concentration during the upstroke and plateau of the action potential finally may activate the outward Ca2+-dependent K+ current (IK(Ca)) to such a degree that repolarization occurs with return of the transmembrane potential to its resting level (see Fig. 59–3A) (Imaizumi et al, 1989; Sui and Kao, 1997c). This IK(Ca) is sensitive to inhibition by tetraethylammonium (TEA). Based on this, TEA increases the amplitude and duration of in-vitro ureteral contractions (Floyd et al, 2008). A voltage-dependent, Ca2+ insensitive outward K+ current (ITO) also appears to be involved in the repolarization (Lang, 1989; Imaizumi et al, 1990). These currents are TEA-insensitive and 4-aminopyridine (4-AP)–sensitive. In the rat, but not the guinea pig, ureter there is a late TEA-, Cd2+– and Ca2+-insensitive outward K+ current that also is involved in the repolarization process. The duration of the action potential in the cat ranges from 259 to 405 milliseconds (Kobayashi and Irisawa, 1964).
Pacemaker Potentials and Pacemaker Activity
Electric activity arises in a cell either spontaneously or in response to an external stimulus. If the activity arises spontaneously, the cell is referred to as a pacemaker cell. Pacemaker fibers differ from nonpacemaker fibers in that their transmembrane resting potential is lower (less negative) than that of nonpacemaker fibers (Lang and Zhang, 1996) and does not remain constant but, rather, undergoes a slow spontaneous depolarization (see Fig. 59–3B). If the spontaneously changing membrane potential reaches the threshold potential, the upstroke of an action potential occurs. The ionic conduction underlying pacemaker activity in the upper urinary tract is due to the opening and slow closure of voltage-activated L-type Ca2+ channels that are amplified by prostanoids (Santicioli et al, 1995a). This is opposed by the opening and closure of voltage and Ca2+-dependent K+ channels. It has been suggested that prostaglandins and excitatory tachykinins, released from sensory nerves, help maintain autorhythmicity in the upper urinary tract through maintenance of Ca2+ mobilization (Lang et al, 2002). Tetrodotoxin and blockers of the autonomic nervous system, both parasympathetic and sympathetic, have little effect on peristalsis, suggesting that autonomic neurotransmitters have a small role in maintaining pyeloureteral motility (Lang et al, 2001, 2002). Changes in the frequency of action potential development may result from a change in the level of the threshold potential, a change in the rate of slow spontaneous depolarization of the resting potential, or a change in the level of the resting potential.
Gosling and Dixon (1971, 1974) provided morphologic evidence of specialized pacemaker tissue in the proximal portion of the urinary collecting system and described species differences. In species with a multicalyceal system, such as the pig, sheep, and human, the “pacemaker cells” are located near the pelvicalyceal border (Dixon and Gosling, 1973). In species with a unicalyceal system, such as the dog, cat, rat, rabbit, and guinea pig, the “pacemaker cells” extend from the pelvicalyceal border to the ureteropelvic junction (UPJ). These atypical smooth muscle cells that give rise to pacemaker activity in the rat and guinea pig, in contrast to typical smooth muscle cells, have less than 40% of their cellular area occupied by contractile elements and demonstrate sparse immunoreactivity for smooth muscle and actin (Klemm et al, 1999; Lang et al 2001). These spindle-shaped cells are 90 to 230 µm in length, and their electrical activity consists of simple waveforms of alternating depolarizing and repolarizing phases that occur at a relatively rapid frequency of 8 to 15/min (Fig. 59–5A) (Tsuchida and Suzuki, 1992; Klemm et al, 1999). Pacemaker potentials have a lower resting membrane potential (RMP), a slower rate of rise, and a lower amplitude than action potentials recorded from nonpacemaker cells. In the guinea pig, these atypical, presumably pacemaker cells comprise greater than 80% of the cells at the pelvicalyceal junction, about 15% of the cells in the proximal renal pelvis, but are not present in the distal renal pelvis or ureter (Klemm et al, 1999). Electrical recordings correlate with histologic findings in that pacemaker potentials were not observed in the distal renal pelvis or ureter (Klemm et al, 1999).
