One of the first observations of a potential neural control of kidney function was made by Claude Bernard in the middle of the 19th century, who reported a unilateral diuresis following section of the greater splanchnic nerve of the anesthetized dog. However, the critical role of the sympathetic nervous system in the control of renal function was long questioned due to the views of Homer Smith, who regarded the renal innervation to be of little importance in determining the function of the kidney. It was not until the 1960s that the innervation of the kidney and its regulation of renal function came under detailed scrutiny. Over the following three-to-four decades a wealth of information has been generated and concepts developed which have provided the foundation for how the renal sympathetic nerves regulate all aspects of vascular, tubular, and secretory functions of the kidney, both normally and in pathophysiological states of cardiovascular diseases. A number of major reviews have brought together the most significant pieces of information about the renal sympathetic nerves over this period. This knowledge is now being translated into therapeutic approaches in man, particularly in relation to hypertension, heart failure, and renal disease. The recent reports by Esler and co-workers that bilateral renal denervation in patients with resistant hypertension resulted in a profound and sustained (≥2 years) reduction in blood pressure reinforces the important contribution of the autonomic control of the kidney in determining cardiovascular homeostasis and how it may be involved in pathophysiological states. These findings are set to reinforce the drive to gain knowledge of both the efferent and afferent innervations of the kidney, and to stimulate the development of further therapeutic avenues to modulate the neural control of the kidney.
Renal Sympathetic Nerves
Introduction
One of the first observations of a potential neural control of kidney function was made by Claude Bernard in the middle of the 19th century, who reported a unilateral diuresis following section of the greater splanchnic nerve of the anesthetized dog. However, the critical role of the sympathetic nervous system in the control of renal function was long questioned due to the views of Homer Smith, who regarded the renal innervation to be of little importance in determining the function of the kidney. It was not until the 1960s that the innervation of the kidney and its regulation of renal function came under detailed scrutiny. Over the following three-to-four decades a wealth of information has been generated and concepts developed which have provided the foundation for how the renal sympathetic nerves regulate all aspects of vascular, tubular, and secretory functions of the kidney, both normally and in pathophysiological states of cardiovascular diseases. A number of major reviews have brought together the most significant pieces of information about the renal sympathetic nerves over this period. This knowledge is now being translated into therapeutic approaches in man, particularly in relation to hypertension, heart failure, and renal disease. The recent reports by Esler and co-workers that bilateral renal denervation in patients with resistant hypertension resulted in a profound and sustained (≥2 years) reduction in blood pressure reinforces the important contribution of the autonomic control of the kidney in determining cardiovascular homeostasis and how it may be involved in pathophysiological states. These findings are set to reinforce the drive to gain knowledge of both the efferent and afferent innervations of the kidney, and to stimulate the development of further therapeutic avenues to modulate the neural control of the kidney.
Neuroanatomy, Pharmacology, and Physiology
Extrinsic Innervation
The efferent innervation of the kidney comprises pre-ganglionic fibers which arise from spinal segments T 11 –L 3 , and traverse to both pre-vertebral (thoraco-lumbar sympathetic chain) and para-vertebral ganglia (aortico-renal, splanchnic, coeliac, superior mesenteric ganglia) which give rise to the post-ganglionic fibers. There is great species variation in the overall contribution from the various ganglia, for example, with 80% arising from the ipsilateral para-vertebral ganglia (T 11 –L 2 ) in the rat and hamster, but only 50% for the cat and monkey (T 11 –L 3 ). Figure 16.1 gives a brief outline of these different neural pathways. Tracer studies with horseradish peroxidase and herpes virus have been used to demonstrate the neural pathways for the sympathetic innervation. The pre-ganglionic sympathetic nerve fibers in the rat are primarily located in the intermediolateral column of the spinal cord, from T 9 –T 13 , suggesting that they descend three to four segments before exiting the spinal column at T 11 –L 3 . A number of nuclei within the central nervous system project to these intermediolateral areas of the spinal cord, including the raphe nuclei, rostral venterolateral medulla, an A5 group, and the paraventricular hypothalamic nucleus. Together, there is a view that descending projections from the supra-spinal systems are the primary regulators of sympathetic outflow to the kidney.

There has been a continuing evaluation of the size and type of nerves forming the efferent innervation of the kidney. Analyses performed in the rabbit utilizing electron microscopy have demonstrated two types of nerve fibers with different diameters in the renal cortex, suggesting that different functionalities may exist within these different nerve populations. Detailed counts of fiber number and diameter in the rat have found that the majority of the fibers are unmylelinated (96%) with the remainder being myelinated. The average diameter of the unmyelinated fibers was approximately 1.3 μm, and while DiBona and colleagues reported a bimodal distribution, this was not observed in the report of Sato and co-workers. More recently, Fazan and colleagues investigated the situation in the mouse and found that, although the nerve fibers had a similar mean diameter, 0.76±0.02 μm, compared to the rat, the distribution was clearly uni-modal. Together, these observations provide no consistent support for the argument that different nerve fibers are directed towards the innervation of specific cell types (vascular, epithelial or granular) in order to control selected functions.
Intrinsic Innervation
The sympathetic nerves generally traverse from the ganglia, running alongside the renal artery, and enter the hilus of the kidney where they begin to divide, with smaller nerve bundles approximately following the major divisions of the blood vessels. The sympathetic nerves begin to divide into smaller bundles which penetrate and form a network throughout the cortical and juxta-medullary areas. Early studies by Barajas and co-workers demonstrated discrete neuro-effector junctions present at the afferent and efferent arterioles, the granular cells of the juxtaglomerular apparatus, the proximal and distal tubules, and the thick limb of the ascending limb of the loop of Henle. This group of investigators, utilizing electron microscopy, went on to show that the sympathetic nerves were typical autonomic fibers having varacosities associated with the neuro-effector junctions that contained dense cored vesicles. Using a tritiated noradrenaline radiographic approach they clearly showed the presence of noradrenaline, and although there was a suggestion that acetylcholine esterase was present, there was no indication that the fibers were cholinergic. Later, more detailed studies indicated that there was a variation in the number of neuro-effector junctions along the nephron, with the greatest number being at the proximal tubule, fewer at the thick ascending limb of the loop of Henle, and the smallest number at the distal tubules and collecting duct. Interestingly, when calculated as the density of neuro-effector junctions per unit length, the density was found to be greatest in the thick ascending limb of the loop of Henle and progressively less in the distal and proximal tubules. There is also a regional variation, with the innervation being greatest along the cortico-medullary border and becoming less in the outer cortex and deeper regions of the medulla. This regional variation in innervation density is paralleled in both the vascular and tubular structures. Figure 16.2 illustrates the cell types where neuro-effector junctions have been described and the functions they may regulate.

