John M. Park, MD
The study of embryology provides a useful foundation for the understanding of definitive human anatomy and various congenital disease processes. During the past few decades, a torrent of molecular information and novel experimental techniques has revolutionized the field of embryology, and the knowledge base continues to expand at an exponential rate. From the urologic surgeon’s perspective, however, the classic, descriptive aspects of anatomic embryology continue to serve as an important reference point from which various congenital problems are solved clinically. The aim of this chapter is to provide a concise presentation of the essential facts of normal genitourinary system development, clarifying the important anatomic features and supplementing them with updated molecular information. Deliberate efforts have been made to separate the ever-expanding molecular information from that of the descriptive, anatomic embryology to keep the main “story” of genitourinary system development clear and understandable from a surgical point of view (Fig. 111–1). To help with visualization of the key events, various schematic drawings are provided. The goal of this chapter is not to provide potential explanations for every congenital defect that might occur in the genitourinary system but to select pertinent examples highlighting the fundamental concepts and principles.
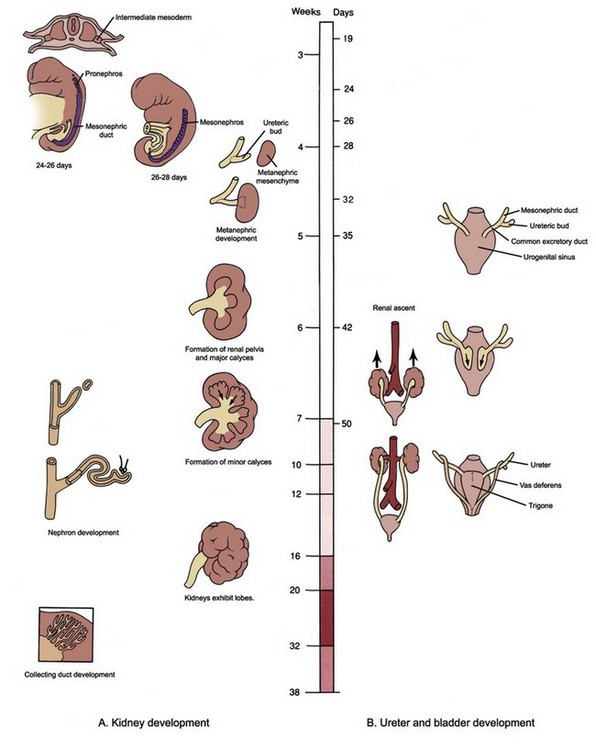
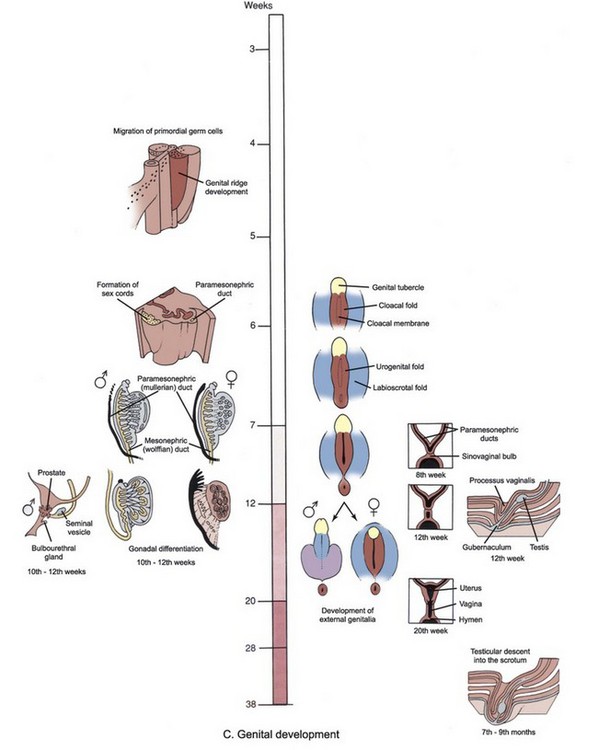
Figure 111–1 A to C, Time line and overview of genitourinary system development.
(Modified from Larsen WJ. Human embryology. New York: Churchill Livingstone; 1997.)
Kidney Development
Early Events
Mammals develop three kidneys in the course of intrauterine life. The embryonic kidneys are, in order of their appearance, the pronephros, the mesonephros, and the metanephros. The first two kidneys regress in utero, and the third becomes the permanent kidney. Embryologically, all three kidneys develop from the intermediate mesoderm. As the notochord and neural tube develop, the mesoderm located on either side of the midline differentiates into three subdivisions: paraxial (somite), intermediate, and lateral mesoderm (Fig. 111–2). As the embryo undergoes transverse folding, the intermediate mesoderm separates away from the paraxial mesoderm and migrates toward the intraembryonic coelom (the future peritoneum). At this time there is a progressive craniocaudal development of the bilateral longitudinal mesodermal masses, called nephrogenic cords. Each cord is seen bulging from the posterior wall of the coelomic cavity, producing the urogenital ridge.
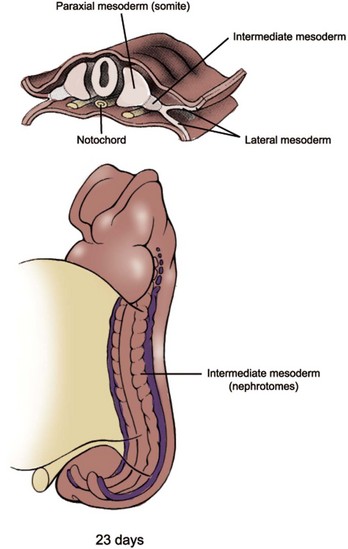
(Modified from Larsen WJ. Human embryology. New York: Churchill Livingstone; 1997.)