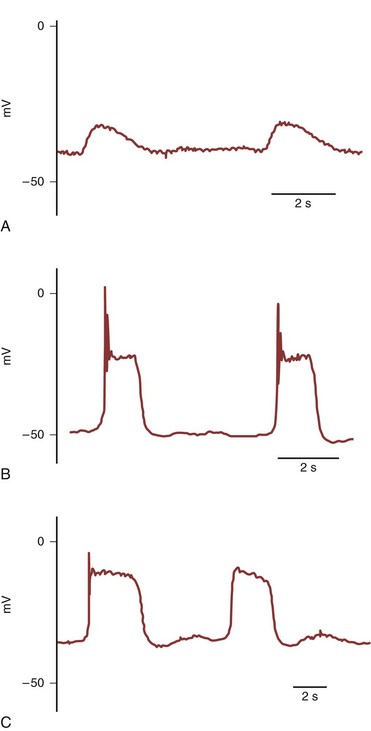
(Modified with permission from Klemm MF, Exintaris B, Lang RJ. Identification of the cells underlying pacemaker activity in the guinea pig upper urinary tract. J Physiol [Lond] 1999;519:867.)
Driven action potentials that fire at lower frequency (3 to 5/min) than pacemaker potentials are recorded from longer (150 to 400 µm) spindle-shaped typical smooth muscle cells (Fig. 59–5B) (Klemm et al, 1999). Most muscle cells of the ureter (100%), distal renal pelvis (97.5%), and proximal renal pelvis (83% are typical nonpacemaker smooth muscle cells with typical action potentials. Lang et al (1998) described fibroblast-like cells resembling the interstitial cells of Cajal (ICC), which serve as pacemaker cells in the intestine, in the proximal portion of the guinea pig renal pelvis. These “ICC-like” cells are irregularly shaped with oval nuclei and many branching interconnecting processes and contain numerous mitochondria, caveolae, and prominent endoplastic reticulum. The ICC-like cells in the guinea pig are not immunoreactive for α-smooth muscle actin, which is present in typical smooth muscle cells, or for c-KIT, a tyrosine kinase receptor that is expressed in intestinal ICC pacemaker cells (Klemm et al, 1999). The immunoreactivity to c-KIT appears to be species specific, because ICC-like cells that are immunoreactive to antibodies raised against the c-KIT proto-oncogene are present in the upper urinary tract of a number of mammals (Lang and Klemm, 2005; Metzger et al, 2005). Electrical recordings from these cells demonstrate action potentials with properties intermediate to the pacemaker and driven action potentials. These intermediate action potentials in the guinea pig have a single spike, a plateau without the superimposed spikes seen in driven action potentials and a rapid repolarization phase (Fig. 59–5C). Intermediate action potentials are noted in 11% to 17% of cells at the pelvicalyceal junction and the proximal and distal renal pelvis (Lang et al, 2002). These ICC-like cells in the upper urinary tract do not appear to be primary pacemaker cells but, rather, may provide for preferential conduction of electrical signals from pacemaker cells to typical smooth muscle cells of the renal pelvis and ureter (Klemm et al, 1999). In the mouse ureteropelvic junction, c-KIT–positive ICC-like cells have been identified that showed high-frequency spontaneous transient inward currents that often occurred in bursts to sum and produce long-lasting large inward currents (Lang et al, 2007b). It is postulated that in the absence of a proximal pacemaker drive these ICC-like cells could act as pacemaker cells and trigger contractions in adjacent smooth muscle cells in the ureteropelvic junction. Thus both atypical smooth muscle cells and ICC-like cells both may play a pacemaker role in the initiation and propagation of pyeloureteric peristalsis (Lang et al, 2006; Lang et al, 2007a).