Pharmacology
Neurotransmitters
A large body of evidence from biochemical and pharmacological studies indicates that the primary neurotransmitter arising from the sympathetic innervation is noradrenaline. Functional studies have shown that renal denervation, in the initial stages, is associated with a marked decrease in noradrenaline content of some 95%, while activation of the renal sympathetic nerves results in an elevation in noradrenaline production or spillover into the renal venous blood. The role of dopamine and potential dopaminergic nerve-mediated influences remains unclear, as all adrenergic nerve varicosities contain dopamine as an intermediary in noradrenaline biosynthesis, but there is little evidence that dopamine acts as a neurotransmitter in the neural regulation of either renal hemodynamic function, renin release or fluid reabsorption.
Adrenoceptors
Multiple types of adrenoceptors are present within the kidney mediating the actions of noradrenaline released from the nerve varicosities, and a range of α 1 – and α 2 -adrenoceptors subtypes exist along the renal vasculature and nephrons. At a functional level, stimulation of the α-adrenoceptors causes a vasoconstriction of vascular smooth muscle and reabsorption of fluid at tubular epithelial cells. Stimulation of β-adrenoceptors increases renin release at the juxtaglomerular granular cells. A number of molecular biological and cloning investigations have defined α 1 -adrenoceptors into α 1A -, α 1B -, and α 1D -subtypes, α 2 -adrenoceptors into α 2A -, α 2B -, and α 2C -subtypes, and β-adrenoceptors as β 1 -, β 2 -, and β 3 -subtypes, all of which are G-protein coupled receptors comprising a superfamily of membrane proteins that signal the actions of adrenaline and noradrenaline. The α 1 -adrenoceptors utilize a range of signaling pathways including activation of phospholipases A and C, mobilization of intracellular calcium stores, as well as opening of voltage-dependent and independent calcium channels which results in a rapid vascular smooth muscle contraction. Interestingly, noradrenaline binding to α 1 -adrenoceptors also activates the MAP kinase pathways which, in the longer term, are responsible for regulating growth and hypertrophy of the vascular smooth muscle. There are reports that α 1A – and α 1B -adrenoceptors are present to a similar degree in the cortex and outer stripe of the medulla, while in the inner stripe of the medulla the α 1B -adrenoceptor subtype appears to predominate. However, in terms of hemodynamic functionality, α 1A -adrenoceptors are more effective than the α 1B -adrenoceptor subtypes in causing renal vasoconstriction.
α-Adrenoceptors are also present on the epithelial cells of the nephron, where they modulate fluid reabsorption. There is evidence, at least in the proximal tubule, that α 1 -adrenoceptor stimulation engages both the phospholipase C and MAP kinase pathways, but these signal to different end-points, that is to the sodium/hydrogen exchanger, isoforms-1 (NHE1) and isoform-3 (NHE3), respectively. At the more distal sections of the nephron, the distal tubule and collecting duct, α 2 -adrenoceptors are the primary subtype, and in this segment their activation results in a decrease in cAMP which blunts the action of other factors signaling through this pathway. A key example is AVP, which stimulates both water reabsorption and ENaC insertion in this region where these actions are blunted by α 2 -adrenoceptor agonists. β 1 -adrenoceptors are found primarily on the granular renin-containing cells of the afferent arteriole, and their activation stimulates adenylyl cyclase which increases intracellular levels of cAMP. The β 2 -adrenoceptors found on the tubules, primarily collecting duct, also utilize cAMP production as the signaling molecule, but their function at this site has not been resolved.
Physiology
Activation of the various signaling pathways via ligand binding to the adrenoceptors increases fluid reabsorption at the level of the proximal tubule. In the proximal tubule, the catecholamines stimulate Na/K-ATPase at the basolateral membrane, resulting in increased sodium reabsorption from the tubular lumen across the apical membrane. There is evidence from micropuncture studies, as well as in vitro studies, that the sodium–hydrogen exchanger is activated, particularly isoform 3 (NHE3), the isoform primarily responsible for the regulation of sodium entry into the epithelial cells at the proximal tubule. The NHE3 protein in the proximal tubule appears to be present in the microvillae, either incorporated into the plasma membrane where it is likely to be active or internally in the subapical vesicles, where it is considered to be inactive in terms of a transporting protein. The mechanisms involved in the translocation of the NHE3 from the plasma membrane into the subapical vesicles are complex and require the interaction of a number of proteins (NHERF1, myosin VI, ezrin, raft, non-raft, and myosin). McDonough has recently reviewed the evidence showing that when blood pressure is elevated acutely or when the sympathetic nervous system is reflexly activated there is increased movement of NHE3 into the subapical compartment where the transporter is inactive. McDonough and co-workers have put forward the concept that translocation of the NHE3 into the subapical regions is a key element whereby sodium reabsorption may be regulated by catecholamines, both in the short-term as well as over a longer timeframe, thereby contributing to cardiovascular homeostasis.
Autocrine and Paracrine Influences on Neurotransmission
The interstitium of the kidney contains a complex milieu of hormones and factors which may vary across the cortex and medulla, but can determine the level of functions of all cell types, vascular smooth muscle, renin containing, and epithelial cells. There are modulator influences at the neuro-effector junction which can come into play and influence the amount of noradrenaline released in response to depolarization caused by the passage of an action potential. An outline of potential interactions with various agents is illustrated in Figure 16.3 .