Pronephros and Mesonephros
The mammalian pronephros is a transitory, nonfunctional kidney, analogous to that of primitive fish. In humans, the first evidence of pronephros is seen late in the third week, and it completely degenerates by the start of the fifth week. The pronephros develops as five to seven paired segments in the region of the future neck and thorax (Fig. 111–3A). Development of the pronephric tubules starts at the cranial end of the nephrogenic cord and progresses caudally. As each tubule matures it immediately begins to degenerate along with the segment of the nephric duct to which the tubules are attached.
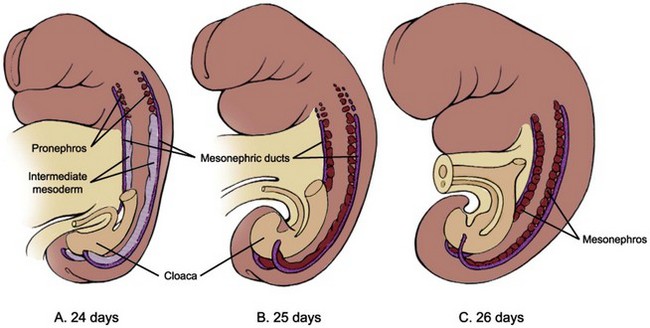
(Modified from Larsen WJ. Human embryology. New York: Churchill Livingstone; 1997.)
The second kidney, the mesonephros, is also transient, but in mammals it serves as an excretory organ for the embryo while the definitive kidney, the metanephros, begins its development (see Fig. 111–3B and C). There is a gradual transition from the pronephros to the mesonephros at about the 9th and 10th somite levels. Development of the nephric ducts (also called the wolffian ducts) precedes the development of the mesonephric tubules. The nephric ducts can be seen as a pair of solid longitudinal tissue condensations at about the 24th day, developing parallel to the nephrogenic cords in the dorsolateral aspect of the embryo. Its blind distal ends grow toward the primitive cloaca and soon fuse with it at about the 28th day. As the ducts fuse with the cloaca they begin to form a lumen at the caudal end. This process of canalization then progresses cranially in a reverse direction, transforming the solid tissue condensations into the definitive nephric ducts with excretory capability. Soon after the appearance of the nephric ducts during the 4th week, mesonephric vesicles begin to form. Initially, several spherical masses of cells are found along the medial side of the nephrogenic cords at the cranial end. This differentiation progresses caudally and results in the formation of 40 to 42 pairs of mesonephric tubules, but only about 30 pairs are seen at any one time because the cranially located tubules start to degenerate starting at about the 5th week. By the 4th month, the human mesonephros has almost completely disappeared, except for a few elements that persist into maturity as part of the reproductive tract. In males, some of the cranially located mesonephric tubules become the efferent ductules of the testes. The epididymis and vas deferens are also formed from the nephric (wolffian) ducts. In females, remnants of cranial and caudal mesonephric tubules form small, nonfunctional mesosalpingeal structures termed the epoöphoron and paroöphoron.
Metanephros
The definitive kidney, or the metanephros, forms in the sacral region as a pair of new structures, called the ureteric buds, sprout from the distal portion of the nephric duct and come in contact with the condensing blastema of metanephric mesenchyme at about the 28th day (Fig. 111–4). The ureteric bud penetrates the metanephric mesenchyme and begins to divide dichotomously. The tip of the dividing ureteric bud, called the ampulla, interacts with the metanephric mesenchyme to induce formation of future nephrons via mesenchymal-epithelial interaction. As the ureteric bud divides and branches, each new ampulla acquires a caplike condensation of metanephric mesenchyme, thereby giving the metanephros a lobulated appearance (Fig. 111–5).
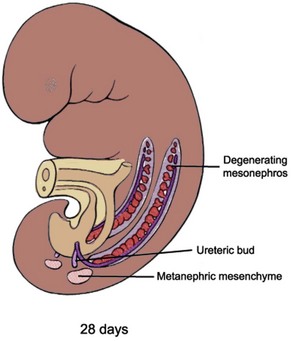
(Modified from Larsen WJ. Human embryology. New York: Churchill Livingstone; 1997.)
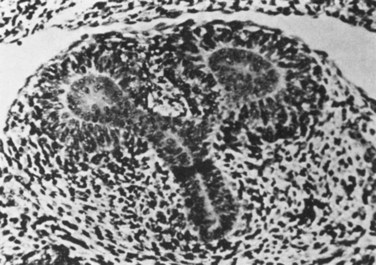
(From Potter EL. Normal and abnormal development of the kidney. Chicago: Year Book Medical Publishers; 1972.)
The ureteric bud and metanephric mesenchyme exert reciprocal inductive effects toward each other, and the proper differentiation of these primordial structures depends on these inductive signals (see Molecular Mechanism of Kidney Development, later). The metanephric mesenchyme induces the ureteric bud to branch and divide, and, in turn, the ureteric bud induces the metanephric mesenchyme to condense and undergo mesenchymal-epithelial conversion. The nephron, which consists of the glomerulus, proximal tubule, loop of Henle, and distal tubule, is thought to derive from the metanephric mesenchyme, while the collecting system, consisting of collecting ducts, calyces, pelvis and ureter, is formed from the ureteric bud (Fig. 111–6).
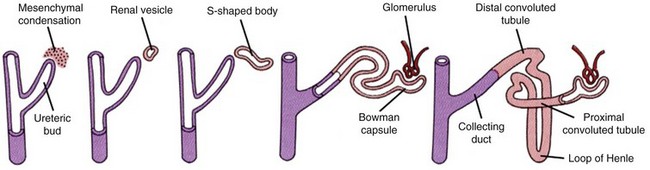
(Modified from Larsen WJ. Human embryology. New York: Churchill Livingstone; 1997.)