c-KIT is a tyrosine kinase receptor that promotes cell migration and proliferation of melanoblasts, hematopoietic progenitors and primordial germ cells. Mice expressing mutant inactivating c-Kit alleles, lack intestinal interstitial cells of Cajal (ICC), have abnormal intestinal peristalsis, and develop bowel obstruction, showing that c-Kit is important in the development of pacemaker activity and peristalsis of the gut (Der-Silaphet et al, 1998). Pezzone et al (2003) identified c-Kit positive cells in the mouse ureter. They suggested that the difference from previous studies in the guinea pig upper urinary tract, in which c-Kit–positivity was not identified in ICC-like cells (Klemm et al, 1999), may be due to species differences, the c-Kit antibody used, and/or the fixation methods. c-Kit gene expression was noted to be upregulated in the embryonic murine ureter prior to its development of unidirectional peristaltic contractions (David et al, 2005). Incubation of isolated cultured embryonic murine ureters with antibodies that neutralize c-Kit activity altered ureteral morphology and inhibited unidirectional peristalsis. These data suggest that c-Kit–containing cells, which are probably ICC-like cells, have an important role in pyeloureteric peristalsis. c-Kit–positive cells have been identified in the human ureter (Metzger et al, 2004; van der Aa et al, 2004) and in the human UPJ (Solari et al, 2003). In the presence of obstruction, c-Kit–positive cells ICC-like cells at the UPJ were sparse or absent (Solari et al, 2003).
Bozler (1942), using small extracellular surface electrodes, demonstrated the characteristic slow spontaneous depolarization of pacemaker-type fibers in the proximal portion of the isolated ureter of a unicalyceal upper collecting system. In a multicalyceal kidney, Morita and associates (1981), using extracellular electrodes, recorded low-voltage potentials that appeared to be pacemaker potentials from the border of the pig minor calyces and the major calyx with the contraction rhythm varying between each calyx. Multiple pacemakers fire simultaneously as coupled oscillators, or individually, as pacemaker activity shifts from one site to another along the renal pelvis of the unicalyceal kidney or the pelvicalyceal border of the multicalyceal pig and sheep kidney (Golenhofen and Hannappel, 1973; Constantinou et al, 1977; Constantinou and Yamaguchi, 1981; Lammers et al, 1996).
Although the primary pacemaker for ureteral peristalsis is located in the proximal portion of the collecting system, other areas of the ureter may act as latent pacemakers. Under normal conditions, the latent pacemaker regions are dominated by activity arising at the primary pacemaker sites. When the latent pacemaker site is freed of its domination by the primary pacemaker, it, in turn, may act as a pacemaker. To demonstrate latent pacemaker sites, Shiratori and Kinoshita (1961) transected the in-vivo dog ureter at various levels. Before transection, peristaltic activity arose proximally from the primary pacemaker. When the ureter was transected at the UPJ, antiperistaltic waves of lower frequency than the previous normoperistaltic waves originated from the UVJ. Division of the ureter at the UVJ did not affect the normoperistaltic waves. After division of the midureter, the normoperistaltic waves in the upper segment remained unchanged, and the lower segment demonstrated antiperistaltic waves, which originated at the UVJ at a frequency less than that of the normoperistaltic waves in the upper segment. Thus cells at the UVJ of the dog may act as pacemaker cells when freed of control from the primary proximally located pacemaker. Latent pacemaker cells are present in all regions of the ureter (Imaizumi et al, 1989; Meini et al, 1995).
Propagation of Electric Activity
Excitable cells possess resistive and capacitative membrane properties similar to those of a cable or core conductor. The transverse resistance of the membrane is higher than the longitudinal resistance of the extracellular or intracellular fluid; this allows current resulting from a stimulus to propagate along the length of the fibers. The spread of current is referred to as electrotonic spread (Hoffman and Cranefield, 1960). The space constant (λ) determines the degree to which the electrotonic potential dissipates with increasing distance from an applied voltage. In a cable, this relation is expressed by
where X is the distance from the applied voltage, P is the displacement of the membrane potential at X, Po is the displacement of the membrane potential at the site of the applied voltage, e is the base of the natural logarithm, and λ is the space constant. Thus the electrotonic potential decreases by 1/e in one space constant. The space constant of the guinea pig ureter measured by extracellular stimulation is 2.5 to 3 mm (Kuriyama et al, 1967).
The time constant τm is expressed by
where R is the membrane resistance and C is the membrane capacity. The time constant τm signifies that a small displacement of potential is decreased by 1/e of its value in one τm. The time constant of the guinea pig ureter measured by extracellular stimulation is 200 to 300 milliseconds (Kuriyama et al, 1967).