Adrenoceptors
At an early stage it was recognized that pre-synaptic α 2 -adrenoceptors were able to act in an auto-inhibitory fashion, whereby when activated by noradenaline released into the neuro-effector junction, they decreased neurotransmitter release caused by subsequent depolarizations. There is evidence that this situation pertains at the kidney. α 2 -adrenoceptors are present in the kidney, and their blockade enhances noradrenaline release and renal nerve induced vasoconstriction in the dog. In the rabbit blockade of α 2 -adrenoceptors peripherally has little effect on renal nerve-induced vasoconstrictions, but potentiates the renal nerve-mediated antidiuresis and antinatriuresis.
Angiotensin II
A second important neuro-modulator is angiotensin II, which is present in the renal interstitium at high levels in conditions of increased endogenous angiotensin II production. Activation of pre-synaptic AT-1 receptors facilitates the release of noradrenaline. This was first reported by Boke and Malik using the isolated perfused rat kidney. They found that renal nerve-induced catacholamine release into the renal vein was facilitated when angiotensin II was added to the perfusate. At a functional level, studies in the anaesthetized rat using both direct and reflex activation of the renal nerves, at levels which had little or no effect on renal hemodynamics, caused decreases in fluid excretion which were blocked in the presence of an angiotensin-converting enzyme inhibitor, and restored following administration of exogenous angiotensin II. Similar observations were reported by Veelken and colleagues in the conscious rat, where administration of the AT1-receptor antagonist ZD 7155 blunted the renal nerve-dependent antidiuresis and antinatriuresis in response to an air-jet stress test. Together, these findings support the view that at both renal vascular and epithelial cell neuro-effector junctions occupation of pre-synaptic AT1 receptors enhances neurotransmission. It would seem that these receptors are fully occupied, even at low endogenous levels of angiotensin II and under normal conditions the facilitation is maximal but when production is prevented, then neurotransmission will be blunted. There may be other interactions at the post-synaptic membranes of the epithelial cells. Quan and colleagues demonstrated that the ability of angiotensin II to stimulate proximal tubule fluid reabsorption was blunted following acute renal denervation, but augmented if the renal sympathetic nerves were stimulated at low levels not affecting filtration rate. A comparable interaction was reported by Abdulla and co-workers, who found that the renal vasoconstriction caused by close renal arterial infusion of angiotensin II was blunted following acute renal denervation. Thus, these observations demonstrate that angiotensin II has modulatory activities at the neuro-effector junction, both pre-synaptically and post-synaptically, which determine the effectiveness of the neural control of renin release, fluid reabsorption, and vascular tone in the kidney.
Nitric Oxide
A third potentially important factor determining noradrenaline release, and hence its impact on functional end-points, is nitric oxide (NO). All isoforms of nitric oxide synthase (NOS), endothelial (eNOS), neuronal (nNOS), and inducible (iNOS) are present in the kidney. eNOS has been found along the vasculature and glomerular capillaries, nNOS is present in the renal nerves, at low concentrations along the tubules and high concentrations in the macula densa, while iNOS is expressed constitutively in the medulla. It is possible that in the environment of the neuro-effector junction, NO may have both pre-synaptic and post-synaptic actions, and this probably contributes to the conflicting findings which have been reported. Thus, in the anaesthetized dog, the low level renal nerve stimulated noradrenaline output was enhanced following NOS inhibition and suppressed in the presence of an NO donor, consistent with an inhibitory pre-synaptic action. In contrast in the rat, NOS blockade suppressed renal nerve mediated noradrenaline output and neurally induced renal vasoconstriction. In terms of the influence of NO on renal nerve stimulated increases in sodium reabsorption, there is a lack of consistency. It is evident that NOS inhibition increases, and exogenous NO decreases, proximal tubule fluid reabsorption, suggesting an inhibitory action of NO on reabsorptive processes. Importantly, this action of NO is only evident if the renal nerves are intact. In an apparent conflict with these studies are the findings that renal nerve-induced increases in fluid reabsorption are prevented by NOS blockade, consistent with a facilitating action of NO. In attempting to clarify these differing reports, it is possible that NO could act in two ways, directly within the post-synaptic cell, either vasculature or epithelial cells, where it may blunt the neurally controlled cell function, or indirectly by facilitating neurotransmitter release. The differing balances between these two sites of action may simply reflect how the NO generating systems may be activated by the various experimental conditions of the investigations.
Reactive Oxygen Species
The generation of cellular energy results in the production of reactive oxygen species which comprise radicals that can damage both nuclear and cytoplasmic proteins and phospholipids. A raised output of reactive oxygen species is recognized as a state of oxidative stress that is associated with a range of metabolic and cardiovascular diseases. Reactive oxygen species comprise superoxide anions, H 2 O 2 , and other reactive radicals which are able to influence both neurotransmission and the responsiveness of the target cells to the neurotransmitter. The actions of the reactive oxygen species may be either direct or indirect, as a consequence of their ability to reduce the bioavailability of NO. There are reports that systemic infusion of tempol, a synthetic diffusible superoxide dismutase mimetic which scavenges superoxide anions, reduced blood pressure and renal sympathetic nerve activity to the same extent before and following NOS blockade. These findings would be compatible with the argument that in states of oxidative stress, for example hypertension, increased production of superoxide anions could enhance the activity of the sympathetic nervous system, and hence the level at which it influences kidney function. The enzymes NAD(P)H and superoxide dismutase are present in both the cortex and medulla of the kidney, with the level of the latter being somewhat higher in the medulla. This group demonstrated that oxidative stress increased the activity of NAD(P)H, but not superoxide dismutase, in the cortex but not the medulla. This would suggest that in renal oxidative stress whereas superoxide anion generation would increase, the level of scavenging would remain unaltered, implying greater activity of the reactive oxygen species. This is important in terms of renal sympathetic nerve activity, as Shokoji et al. demonstrated that direct application of tempol or DETC, a blocker of superoxide dismutase, directly onto renal sympathetic nerve fibers decreased and increased nerve traffic, respectively. These findings imply that oxidative stress in the kidney can itself alter the level of reactive oxygen species in the local environment, which may directly affect the level of renal sympathetic nerve activity and renal nerve-dependent function.