In principle, all nephrons are formed in the same way and can be classified into fairly well-defined developmental stages (Larsson et al, 1983) (Fig. 111–7). The metanephric mesenchyme first condenses to form a four- to five-cell layer condensate around the ampulla of the advancing ureteric bud. Near the interface of the ampulla and its adjacent ureteric branch, a cluster of cells separates from the condensate and forms an oval mass, called a pretubular aggregate. An internal cavity forms within the pretubular aggregate, at which point the structure is called a renal vesicle (stage I). Cells of the stage I renal vesicle are tall and columnar and are stabilized by their attachments to the newly formed basement membrane. It has not yet established a contact with the ampulla of the ureteric bud, but it subsequently forms a luminal connection. Multipotential precursors residing in renal vesicles ultimately give rise to all the epithelial cell types of the nephron (Herzlinger et al, 1992). Nephron segmentation into glomerular and tubular domains is initiated by the sequential formation of two clefts in the renal vesicle (stage II). Creation of a lower cleft, termed the vascular cleft, precedes formation of a comma-shaped body. Generation of an upper cleft in the comma-shaped body precedes formation of an S-shaped body. At this stage, the cup-shaped glomerular capsule is recognized in the lowest limb of the S-shaped tubule. Epithelial cells lining the inner wall of this cup will comprise the visceral glomerular epithelium, or podocyte layer. Cells lining the outer wall of the cup will form parietal glomerular epithelium, which lines the Bowman capsule. The glomerular capillary tuft is formed via recruitment and proliferation of endothelial and mesangial cell precursors. The rest of the S-shaped tubule develops into the proximal tubule, the loop of Henle, and the distal tubule. When the cup-shaped glomerular capsule matures into an oval structure the nephron has now passed into stage III of development. Now the nephron can be divided into identifiable proximal and distal tubules. The stage IV nephron is characterized by a round glomerulus that closely resembles the mature renal corpuscle. The morphology of the proximal tubule resembles that of a mature nephron, whereas the distal segments are still primitive. In some species, such as rodents, all stages of nephron development are present at birth, whereas in others, such as humans, all nephrons at birth are in varying steps of stage IV. Mesenchymal cells that do not become tubular epithelium give rise to interstitial stromal cells, which differentiate into a diverse population including fibroblasts, lymphocyte-like cells, and pericytes. Overall, these events are reiterated throughout the growing kidney so that older, more differentiated nephrons are located in the inner part of the kidney near the juxtamedullary region and newer, less differentiated nephrons are found at the periphery (Fig. 111–8). In humans, although renal maturation continues to take place postnatally, nephrogenesis is completed before birth at around 32 to 34 weeks of gestation.
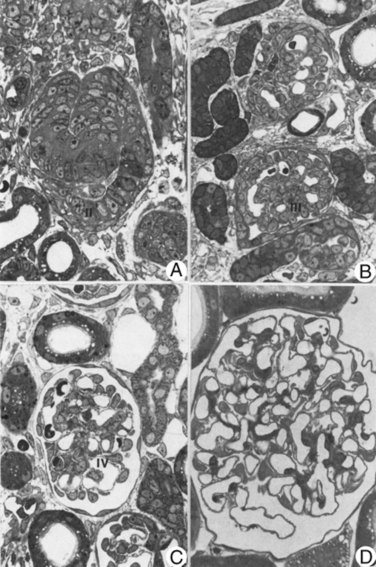
(Reproduced from Larsson L, Maunsbach AB. The ultrastructural development of the glomerular filtration barrier in the rat kidney: a morphometric analysis. J Ultrastruct Res 1980;72:392.)
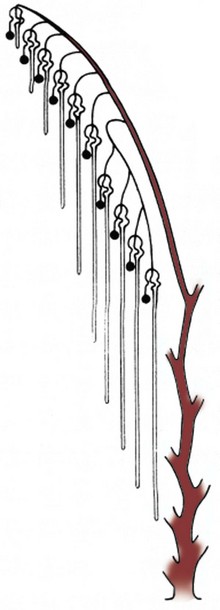
(Reproduced from Potter EL. Normal and abnormal development of the kidney. Chicago: Year Book Medical Publishers; 1972.)
Collecting System
The dichotomous branching of the ureteric bud determines the eventual pelvicalyceal patterns and their corresponding renal lobules (Cebrian et al, 2004) (Fig. 111–9). In humans, the first nine branch generations are formed by approximately 15 weeks’ gestation. By 20 to 22 weeks, ureteric bud branching is completed. Thereafter, collecting duct development occurs by extension of peripheral branch segments. Between 22 and 24 weeks of human fetal gestation the peripheral (cortical) and central (medullary) domains of the developing kidney are established. The renal cortex, which represents 70% of total kidney volume at birth, becomes organized as a relatively compact, circumferential rim of tissue surrounding the periphery of the kidney. The renal medulla, which represents 30% of total kidney volume at birth, has a modified cone shape with a broad base contiguous with cortical tissue. The apex of the cone is formed by convergence of collecting ducts in the inner medulla and is termed the papilla. Distinct morphologic differences emerge between collecting ducts located in the medulla compared with those located in the renal cortex. Medullary collecting ducts are organized into elongated linear arrays that converge centrally in a region devoid of glomeruli. In contrast, collecting ducts located in the renal cortex continue to induce metanephric mesenchyme. The most central segments of the collecting system, formed from the first five generations of ureteric bud branching, undergo remodeling by increased growth and dilatation of these tubules to form the pelvis and calyces.