The ureter acts as a functional syncytium. Engelmann (1869, 1870) showed that stimulation of the ureter produces a contraction wave that propagates proximally and distally from the site of stimulation. Under normal conditions, electric activity arises proximally and is conducted distally from one muscle cell to another across areas of close cellular apposition referred to as intermediate junctions (Uehara and Burnstock, 1970; Libertino and Weiss, 1972). The similarity of these close cellular contacts to nexuses, which have been shown to be low-resistance pathways for cell-to-cell conduction in other smooth muscles (Barr et al, 1968), suggests that a similar mechanism for conduction may be present in the ureter. Gap junctions, consisting of groups of channels in the plasma membrane of adjacent smooth muscle cells, enable exchange of ions and small molecules and play a role in electrical coupling between adjacent cells and electromechanical coupling (Gabella, 1994; Santiciolli and Maggi, 2000). 18β-glycyrrhetinic acid, a gap junction inhibitor, inhibits cell-to-cell electrical coupling in the guinea pig renal pelvis and ureter and dissociates electrical and mechanical events (Santicioli and Maggi, 2000). Conduction velocity in the ureter is 2 to 6 cm/sec (Kobayashi, 1964; Kuriyama et al, 1967); it has been shown to vary with temperature, the time interval between stimuli (van Mastrigt et al, 1986), and the pressure within the ureter (Tsuchiya and Takei, 1990). This compares to conduction velocities ranging from 1.5 to 2 m/sec in cardiac Purkinje fibers (Rosen et al, 1981) and from 10 to 100 m/sec in the dorsal and ventral roots of the spinal cord (Biscoe et al, 1977). Conduction in the ureter is similar to that in cardiac tissue, even to the extent that the Wenckebach phenomenon (a partial conduction block) has been demonstrated in the ureter, as it has been in specialized cardiac fibers (Weiss et al, 1968).
Contractile Activity
The contractile event is dependent on the concentration of free sarcoplasmic Ca2+ in the region of the contractile proteins, actin and myosin. Any process that results in a significant increase in Ca2+ in the region of the contractile proteins favors the development of a contraction; any process that results in a significant decrease in Ca2+ in the region of the contractile proteins favors relaxation (Fig. 59–6).
Contractile Proteins
In smooth muscle, on the other hand, Ca2+ appears to act as an activator. The most widely accepted theory suggests that phosphorylation of myosin is involved in the contractile process and that a troponin-like system does not constitute the primary regulatory mechanism, as it does in skeletal muscle. With excitation, there is a transient increase in the sarcoplasmic Ca2+ concentration from its steady-state concentration of 10−8 to 10−7 M to a concentration of 10−6 M or higher. At this higher concentration, Ca2+ forms an active complex with the Ca2+–binding protein calmodulin (Watterson et al, 1976; Cho et al, 1988). Calmodulin without Ca2+ is inactive (Fig. 59–7). The Ca2+–calmodulin complex activates a calmodulin-dependent enzyme, myosin light-chain kinase (see Fig. 59–7). The activated myosin light-chain kinase, in turn, catalyzes the phosphorylation of the 20,000-D light chain of myosin (Fig. 59–8). Phosphorylation of the myosin light chain allows actin to activate myosin Mg2+-ATPase activity, leading to hydrolysis of ATP and the development of smooth muscle tension or shortening (Fig. 59–9). Actin cannot activate the ATPase activity of the dephosphorylated myosin light chain.
Evidence indicates that phosphorylation of the enzyme myosin light-chain kinase by a cAMP-dependent protein kinase decreases myosin light-chain kinase activity by decreasing the affinity of this enzyme for calmodulin (Adelstein et al, 1981).
Although Ca2+ is required for most smooth muscle contractile events, there is evidence in some smooth muscles, including in the rabbit and human bladder, that Ca2+-independent contractions can occur (Yoshimura and Yamaguchi, 1997). Carbachol, a muscarinic cholinergic agonist, and phorbol ester, which activate protein kinase C (PKC), can induce contraction in Ca2+-depleted bladder strips that can be inhibited by a PKC inhibitor, H7. It is suggested that activation of PKC, coupled with agonist stimulation of the muscarinic receptor, can induce a Ca2+-independent contraction.