Control of the Renal Circulation
Activation of Renal Sympathetic Nerves
The earliest studies by Cohnheim and Roy using a renal plethysmograph, demonstrated that renal volume was decreased following asphyxia or when the cut ends of the renal nerves were stimulated. Indeed, these early plethysmographic studies also identified the spinal origin of the vasoconstrictor fibers. The advent of the modern flowmeters to measure renal blood flow dynamically allowed studies in anaesthetized dogs, cats, rabbits, and rats which convincingly demonstrated that direct electrical stimulation of the renal nerves caused frequency related reductions in renal blood flow. Furthermore, reflex activation of the renal sympathetic nerves, either as a consequence of activation of the baroreceptor reflex by reduction in carotid sinus pressure or activation of the somatosensory system resulted in a renal nerve-mediated reduction in renal blood flow. Together, these reports indicate that renal nerves cause contraction of the vascular smooth muscle of the resistance vessels, thereby reducing blood flow. While the innervation of the afferent arteriole would cause a reduction in renal blood flow because it is the major resistance bed within the kidney, the impact and relative importance of a neurally induced vasoconstriction at the efferent arteriole is less clear cut in terms of its overall contribution to the reduction in renal blood flow. Luff and co-workers were able to identify at the ultrastructural level two types of fibers in the rabbit that were differentially distributed, one type solely innervating the afferent arteriole and a second type evenly distributed to both afferent and efferent arterioles, which they argued enabled an independent regulation of the two resistance vasculatures. However, an alternative view is that because of the differing wall thicknesses and lengths of the afferent and efferent arterioles, even if both vessels constricted to a similar degree, the efferent arteriolar constriction would have a greater impact on glomerular filtration pressure, and hence filtration rate.
A number of studies have reported a disparity in the magnitudes of the reduction in renal blood flow and glomerular filtration rate produced by renal nerve stimulation. Studies in the anaesthetized rabbit, cat, and rat demonstrated that modest neurally induced reductions in renal blood flow (of 15–20%) were accompanied by either no change or a small 2–5% reduction in glomerular filtration. However, if angiotensin II activity was reduced by converting enzyme inhibitors, angiotensin II type 1 (AT-1) receptor blocking drugs or β-adrenoceptor antagonists, the neural impact on glomerular filtration rate became greater, with the magnitude of reductions in glomerular filtration rate becoming roughly proportionate with renal blood flow. These reports gave rise to the important concept that the renal nerves, indirectly via locally produced angiotensin II acting at the efferent arteriole, could ensure that over a modest range of variation in renal blood flow, the glomerular filtration rate, and hence filtered load presented to the nephrons, was maintained at a relatively constant level.
Renal Denervation
An important question is whether under basal, unstressed conditions the renal sympathetic nerves have a tonic influence on basal blood flow through the kidney. The reasons for this uncertainty reside in the manner of the experimental studies, whether anaesthetized or conscious preparations were used, the degree of surgical stress and type of anaesthesia used, and, to a degree, the species under study. Thus, in a number of reports in the anaesthetized rat, there was very little change in renal blood flow following acute renal denervation. However, in these studies there was often a relatively long period of time between basal measurements, the surgical manipulation and denervation of the kidney, and the post-surgery measurements, with the result that it was difficult to determine whether changes had taken place. In an attempt to resolve this issue, Kompanowska-Jezierska and colleagues inserted an electro-cautery wire around the bulk of the nerves, and instantaneous denervation occurred when a current was passed to destroy the neural tissue. Under these conditions, it was found that over the first 10 to 20 minutes an increase of some 20% in blood perfusing the outer cortex occurred when measured by laser Doppler flowmetry, suggesting there was sufficient activity within the renal nerves to decrease basal renal blood flow. This view was to a degree supported by the conscious rabbit reports of Malpas and co-workers, who found that seven days after renal denervation, renal blood flow was some 55% to 65% higher in the denervated compared to the innervated kidney, the large difference most likely indicative of greater basal sympathetic outflow in the rabbit.
The studies of Miki and co-workers using the conscious rat showed that while basal renal blood flow in the groups of animals with either intact or denervated kidneys could not be distinguished, an increase in renal sympathetic nerve activity during grooming and movement caused a proportionate decrease in blood flow to the intact kidneys of some 15%, whereas that to the denervated kidneys tended to increase in line with blood pressure. The relationship between renal blood flow and stress-induced activation of the sympathetic nervous system was the basis of the study undertaken by Brod and co-workers. They demonstrated in man that, in the unstressed state, administration of an adrenergic blocking drug, dibenamine, had no effect on para aminohippurate (PAH) clearance (effective renal plasma flow), but if the patients were tense, anxious, and stressed, the dibenamine administration was associated with a rise in PAH clearance compatible with the view that there was a tonic renal nerve-induced reduction in renal blood flow. Thus, the degree of tonic influence of the renal sympathetic nerves on basal hemodynamics is dependent on the level of stress impinging on the subjects, and in the normal conscious state the renal sympathetic nerves have relatively little impact.
Neural Regulation of Intra-Renal Hemodynamics
Measurement of Intra-Renal Hemodynamics
The measurement of blood flow through the cortical and medullary regions of the kidney is difficult and fraught with technical limitations. Videomicroscopic techniques have been used to evaluate blood flow through single vasa recta vessels. The limitation of many techniques used such as the Rhubidium-86 methodology is single estimations from one kidney, the H 2 -washout method is the accuracy of curve fitting of the data, and the trapping of labeled microspheres in glomerular is only two to three measurements per kidney. More recently, there has been greater use of laser-Doppler technology, which allows continuous measurements of blood perfusion through the cortex and medulla of the same kidney. The limitations of this technique are that the values recorded are not flow, but are a flux measurement derived from the product of the velocity as well as the number of red cells moving through the volume of tissue illuminated by the laser. Consequently, the values arising from this technique represent qualitative rather than quantitative evaluations of blood flow.