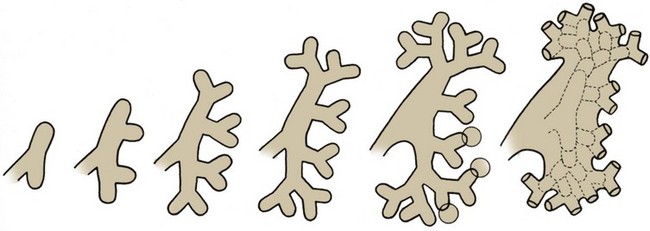
(Reproduced from Potter EL. Normal and abnormal development of the kidney. Chicago: Year Book Medical Publishers; 1972.)
Renal Ascent
Between the sixth and ninth weeks the kidneys ascend to a lumbar site just below the adrenal glands (Fig. 111–10). The precise mechanism responsible for renal ascent is not known, but it is speculated that the differential growth of the lumbar and sacral regions of the embryo plays a major role. As the kidneys migrate they are vascularized by a succession of transient aortic sprouts that arise at progressively higher levels. These arteries do not elongate to follow the ascending kidneys but instead degenerate and are replaced by successive new arteries. The final pair of arteries forms in the upper lumbar region and becomes the definitive renal arteries. Occasionally, a more inferior pair of arteries persists as accessory lower pole arteries. When the kidney fails to ascend properly its location becomes ectopic. If its ascent fails completely it remains as a pelvic kidney. The inferior poles of the kidneys may also fuse, forming a horseshoe kidney that crosses over the ventral side of the aorta. During ascent the fused lower pole becomes trapped under the inferior mesenteric artery and thus does not reach its normal site. Rarely, the kidney fuses to the contralateral one and ascends to the opposite side, resulting in a cross-fused ectopy.
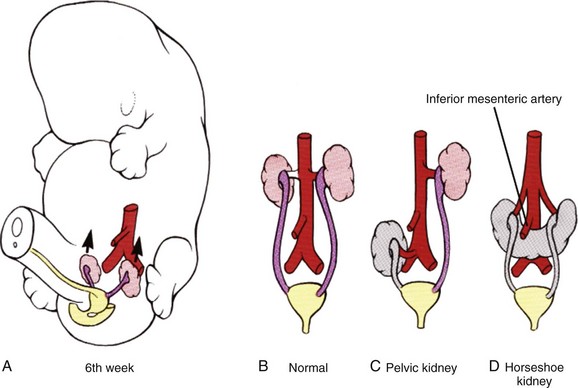
(Modified from Larsen WJ. Human embryology. New York: Churchill Livingstone; 1997.)
Molecular Mechanism of Kidney Development
The details of inductive interactions among metanephric mesenchyme, ureteric bud epithelia, and, more recently, the stroma, are becoming clearer and provide insights into the complex regulatory mechanisms underlying renal development. Formation of renal tubules and collecting system occurs sequentially and requires dynamic interactions among epithelial, mesenchymal, and stromal cells. Many of the early events in embryonic kidney development were first elucidated by manipulating lower vertebrate embryos and by utilizing a mammalian in-vitro organ culture system. Clifford Grobstein’s pioneering work in the 1950s led to an organ culture technique (Grobstein, 1956) whereby the metanephric mesenchyme is separated from the ureteric bud during the early part of kidney development and grown in vitro on a filter. An inducer tissue, such as ureter or spinal cord, cultured on the opposite side of the filter then provides the inductive signal (Fig. 111–11). This ingenious experimental approach has established the kidney as a model system for studying the role of epithelial-mesenchymal interaction in organ development. The development of many other organs, including lung, salivary glands, gonads, prostate, and bladder, also require epithelial-mesenchymal interaction for the controlled differentiation and proliferation of tissues.
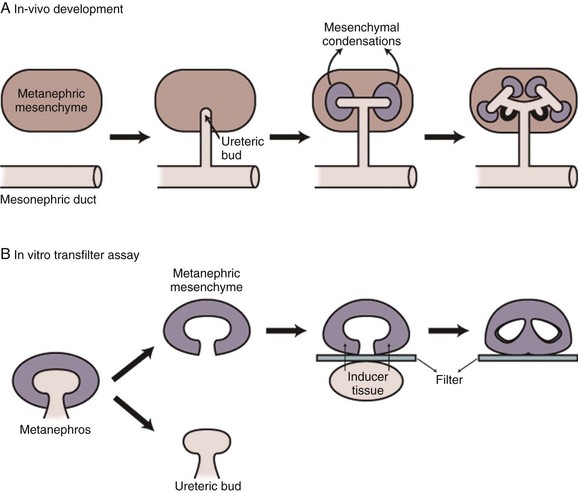
(Modified from Vainio S, Muller U. Inductive tissue interactions, cell signaling, and the control of kidney organogenesis. Cell 1997;90[6]:975.)
Formation of Nephric Ducts
The first recognizable event in renal development may be the formation of nephric ducts within the region of the intermediate mesoderm. The molecular signals responsible for this early event, in which seemingly unorganized mesenchymal cells aggregate to become an epithelial duct, remain essentially unknown, but details are beginning to emerge. The early intermediate mesoderm destined to become nephric ducts is distinguished by expression of the transcription factors LIM1, PAX2, and SIM1, but only LIM1 appears to be absolutely essential for nephric duct formation (Shawlot and Behringer, 1995). PAX2 may be important for maintaining other marker gene expression in the nephric ducts (Torres et al, 1995). Available data suggest a model in which few opposing secreted factors from the surrounding tissues cumulatively restrict LIM1 expression to the intermediate mesoderm. LIM1 then activates PAX2 expression to further orchestrate the formation of nephric ducts.