Calcium and Excitation-Contraction Coupling
The mechanical event of ureteral peristalsis follows an electric event to which it is related. The Ca2+ involved in the ureteral contraction is derived from two main sources. Because smooth muscle cells have a very small diameter, the inward movement of extracellular Ca2+ into the cell through L-type Ca2+ channels during the upstroke of the action potential provides a significant source of sarcoplasmic Ca2+ (Brading et al, 1983; Hertle and Nawrath, 1989; Yoshida et al, 1992; Maggi et al, 1994a; Maggi and Giuliani, 1995; Floyd et al, 2008) (see Fig. 59–6). This inward movement across the cell membrane is the major source of calcium used for contraction in most smooth muscles. Na+-Ca2+ exchange, with an outward movement of Na+ and an inward movement of Ca2+, also plays a role in the ureteral contraction (Lamont et al, 1998). Furthermore, in response to an excitatory impulse, Ca2+ release from tightly bound storage sites (i.e., the endoplasmic or sarcoplasmic reticulum) also increases the Ca2+ concentration in the sarcoplasm (Burdyga et al, 1998; Burdyga and Wray, 1999a; Lang et al, 2002). Calcium may be released from the sarcoplasmic reticulum (SR) of smooth muscle by an inositol 1,4,5-trisphosphate (IP3)–induced release mechanism or by Ca2+-induced Ca2+ release (CICR) (Somlyo and Somlyo, 1994). These processes appear to be species dependent. CICR, which involves ryanodine receptors, appears to be the sole mechanism for calcium release from the SR in the guinea pig ureter, whereas the SR stored in the rat ureter appears to be exclusively under the control of IP3 receptors (Burdyga et al, 1995). IP3 and ryanodine receptors are expressed in the human ureter (Floyd et al, 2008). The opening of caffeine-sensitive ryanodine receptors produces small local elevations of Ca2+ that are termed Ca2+ sparks (Nelson et al, 1995). In addition to providing a source of calcium for contraction, Ca2+ released from the SR activates Ca2+–sensitive surface membrane channels and modulate membrane excitability (Imaizumi et al, 1989; Carl et al, 1996). Both calcium-activated outward potassium currents (KCa), or STOCS (spontaneous transient outward currents), and calcium-activated inward chloride currents (ClCa), or STICS (spontaneous transient inward currents), have been identified in smooth muscles. These currents affect membrane potential and thus affect calcium entry through L-type Ca2+ channels in the membrane. The Ca2+–activated chloride currents are present in rat but not guinea pig ureteral smooth muscle (Burdyga and Wray, 2002). Thus some Ca2+ released from SR results in contraction, but it has been shown that caffeine-induced release of SR Ca2+, in the form of Ca2+ sparks, increases outward potassium currents (KCa), or STOCS, with an inhibitory effect on action potentials and contractility (Borisova et al, 2007). At least in the guinea pig ureter, this increase in the outward potassium current hyperpolarizes the membrane and determines the refractory period, which is important in determining the frequency of ureteral peristalsis and may have a protective effect on the kidney (Burdyga and Wray, 2005).
Support for use of a dual source of Ca2+ in the ureter has been provided by Vereecken and coworkers (1975), who noted that it took approximately 45 minutes for spontaneous contractions of isolated guinea pig ureters to cease when the tissue was placed in a Ca2+-free medium. They interpreted this to indicate that some of the Ca2+ involved in the contractile process is derived from tightly bound intracellular stores. They also noted that recovery of the contractile response to electric stimuli was almost immediate when the tissue was returned to a physiologic solution containing a normal concentration of Ca2+. This suggests that free extracellular Ca2+ entering the cell during excitation also provides a source of Ca2+ for the contractile machinery. A similar conclusion was reached by Hong and associates (1985). There is, however, some evidence that Ca2+ release from the sarcoplasmic reticulum may not play a significant role in ureteral contractions, at least in the guinea pig (Maggi et al, 1994a, 1995, 1996), and some perturbations of contractility may be related to movements of ions other than Ca2+. The increase in developed force in the guinea pig ureter, with intracellular acidification and decrease with intracellular alkalinization, appear to result from modulation of outward K+ currents rather than effects on inward Ca2+ currents (Smith et al, 1998). That is, alkalinization (increasing intracellular pH) enhances outward K+ currents, and this reduces excitability; acidification has the opposite effect. Relaxation results from a decrease in the concentration of free sarcoplasmic Ca2+ in the region of the contractile proteins. The decrease in sarcoplasmic Ca2+ can result from the uptake of Ca2+ into intracellular storage sites (Maggi et al, 1994a, 1995) or from extrusion of Ca2+ from the cell (Burdyga and Magura, 1988).