Activation of the Renal Nerves
There have been a series of investigations addressing how and whether the renal sympathetic nerves may differentially regulate blood flow through the cortex and medulla. Early studies in this area applying the H 2 -washout approach in the anaesthetized dog indicated that adrenergically-mediated decreases in flow were of comparable magnitude in both cortical and medullary regions. Similar findings were reported using the Rhubidium-86 methodology, in that stimulation of the renal sympathetic nerves caused equivalent decreases in flow through both cortex and medulla. By contrast in later reports, Rudenstam et al. used laser-Doppler flowmetry in the rat and observed a relative resistance of medullary perfusion to decrease in response to renal sympathetic nerve stimulation. Evans and co-workers, using the same technique in the anaesthetized rabbit, demonstrated smaller reductions of the perfusion in the medulla than in the cortex or total renal blood flow. Further studies in rabbits showed that he magnitude of reduction was similar across the medullary region, irrespective of the depth at which measurements were made (inner or outer medulla). This, to a degree, contrasts with the findings in the anaesthetized rat, where the inner medullary perfusion was less responsive than the outer medullary area to renal nerve stimulation. The reasons underlying the differences reported in the sensitivity of the two vascular regions (cortex versus medulla) to adrenergic stimulation are unclear, but they may reside in the differing characteristics of the afferent and efferent arterioles (which may also vary between outer and inner cortical regions), possible variations in innervation density, species variation or the mix of paracrine and autocrine factors residing in the interstitium in these different regions. The potential interactions of all these factors have been considered in detail in recent reviews.
Control of Renal Tubular Solute and Water Transport
Renal Denervation
Claude Bernard first noted that section of the splanchnic nerve of the anaesthetized dog caused an increase in urine flow. Questions arising from this finding were whether the raised fluid excretion was due indirectly to an increase in glomerular filtration rate, whether it was the result of a direct action on the tubular reabsorptive processes of the epithelial cells or a combination of these mechanisms. This problem was addressed by Bonjour and co-workers using the anaesthetized dog, who convincingly demonstrated that the elevated urine flow and sodium excretion subsequent to the section of the renal sympathetic nerves was independent of any changes in glomerular filtration rate. They concluded that the raised fluid excretion reflected a direct influence of the nerves on tubular function. Thereafter, this view was supported by a series of reports using micropuncture techniques which directly examined reabsorptive rates along accessible segments of the nephron. Bello-Reuss and colleagues observed in the anaesthetized rat that section of the splanchnic nerves had a minimal effect on single nephron glomerular filtration rate, but was associated with significant decreases in both absolute and fractional sodium and water reabsorption at the proximal tubule. These conclusions were supported by other investigators at that time, using comparable techniques to directly measure tubular function.
Renal Nerve Stimulation
The removal of the influence of the renal sympathetic nerves represents only one way of illustrating their action, and to fully appreciate their influence a corresponding series of studies were necessary in which the renal nerves were activated. In a ground-breaking study, LaGrange and co-workers, using the anaesthetized dog, found that direct electrical stimulation of the nerves at levels that were sub-threshold for changing either renal blood flow or glomerular filtration rate, caused a 30–40% fall in water and sodium excretion, which was interpreted as a direct action of the renal nerves on tubular fluid reabsorption. A similar situation was found to exist in the rabbit and rat, in that electrical stimulation of the renal nerves at levels sub-threshold for decreasing renal blood flow, decreased both urine flow and sodium excretion. These observations were supported by micropuncture studies which more directly evaluated the tubular actions of the nerves. These studies demonstrated that the absolute and fractional sodium and water reabsorption of the proximal tubule was increased by low frequency direct electrical stimulation of the renal nerves which was without effect on single nephron filtration rate. Moreover, DiBona and Sawin found in the anaesthetized rat that low frequency stimulation of the renal nerves, at rates which did not alter renal blood flow or glomerular filtration rate, also increased fluid reabsorption at the thick limb of the ascending loop of Henle.
Thus, there is a large body of information which substantiates the view that the renal sympathetic nerves, when activated at low rates which have minimal effects on renal hemodynamics, have a direct action on the transport processes of the epithelial cells of the proximal tubule and the thick ascending limb of the loop of Henle. The situation regarding the distal tubule and the collecting duct has not been investigated in depth, primarily because of the technical hurdles required to evaluate reabsorption in these segments in vivo . Interestingly, Bankir and colleagues have reviewed evidence for the concept that activation of vasopressin V2 receptors along the collecting duct not only increases water abstraction, but also activates epithelial sodium channels (ENaC) in the principal cells of this nephron segment. The EnaC-mediated antinatriuresis takes place in association with V2-induced increases in cAMP, and occurs at high plasma levels of vasopressin typically seen in pathophysiological states. The possibility arises that activation of α 2 -adrenoceptors along this nephron segment, which are known to suppress cAMP, could interact with vasopressin to determine the level not only of water retention, but also of the sodium due to ENaC insertion. The relationship between vasopressin and adrenoceptor activation at this nephron segment remains a source of investigation.
Neural Control of Renin Release
Renin Containing Cells
Renin Production
The granular cells of the juxtaglomerular apparatus contain renin. These cells are found in the afferent arterioles at increasing density as the vessel approaches the glomerulus. The origin of these cells has been examined in a study by Sequeira-Lopez et al. using single cell PCR and double immunostaining combined with lineage markers. By transplanting embryonic kidneys between genetically lineage marked mice containing cells for renin, smooth muscle, and endothelial cells with wild-type mice, they showed two distinct populations of cells expressing either renin or smooth muscle cell markers, but never both in the same cell. During the course of maturation, the renin cell began to express the smooth muscle cell markers, suggesting that renin cells could progress into smooth muscle cells and, if required, back into renin cells. Importantly, it appears that the transformation the other way round cannot happen, that is, the smooth muscle cells do not transform into renin cells. The situation seems to be that when demand for renin is high, for example as a consequence of reduced renal perfusion pressure or following a low dietary sodium intake, some smooth muscles will transform back into renin producing cells. Expression of the renin gene generates a specific mRNA which translates into a large protein, pre-pro-renin, which then undergoes processing to generate pro-renin. The pro-renin then undergoes one of two fates, it can be secreted constitutively in an unchanged form, which represents “inactive” renin or it is incorporated into the secretory granules, where it undergoes further modification into mature renin. The secretion of renin occurs when exocytosis of the granules is stimulated. The exocytosis of the granular cells is regulated by two main intracellular signaling molecules, cAMP which stimulates renin release and intracellular calcium ion concentration (Ca 2+ ) which inhibits renin secretion.