Ureteric Bud Outgrowth toward Metanephric Mesenchyme
The outgrowth of the ureteric bud from the nephric duct and its invasion into the condensing blastema of metanephric mesenchyme is a crucial initiating event in the development of the adult kidney (metanephros). Many candidate genes have been identified to play a critical role in this process (see http://golgi.ana.ed.ac.uk/kidhome.html). In particular, several lines of experimental evidence have revealed a crucial role of the RET-GDNF-GFRα1 pathway in the ureteric bud outgrowth (Fig. 111–12). Glial cell line–derived neurotrophic factor (GDNF) is a secreted peptide expressed in the metanephric mesenchyme that activates the RET receptor, which is expressed along the nephric duct. GDNF activation of RET requires the glycosylphosphatidylinositol (GPI)-linked protein GFRα1, which is expressed in both metanephric mesenchyme and nephric duct. Gene knockout mutations in Ret, GDNF (Moore et al, 1996; Pichel et al, 1996; Sanchez et al, 1996), and GFRα1 (Cacalano et al, 1998) inhibit ureteric bud outgrowth. In organ culture systems, recombinant GDNF is sufficient to induce ectopic ureteric bud outgrowth (Sainio et al, 1997) (Fig. 111–13). However, the competence of the nephric duct to respond to GDNF is restricted along the anteroposterior axis. This anteroposterior restriction might be mediated by suppressors of RET signaling within the surrounding tissue, such as bone morphogenetic protein-4 (BMP4). Mice that are deficient for BMP4 show more broadened ureteric buds and/or secondary anterior buds, suggesting that full BMP4 activity is required to limit RET signaling to the caudal aspect adjacent to the developing metanephric mesenchyme (Miyazaki et al, 2000). Similarly, in organ culture, BMP4 can suppress the activity of GDNF to induce ectopic ureteric bud formation (Brophy et al, 2001). Proper positioning of the ureteric bud is also controlled by the localized expression of GDNF within the metanephric mesenchyme. Both positive and negative regulators have been described for GDNF localization. Homozygous mutation of a transcription factor Eya1 causes failure of ureteric bud outgrowth, and its metanephric mesenchyme lacks GDNF expression, suggesting that Eya1 regulates GDNF expression (Xu et al, 1999). In humans, haploinsufficiency of Eya1 results in a dominantly inherited disorder called branchio-oto-renal syndrome, which involves kidney and urinary tract anomalies (Abdelhak et al, 1997). Expression of PAX2 in the metanephric mesenchyme is also required for GDNF activation (Brophy et al, 2001). GDNF expression is also suppressed at the anterior boundary of the metanephric mesenchyme through the concerted action of the FoxC1 and FoxC2 transcription factors (Kume et al, 2000). Mutations in either Fox gene result in an expansion of GDNF expression and the formation of ectopic ureteric buds. Most FoxC1 homozygous mutants have duplex kidneys, in which the upper ureter is dilated and connects aberrantly to ectopic nephric duct derivatives in males such as seminal vesicles and vas deferens. In the developing kidneys, Slit2 is primarily expressed in the nephric duct whereas Robo2 is expressed in the metanephric mesenchyme (Piper et al, 2000). Mice deficient in Slit2 or Robo2 exhibit ectopic ureteric bud formation, multiple ureters and hydroureter, and anterior expansion of GDNF expression (Grieshammer et al, 2004). SPRY1 negatively regulates GDNF-RET signaling. Loss of SPRY1 function in mice results in renal malformations, including multiple ureters, duplex kidneys and hydroureter, and increased expression of GDNF in the metanephric mesenchyme (Basson et al, 2006). The data therefore suggest that multiple factors regulate, both positively and negatively, the precise timing and localization of GDNF, which then functions as a guidance cue to activate RET.
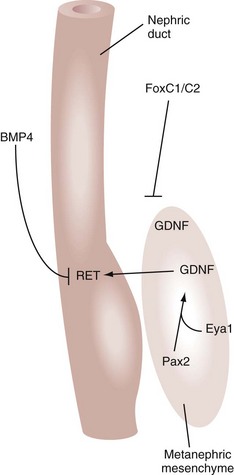
(Modified from Dressler GR. Tubulogenesis in the developing mammalian kidney. Trends Cell Biol 2002;12:390–5.)
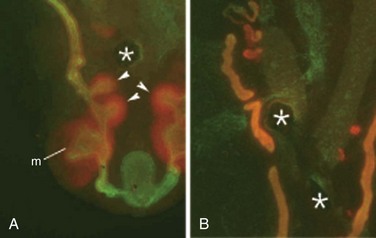
(From Dressler GR. Tubulogenesis in the developing mammalian kidney. Trends Cell Biol 2002;12:390–5.)