In addition to the Ca2+– signaling cascade that affects contractility, there is a Rho/Rho-kinase signaling pathway that affects contractility by altering the Ca2+-sensitivity of the contractile system (Somlyo and Somlyo, 2003). The Rho-kinase pathway is involved in ureteral contractions in a number of species (Levent and Buyukafsar, 2004; Shabir et al, 2004; Hong et al, 2005). Rho-kinase inhibits myosin phosphatase by phosphorylation of its regulatory subunit, which prevents dephosphorylation of myosin light chain, which, in turn, leads to Ca2+ sensitization of the smooth muscle with a subsequent increase in contractility. Y-27632, an inhibitor of Rho-kinase, decreases both spontaneous and EFS-induced contractile responses of in vitro human ureteral segments (Hong et al, 2005). Y-27632 inhibits kinase activity by competing with ATP for binding to the Rho-kinases.
Urothelial Effects on Contractile Activity
Furchgott (1999) showed that the endothelium produced a factor that had a relaxing action on the smooth muscle layer of blood vessels. This factor was originally termed endothelium-derived relaxing factor (EDRF) and was subsequently shown to be nitric oxide (NO). Mastrangelo et al (2003) showed that the urothelium of rat ureter produced NO, which inhibited contractile responses of the rat ureter. Recently in the rat ureter, it was shown that the urothelium inhibited spontaneous contractions of isolated ureteral segments and that removal of the urothelium potentiated the stimulatory effects of neurokinin A, vasopressin, carbachol, bradykinin, and angiotensin II (Mastrangelo and Iselin, 2007). In intact ureteral segments, cyclooxygenase inhibitors potentiated the stimulatory effects of neurokinin A, vasopressin, carbachol, bradykinin, and angiotensin II. Cyclooxygenase inhibitors had no effect on the responses to these agents in urothelium-free ureters. These data suggest that the inhibitory effects of the urothelium on ureteral contractile events may involve the participation of a urothelial cyclooxygenase product such as prostacyclin.
Second Messengers
The functional response to a number of hormones, neurotransmitters, and other agents is mediated by means of second messengers. The agonist, or first messenger, interacts with a specific membrane-bound receptor (Alquist, 1948; Furchgott, 1964); the agonist-receptor complex then activates or inactivates an enzyme, which leads to alteration of a second messenger within the cell. These second messengers include cyclic AMP (cAMP), cyclic GMP (cGMP), Ca2+, inositol 1,4,5-trisphosphate (IP3), and diacylglycerol (DG). They mediate the functional response to the agonist (first messenger) through a process that frequently involves protein phosphorylation.