Renin Cell Electrophysiology
The membrane potential of the granular cells is determined by a variety of ion channels influenced by cAMP and Ca 2+ . Large conductance calcium-sensitive voltage-activated channels (BKa) are opened by increased intracellular cAMP, resulting in hyperpolarization. L-type voltage-dependent calcium channels (Ca v 1.2) which inhibit cAMP-mediated exocytosis. The normal physiological process is one where the cAMP stimulation of renin granule exocytosis is protected against activation of L-type calcium channel by the hyperpolarization that results from the cAMP induced opening of the BKa. In this way, renin secretion can occur in a regulated manner independent of intracellular Ca 2+ . These relationships and interactions are illustrated in Figure 16.4 .

There are three primary intracellular signaling pathways which interact to determine the rate of renin secretion: cAMP; cGMP; and cytosolic calcium. Good evidence exists that cAMP is the key signaling molecule. In mice with deletion of the G-protein G sα in juxtaglomerular cells, plasma renin is low and the mice are unresponsive to stimuli such as β 1 -adrenoceptor stimulation which utilize cAMP. On the other hand in intact mice, challenges that stimulate adenyl cyclase or suppress phosphodiesterase enzymes result in increased renin release. The role of intracellular calcium in the regulation of renin secretion is only now becoming clear. Angiotensin II-, endothelin-, and ADH- receptor-mediated increases in intracellular calcium suppress the exocytosis of the renin granules. Indeed, the intracellular calcium levels are inversely proportional to those of cAMP, that is, as intracellular calcium decreases there is an increase in cAMP and renin release, and vice versa . It would seem that adenylyl cyclase isoforms 5/6 are inhibited by increased intracellular calcium, thereby determining the rate of cAMP generation. Taken together, the scenario put forward is that cAMP is the primary intracellular signaling molecule eliciting renin release, and this occurs through activation of β 1 -adrenoceptors. The other factors and mechanisms, which exert their actions via changes in calcium entry into the granular cells and therefore the activity of adenyl cyclase 5/6, will serve to modulate the sensitivity of the cAMP-dependent pathways for renin secretion.
Activation of the Renal Nerves
The stimulation of renal secretion and the exocytosis of the granules are regulated through three main routes, the renal baroreceptor mechanism, the macula densa and the renal sympathetic nerves. Many of the early investigations utilized either electrical stimulation or reflex activation of the renal sympathetic nerves, and showed that plasma renin activity or renin secretion was increased which could be prevented by administration of β-adrenoceptor antagonists. Often interpretation of these findings was complicated by the concomitant reductions in renal blood flow and glomerular filtration rate which could have also contributed to the rise in renin release. The reports by Kopp et al. and Osborn et al. demonstrated that electrical stimulation of the renal sympathetic nerves at low frequencies producing no or small decreases in renal blood flow resulted in increases in renin release that were prevented by administration of a β 1 -adrenoceptor antagonist in anesthetized dogs. This view of renin release from the granular cells being due to a direct action of neurally released noradrenaline has been supported by comparable observations in the rabbit and rat, as well as man.
It is now clear that the neurally-induced renin release is associated with a rise in renal renin mRNA. This effect becomes measurable at relatively high levels of renal nerve stimulation over an extended period of time, of approximately an hour, causing large increases in plasma renin activity. Interestingly, a number of reports have indicated that cAMP stimulates renin gene expression by binding to an enhancer region upstream of the gene, as well as increasing the stability of renin mRNA. The mechanisms involve cAMP-dependent phosphorylation mediated by ERK kinases. Thus, the nerve-mediated increase in cAMP may act in several ways, first to cause an immediate release of renin as a result of exocytosis of the granules; second to increase renin gene expression; and third to cause a stabilization and prolongation of the action of renin mRNA, all actions to ensure that sufficient renin is available and stores are replenished.
Neural and Non-Neural Interactions and Renin Release
A number of early observations gave rise to the concept that a background level of renal nerve activity was necessary to allow the differing renin releasing mechanisms to operate normally. It was the initial studies of Stella et al. in the anaesthetized cat that demonstrated that increased renin secretion as a result of reductions in renal perfusion pressure or renal blood flow were blunted in the denervated compared to the innervated kidneys. Furthermore, Johns demonstrated that during reflex activation of the sympathetic nervous system following reduction of perfusion pressure at the carotid sinus, the magnitude of renin release when renal perfusion pressure was reduced was greater in the innervated than denervated kidneys. These findings were extended by Holdaas et al. and Osborn et al. using the anaesthetized dog, who found that furosemide-induced renin release was enhanced when the renal sympathetic nerves were intact compared to that obtained when the kidneys were denervated. Indeed, Kopp and DiBona clearly demonstrated in the dog that the relationship between the magnitude of renin released in response to a particular level of electrical stimulation of the renal nerves was dependent on the prevailing level of renal perfusion pressure. The mechanisms involved at the cellular level remain undefined.