Ureteric Bud Branching
Once the ureteric bud has contacted the condensing metanephric mesenchyme it undergoes a dichotomous branching morphogenesis (Cebrian et al, 2004). Many of the same factors that regulate the initial outgrowth of the ureteric bud also appear to be essential for the subsequent branching of the ureteric bud. Ureteric bud branching is positively regulated by genetic and nutritional factors. PAX2, a paired-box type transcription factor that is mutated in humans with renal coloboma syndrome, is a positive regulator of ureteric bud branching. During renal development, Pax2 is expressed in the nephric duct, ureteric bud, and metanephric blastema induced by ureteric bud branch tips. Mice with Pax2 mutation exhibit decreased ureteric bud branching and renal hypoplasia (Porteous et al, 2000). Ureteric branching is also positively regulated by vitamin A and its retinoic acid receptor signaling, which promote Ret expression. Rarα and Rarβ2 are expressed in stromal cells surrounding Ret-expressing ureteric bud branch tips. Mice deficient in these receptors exhibit a decreased number of ureteric bud branches and diminished expression of Ret (Batourina et al, 2001). Certain markers such as Wnt11 might already be compartmentalized to opposing poles of the dilated bud tips, even before a morphologic branch point is evident (Pepicelli et al, 1997). In mice deficient for the homeobox gene Emx2 (Miyamoto et al, 1997), ureteric bud outgrowth into the metanephric mesenchyme appears normal but the leading edge never dilates and branching is suppressed. Thus ureteric development is arrested before the first branching event, and the resulting metanephric mesenchyme does not express any markers for induction. Similarly, mice with mutation of Sall1 exhibit developmental arrest just after ureteric bud outgrowth and before dilation of the leading edge (Nishinakamura et al, 2001). In normal murine embryos, Sall1 is expressed in the metanephric mesenchyme. Thus Sall1 might control mesenchyme-derived signals that are necessary for ureteric bud dilation and the early branch point determination. Clearly, the pattern of ureteric bud branching and the expression of ureteric bud–specific genes are influenced by the metanephric mesenchyme. Indeed, the heterologous mesenchyme derived from lung primordia can not only change the pattern of ureteric bud branching to that of lung epithelia but also induce the ureteric bud tissues to express lung-specific genes (Lin et al, 2001). Recent studies demonstrate that BMP/activin-like kinase-2 (ALK3) signaling negatively regulates early ureteric bud branching in vivo (Hartwig et al, 2008). The cell surface receptor ALK3 binds BMP2 and BMP4 with high affinity and is expressed in the nephric duct. Inactivation of ALK3 changes the pattern of primary ureteric bud branching from bifid to trifid and increases the number of first- and second-generation branches. These defects are associated with decreased formation of subsequent branch generations, resulting in a decreased complement of collecting ducts. These observations suggest that the pattern of early ureteric bud branching is a critical determinant of subsequent branching morphogenesis. Thus ureteric bud epithelial branching morphogenesis is controlled by both intrinsic and extrinsic factors working in concert to generate a kidney-specific branching pattern.
Tubulogenesis
Classic tissue recombination experiments focused almost exclusively on the relationship between metanephric mesenchymal cells and ureteric bud epithelial cells. It is now clear that at least three cell types are involved in the control of renal development: the ureteric bud tip cells, the condensed mesenchymal cells, and the stromal or interstitial mesenchymal cells (Fig. 111–14). It is not known whether the mesenchyme is a homogeneous cell population before its interaction with the ureteric bud. It is clear, however, that once induced by the ureteric bud the metanephric mesenchyme patterns itself into at least two different cell populations, a tubular one and a stromal one. The tubular cell population is thought to derive from mesenchymal cells in direct contact with the ureteric bud ampulla (Vainio et al, 1989; Stark et al, 1994; Torres et al, 1995), whereas the stromal cell population surrounds the tubular cells (Hatini et al, 1996). Once the mesenchyme has been patterned, the cells in the tubular zone undergo morphogenesis to become renal tubular epithelial cells. There is evidence that this process is dependent not only on signals from the ureteric bud but also on signals from the mesenchyme itself. One of these autocrine signals may be Wnt4, whose expression is induced in cells of the tubular zone on interaction with the ureteric bud. In Wnt4 gene knockout mice the ureteric bud forms and invades the metanephric mesenchyme but subsequent development of epithelial tubules is abolished (Stark et al, 1994). This suggests that two signals are essential for renal tubule formation—initial ureteric bud derived signals activating Wnt4 expression in the metanephric mesenchyme and Wnt4 itself as a mesenchymal autocrine signal. Signals from the stromal cell population also contribute to tubule formation as well, because tubulogenesis is perturbed in Bf2 gene knockout mice (Hatini et al, 1996). The discovery that Wnt4 acts as a downstream signal during the induction cascade leading to renal tubulogenesis leads to the question regarding the nature of the initial ureteric bud–derived signals. In-vitro data suggest a role for fibroblast growth factor-2 (FGF2) and other uncharacterized factors secreted by the ureteric bud (Karavanova et al, 1996). Candidate molecules that may cooperate with FGF2 are Wnt11 and BMP7 (Kispert et al, 1996; Vukicevic et al, 1996). Localization of RET protein to the ureteric bud tips is reinforced by both GDNF (Pepicelli et al, 1997) and signals emanating from surrounding stromal cells. For example, retinoic acid receptors are expressed in the stromal cells and are required for stromal cell–mediated signaling to maintain high levels of RET expression in the bud tips (Mendelsohn et al, 1999; Batourina et al, 2001). Consistent with the role of retinoic acid receptors in maintaining RET expression in the dividing ureteric bud, rats suffering from vitamin A deficiency have smaller kidneys and fewer nephrons (Lelievre-Pegorier et al, 1999). The cellular crosstalk among stromal, mesenchymal, and ureteric bud cells is further highlighted by gain- and loss-of-function experiments involving FGFs and BMPs. Fgf7 null mutant mice have fewer branch points and correspondingly fewer nephrons, whereas ectopic FGF7 in organ culture can stimulate branching (Qiao et al, 1999). FGF1 and FGF10 affect elongation of the ureteric bud stalk before the branch-point decision is made (Qiao et al, 2001). Null mutations in Bmp7 are associated with even more severe phenotypic anomalies, exhibiting limited branching morphogenesis and complete renal developmental arrest. Yet it is difficult to assess how FGFs and BMPs exert their collective effects on branching given the interplay among all the cell types present in the early kidney (Dudley et al, 1999). In addition to the proteins just mentioned, a growing list of growth factors, secreted peptides, and their receptors have been implicated in the control of branching morphogenesis, most by using a variety of in-vitro model systems (Pohl et al, 2000; Davies, 2001). For many of these factors, however, genetic studies have not proved conclusive in assigning specific functional roles during ureteric bud branching in vivo, either because of potential redundancies or embryonic lethalities before the onset of kidney development. Nevertheless, the role of these factors in the renal development must be considered.