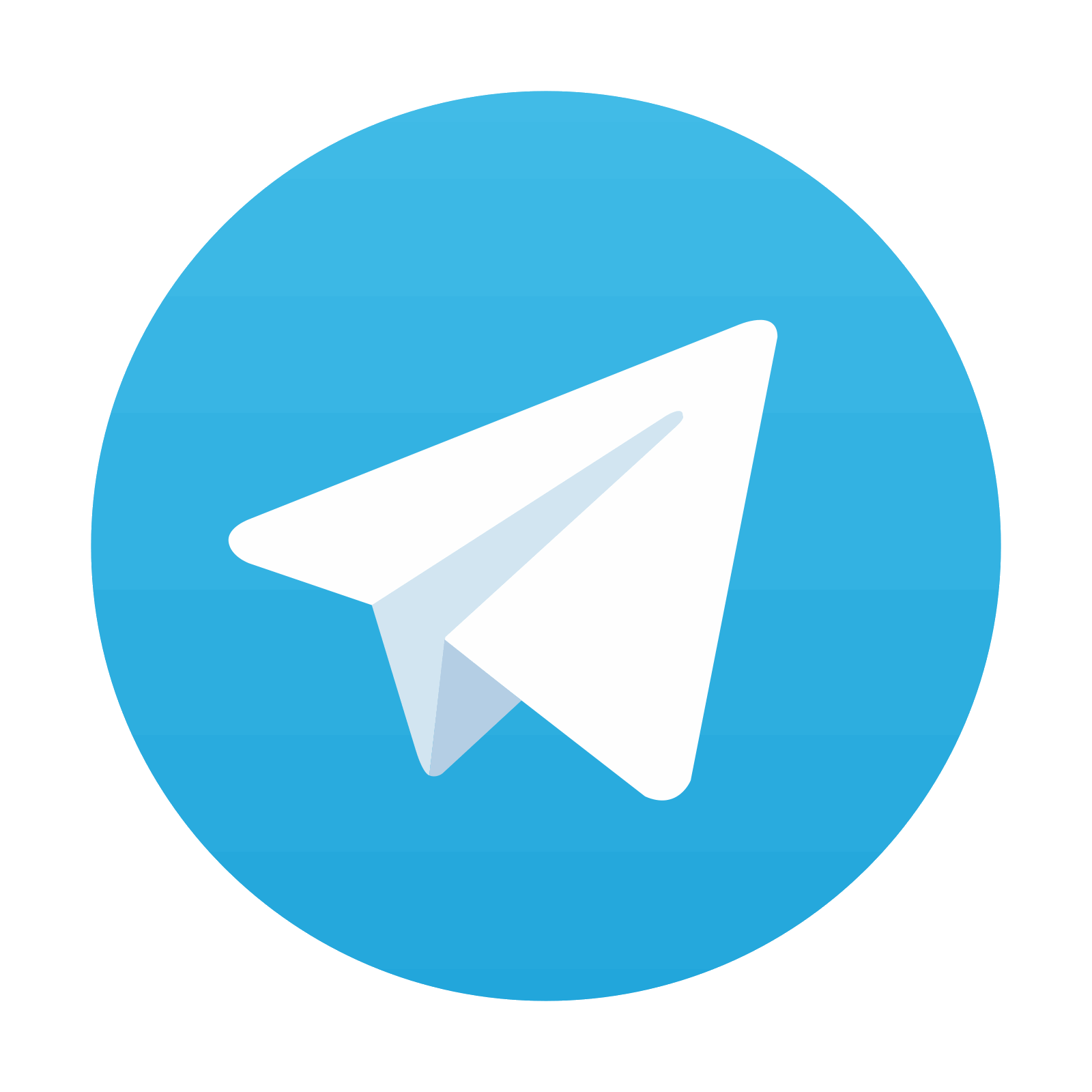
Stay updated, free articles. Join our Telegram channel
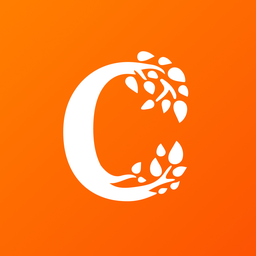
Full access? Get Clinical Tree
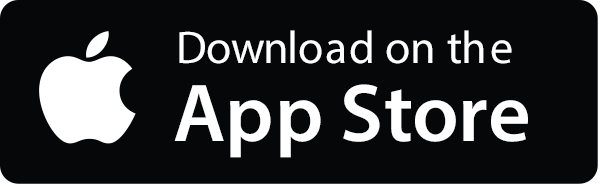
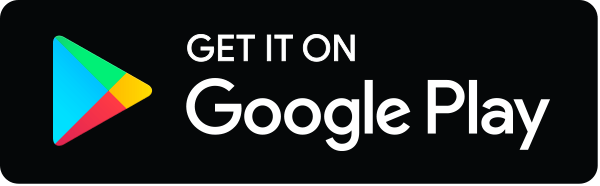