Integration of Renal Nerve Activity and Function
Recruitment of Functionalities
An important consideration after having defined the exact mechanisms by which the renal nerves exert their influence on the different end-points of kidney function, renin release, fluid reabsorption, and renal hemodynamics, is the relationship between the recruitment of these functionalities under normal conditions. At low levels of renal nerve stimulation, which is sub-threshold for changing fluid reabsorption and renal hemodynamics, there is an increase in renin secretion. At somewhat higher levels of renal nerve activation, but again sub-threshold for impacting on renal hemodynamics, not only are there larger increases in renin secretion, but there is also an antidiuresis and antinatriuresis which has been demonstrated in the anaesthetized dog, rabbit, and rat. It is clear that the renal nerve-induced sodium and water retention under these conditions reflects a direct action on proximal tubule reabsorptive processes, as described in micropuncture studies using the anaesthetized rat. At high levels of renal nerve stimulation, the raised renin release and fluid retention is enhanced, but is now accompanied by a reduction in renal blood flow and, depending on the actual level of stimulation, a decrease in glomerular filtration rate. Indeed, this progressive recruitment of renal functionalities has been shown to occur in man in an elegant study by Wurzner et al. who used lower body negative pressure (LBNP) to reflexly activate the sympathetic nervous system. They found that as LBNP was progressively reduced, there were associated proportionate increases in plasma noradrenaline and plasma renin activity, while sodium excretion was only reduced at the lowest LBNP used. During these challenges neither renal blood flow nor glomerular filtration rate were changed. The way in which these functionalities are recruited is illustrated in Figure 16.5 .

There are two important considerations which arise from this pattern of responses. At low levels of renal sympathetic nerve activity, the major impact will be on renin release and sodium and water reabsorption. At these low levels of activity there will only be small increases in circulating renin (50–70%) and reductions in sodium excretion of some 20–40%. However, if these effects persist over a long timeframe, they could have a profound impact on extracellular fluid volume, and thereby on the level at which blood pressure is set. Again, it is worth reiterating that these renin releasing and sodium retaining responses can occur with little evidence of major reductions in renal hemodynamics.
By contrast, when renal sympathetic nerve activity is raised to higher levels by major stressors (for example emotional challenges, severe exercise), then blood flow through the kidney will be markedly reduced. However, it is important to emphasize that these are short acting events, and therefore they are likely to have only minor influences on overall cardiovascular homeostasis.
Patterns of Electrical Stimulation of the Renal Nerves
Patterns of Efferent Activity
The concepts of how the renal nerves influence kidney function have been based largely on experiments in which the sympathetic nerves to the kidney have been dissected out, placed on electrodes, and stimulated using square wave pulses of defined width and voltage at increasing frequencies and constant current delivery. It has been recognized that this pattern of stimulation bears no relation to that passing down the nerves naturally to reach the kidney. Indeed, multifiber nerve recordings have shown the signal to be composed of a bursting nature where larger or smaller numbers of fibers fire together in a coordinated or disparate fashion, with the result that complex patterns are generated. The issue is whether the effectiveness or impact of the nerves on one or more functions might be different if they were stimulated in a way more representative of that occurring under natural conditions.
Lacroix et al. and Nilsson et al., using isolated mesenteric and nasal vessels, demonstrated that larger sympathetically-mediated vasoconstrictions were produced using high frequency bursts of impulses compared to the delivery of the same number of impulses as a continuous train. This was to a degree reinforced by the observations of Hardebo, who found that the high frequency bursting pattern caused a greater release of noradrenaline from cerebral vessels than that achieved with the same number of impulses delivered as a continuous stream. Thus, the amount of neurotransmitter released can be influenced by the exact pattern of stimulation used.
Electrical Activation with Frequency Enriched Stimuli
Hemodynamics and Function
The question therefore arises as to whether the effectiveness or impact of the renal sympathetic nerves could be influenced by the pattern of the stimulation parameters. This was investigated by DiBona and Sawin in the anaesthetized rat using a complex computer generated stimulus pattern basically of a sine wave, within which was embedded a randomly generated white noise signal. The patterned stimulus was designed to approximate that which the kidney might normally be expected to receive. The authors used two levels of intensities, one impacting on renal hemodynamics and one at a lower level which only influenced excretory function. Importantly, they showed that for the same integrated voltage applied to the nerves, the magnitude of reduction in renal blood flow was greater with the sinusoidal patterned stimulus than with the square wave stimulus at all frequencies tested. This data is presented in Figure 16.6 , where there is a clear shift of the frequency–response curve to the left with the sinusoidal stimuli compared to the square wave stimuli. In a similar way, application of sub-threshold stimuli for changing renal hemodynamics had no effect on sodium excretion if delivered in a square wave form, but did cause an antidiuresis and antinatriuresis at the same integrated voltage if the sinusoidal signal was used, and this is illustrated in Figure 16.7 . Thus, together these reports serve to emphasize that the way in which the neural signals are delivered into the kidney can determine the impact on end-organ function.


Hemodynamics
Malpas and colleagues, using the anaesthetized rabbit, investigated whether the bursting pattern of naturally occurring renal nerve activity could in any way determine the dynamic regulation of renal blood flow. Utilizing a similar patterned stimulus, i.e., a sine wave of varying voltage within which square wave pulses were embedded, as against steady square wave pulses, they showed that the frequency of the sine wave could determine the degree of reduction in renal blood flow, even though the total current delivered was the same. The authors proposed that the low frequency pulses in renal sympathetic nerve activity were likely to result in an enhanced dynamic gain to allow the renal blood flow to respond rapidly to normal everyday variations in blood pressure. Interestingly, this hypothesis was not supported by an investigation in the rat in which the renal nerves were similarly stimulated with sine wave modulation with embedded high frequency pulses. These investigators found that there were reductions in renal blood flow with superimposed low frequency oscillations, but that the magnitude of these vasoconstrictor responses were not altered when basal tone was increased by exogenous administration of noradrenaline or angiotensin II. The reasons why there is a disparity between these reports are unclear, but may reflect a fundamental difference in the regulation of the renal vasculature in the rabbit versus the rat.
Analysis of Renal Nerve Activity
The central mechanisms involved in the generation, regulation, and analysis of the sympathetic outflow have been reviewed in detail by Malpas. It has become possible to resolve the frequency and amplitude components, phase relationships, and coherence between renal sympathetic nerve activity, renal blood flow, and blood pressure. These techniques have been applied to gain insight into how low levels of renal sympathetic nerve activity may influence renal function. It is evident that renal sympathetic nerve activity is at a level which tonically reduces renal blood flow, at least, in the rabbit. Moreover, following power spectral analysis of the renal blood flow signal it has become apparent that at a spectral frequency range above 0.6 Hz in the renal sympathetic nerve signal, the nerves exert a tonic vasoconstrictor action on the renal vasculature. However, the lower frequency power spectral peaks, below 0.6 Hz, cause slow cycles of vasoconstriction and vasodilation which, it has been argued, may enhance the responsiveness of the vasculature to other stimuli to allow renal blood flow and glomerular filtration rate to dynamically adapt to ensure constancy of filtered load, and hence fluid processing by the nephron. Indeed, it has been emphasized that this role of the renal nerves is integral to the maintenance of cardiovascular homeostasis.