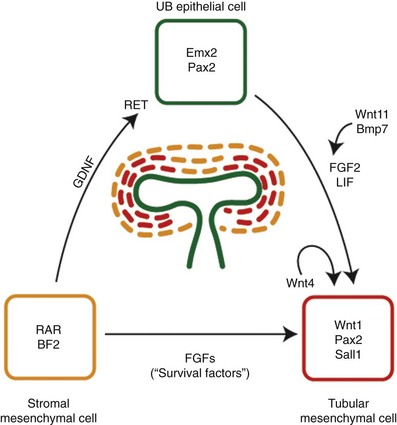
(Modified from Dressler GR. Tubulogenesis in the developing mammalian kidney. Trends Cell Biol 2002;12:390–5.)
Mesenchymal-Epithelial Conversion
The inductive signals emanating from the ureteric bud promote condensation of the metanephric mesenchymal cells around the ureteric bud tips and subsequent tubulogenesis. Mice with null mutations of Pax2 or Wt1 fail to exhibit ureteric bud outgrowth and, in both cases, the metanephric mesenchyme does not respond to induction even when recombined with strong inducers in vitro (Kreidberg et al, 1993; Brophy et al, 2001). The establishment of glomerular versus tubular cell fates is dependent on negative feedback between Wt1 and Pax2 (Ryan et al, 1995). During early kidney development, the expression domain of Pax2 is complementary to that of Wt1 in S-shaped bodies. Wt1 expression is restricted to glomerular epithelial precursors (Pelletier et al, 1991), whereas Pax2 expression is restricted to the portion that gives rise to tubular epithelial precursors of the proximal and distal nephron segments and later repressed in differentiated tubular epithelium (Dressler and Douglass, 1992). Evidence in support of Wnt proteins as mesenchyme inducers has been gained from in-vitro induction assays using Wnt-expressing cell lines (Herzlinger et al, 1994; Kispert et al, 1998). Of the Wnt mutants examined to date, only Wnt4, which is expressed in the mesenchyme and not the ureteric bud, is crucial for propagation of the inductive signals. Although Wnt4 mutant mesenchyme is able to aggregate in response to ureteric bud contact, these mutant aggregates do not form polarized epithelia. Rat ureteric bud cells secrete tubulogenic factors, such as leukocyte-inhibitory factor (LIF), which, together with FGF2, appears to stimulate growth and tubulogenesis in vitro (Plisov et al, 2001). Once induced to form aggregates, metanephric mesenchyme becomes polarized into an early renal vesicle. This vesicle is closely associated with the branching ureteric bud and will eventually connect to the ureteric bud epithelium to form a continuous tubule. Profound changes take place in the expression of cell adhesion molecules such as cadherins. Shortly after induction, metanephric mesenchyme expresses R-cadherin, cadherin-6, and E-cadherin, along with suppression of the mesenchyme-specific cadherin-11. Both R-cadherin and cadherin-6 mutants show defects in the rate of mesenchymal condensation and polarization (Mah et al, 2000; Dahl et al, 2002). Some renal vesicles in cadherin-6 mutants also fail to fuse to the ureteric bud epithelia, resulting in “dead end” tubules and a subsequent loss of nephrons.
Renal Vascular Development
The origin of intrarenal vasculature is not completely understood. Until recently it was thought that renal vasculature derived exclusively from branches off the aorta and other pre-existing extrarenal vessels (“angiogenic” hypothesis). There is evidence, however, that the renal vessels may originate in situ, within the embryonic kidney from vascular progenitor cells (“vasculogenic” hypothesis) (Loughna et al, 1996; Tufro et al, 1999). Using antibodies to Flk-1, a vascular endothelial growth factor (VEGF) receptor present in angioblasts and mature endothelial cells, it was demonstrated that endothelial cell precursors were already present in the prevascular rodent kidneys before any vessels were discernible from a morphologic standpoint. When embryonic kidneys are cultured at the usual atmospheric oxygen concentration, vessels do not develop. However, if the explants are cultured in a hypoxic atmosphere containing 5% oxygen, capillary sprouts develop within and outside the glomeruli, an effect that is inhibited by anti-VEGF antibodies (Tufro-McReddie et al, 1997). Depending on the developmental potential of the cells involved, both angiogenesis and vasculogenesis may play a role in the development of renal vasculature (Abrahamson et al, 1998).
Bladder and Ureter Development
Formation of Urogenital Sinus
At the third week of gestation the cloacal membrane remains a bilaminar structure composed of endoderm and ectoderm. During the fourth week the neural tube and the tail of the embryo grow dorsally and caudally, projecting itself over the cloacal membrane; and this differential growth of the body results in embryo folding. The cloacal membrane is now turned to the ventral aspect of the embryo, and the terminal portion of the endoderm-lined yolk sac dilates and becomes the cloaca (Fig. 111–15). According to the embryonic theories of Rathke and Tourneux, the partition of the cloaca into an anterior urogenital sinus and a posterior anorectal canal occurs by the midline fusion of two lateral ridges of the cloacal wall and by a descending urorectal septum. This process is thought to occur during the fifth and sixth weeks, and it is culminated by the fusion of this urorectal septum with the cloacal membrane. Recently, however, some investigators have challenged this classic view with evidence that there is neither a descending septum nor fusing lateral ridges of the cloacal wall (van der Putte, 1986; Kluth et al, 1995). There is further evidence that the urorectal septum never fuses with the cloacal membrane (Nievelstein et al, 1998). According to these observations the congenital cloacal and anorectal malformations, which were previously thought to occur due to a failure of septum formation and its fusion with the cloacal membrane, may in fact occur from an abnormal development of the cloacal membrane itself (Nievelstein et al, 1998) (Fig. 111–16).
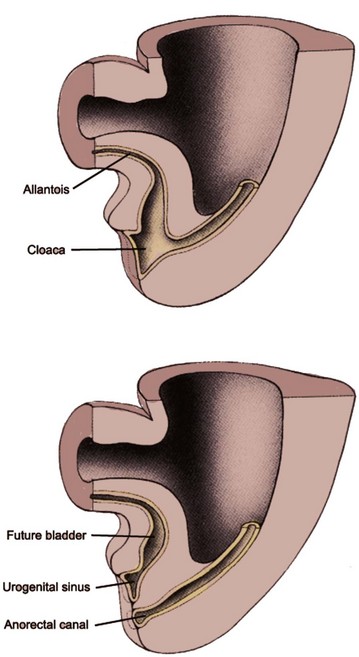
(Modified from Larsen WJ. Human embryology. New York: Churchill Livingstone; 1997.)
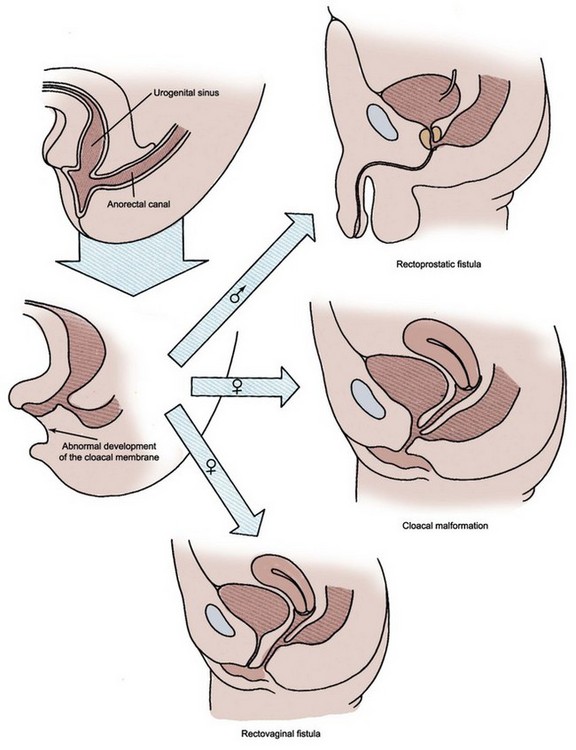
(Modified from Larsen WJ. Human embryology. New York: Churchill Livingstone; 1997.)
Formation of Trigone
By day 33 of gestation, the common excretory ducts (the portion of nephric ducts distal to the origin of ureteric buds) dilate and connect to the urogenital sinus. The formation of these final connections involves apoptosis, which enables the ureters to disconnect from the nephric ducts, and fusion, in which the ureteral orifice inserts into the urogenital sinus epithelium at the level of the trigone (Batourina et al, 2005). According to the classic view (Weiss 1988), the right and left common excretory ducts fuse in the midline as a triangular area, forming the primitive trigone, structurally different from bladder and urethra. The ureteral orifice extrophies and evaginates into the bladder by day 37 and begins to migrate in a cranial and lateral direction within the floor of the bladder. During this process the nephric duct orifice diverges away from the ureteral orifice and migrates caudally, flanking the paramesonephric (müllerian) duct at the level of the urogenital sinus. This is the site of the future verumontanum in males and vaginal canal in females. Recent studies, however, have challenged this classic mechanism of trigone development. Using the cell lineage studies in mice, the trigone was found to form mostly from bladder smooth muscle cells with only a minor contribution from the ureters (Viana et al, 2007).
The embryonic pattern of ureteral orifice incorporation into the developing bladder is inferred primarily from clinical observations of duplex kidneys. The upper pole ureteral orifice rotates posteriorly relative to the lower pole orifice and assumes a more caudal and medial position. Weigert and Meyer recognized the regularity of this relationship between upper and lower pole ureteral orifices, which has come to be known as the Weigert-Meyer rule. According to this concept, an abnormally lateral lower pole ureteral orifice may result from a ureteric bud arising too low on the nephric duct, therefore resulting in premature incorporation and migration within the developing bladder. In such a ureteral orifice, vesicoureteral reflux is more likely to occur due to an inadequate intramural tunnel. In contrast, an abnormally caudal upper pole ureteral orifice may result from a ureteric bud arising too high on the nephric duct. It may drain at the bladder neck and verumontanum or remain connected to the nephric (wolffian) duct derivatives such as the vas deferens in males (Mackie and Stephens, 1977; Schwarz and Stephens, 1978). In females, the ectopic upper pole ureter may insert into the remnants of the nephric ducts (e.g., Gartner duct cyst) or vaginal vestibule (Fig. 111–17).
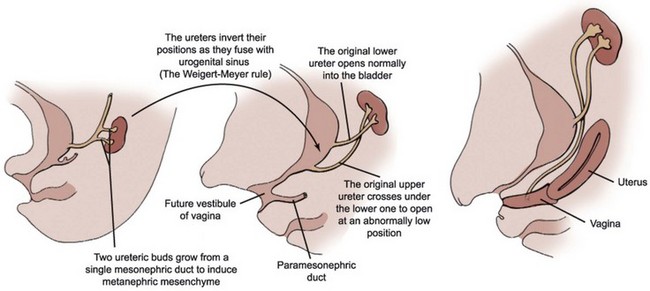
Figure 111–17 Development of an ectopic upper pole ureter draining into the vagina.
(Modified from Larsen WJ. Human embryology. New York: Churchill Livingstone; 1997.)