The situation in the rat appears somewhat different, as many reports demonstrate that under basal conditions the renal sympathetic nerves have a much smaller, if any, influence on basal renal blood flow. DiBona and Sawin evaluated the transfer function gain between blood pressure and renal blood flow under a variety of conditions where renal sympathetic nerve activity was removed or enhanced. There was a characteristic pattern to the transfer function gain under basal conditions. The transfer function gain decreased below zero over the frequency range 0.02 and 0.06 Hz, which was taken to represent a slower tubuloglomerular feedback mechanism of autoregulation, and increased above zero with a plateau region over the higher frequency range 0.1 to 0.2 Hz, which was taken to reflect the myogenic component of autoregulation. They found that acute denervation had little impact on the overall pattern of transfer function gain over the whole frequency range. In contrast, in two pathophysiological states, congestive heart failure and in the spontaneously hypertensive rat where basal renal sympathetic nerve activity is elevated, transfer function gain was suppressed over both these frequency ranges, but was relatively normalized following acute renal denervation. The authors concluded that in the rats with elevated renal nerve activity, the coupling between blood pressure and renal blood flow was overridden such that autoregulation of renal blood flow was impaired. These authors went on to show that this effect was mediated via angiotensin II, as blockade of angiotensin AT1 receptors with losartan depressed the transfer function gain in rats fed on low or normal dietary sodium intake, but not a high dietary sodium intake. Indeed, the outcome of these studies indicated that the renin–angiotensin system enhanced the tubuloglomerular feedback component of autoregulation, but not the myogenic component, and that the elevated angiotensin II increased the efficiency of transfer of the neural signal to control the renal vasculature.
Reflex Regulation of the Renal Nerves
Activity within the renal sympathetic nerves represents an integration of a number of sensory inputs arising from different regions of the body by the central nervous system. Malpas has recently reviewed the central mechanisms regulating sympathetic outflow to the periphery, including that to the kidney. The changing output of renal sympathetic nerve activity represents a means by which renal functionalities, that is, renin release, sodium reabsorption, and renal hemodynamics are regulated at an appropriate level. The different sensory systems sending information into the central nervous system are shown in Figure 16.8 .

Cardiovascular Baroreceptors
High Pressure Baroreceptors
A number of early studies demonstrated that the high pressure baroreceptors in the carotid sinuses exerted an important influence on the neural regulation of the kidney. Studies undertaken in anaesthetized and conscious dogs in which carotid sinus pressure was reduced mechanically or as a consequence of periods of head up tilt increased renal nerve activity, renin secretion, and caused a renal nerve-dependent antidiuresis and antinatriuresis with relatively little change in renal hemodynamics. This means that during everyday activity, the baroreflex control of blood pressure will impact on kidney function; renin release and sodium and water retention to a greater degree than vasomotor tone. However, what has become evident from the conscious rat studies of Miki and colleagues, is that the baroreflex control of renal sympathetic nerve activity is shifted towards higher blood pressure as muscle activity increases, for example during grooming and exercise on a treadmill.
Low Pressure Baroreceptors
The low pressure baroreceptors are contained in the cardiopulmonary areas, and are mechanosensitive nerves embedded in the atria and great veins (superior and inferior venae cavae and pulmonary vein) which respond to stretch. Activation of these sensory nerves by inflation of balloons in the left atria results in a reflex decrease in renal sympathetic nerve activity associated with a rise in water and sodium excretion. While many of these studies were undertaken in anaesthetized preparations, DiBona and Sawin demonstrated in the conscious rat that volume expansion decreased and volume depletion increased renal sympathetic nerve activity. These observations correlated with a key report by Miki et al. in the conscious dog showing that head-out total body water immersion, a maneuver that shifts fluid into the more central compartments of the cardiovascular system, caused a reflex renal sympatho-inhibition associated with a diuresis and natriuresis. The cardiopulmonary receptors were demonstrated to be essential in this reflex, as following chronic cardiac denervation both the renal sympatho-inhibition and excretory responses were markedly blunted when these dogs were subjected to the water immersion challenge.
Somatosensory System
There are mechanosensory nerve fibers in the joints and tendons, chemosensitive nerves within the muscle tissue itself depolarized by metabolites. The skin contains nociceptors and thermoreceptors. Each of these classes of sensory nerves sends important afferent information into the central nervous system to be integrated. At the level of the kidney, an early study by Thames and Abboud demonstrated that sciatic nerve stimulation caused profound reductions in renal blood flow. In the anaesthetized rat, electrical stimulation of the brachial nerves, application of capsaicin subcutaneously to depolarize afferent nerve endings or inhalation of noxious fumes caused increases in renal sympathetic nerve activity and a renal nerve-mediated antidiuresis and antinatriuresis with little change in renal hemodynamics. Interestingly, the magnitude of the renal sympathetic nerve activity responses is under tonic inhibitory control by the cardiopulmonary receptors, as suggested by studies showing that activation of the cardiopulmonary receptors blunts the sympatho-excitatory and antinatriuretic responses to capsaisin-induced activation of the afferent nerves. These studies illustrate the point that the somatosensory system does provide an important input to the brain, but its impact is modulated by input from the cardiovascular baroreceptors.
Visceral System
Less well-studied, but nonetheless important, are the chemo- and mechano sensory nerves in the visceral system. In early studies, Weaver and co-workers demonstrated that activation of chemosensitive nerve fibers in the small intestine of the cat, by bradykinin, caused a reflex activation of the renal sympathetic nerves. Furthermore, they demonstrated that the effectiveness of this sensory input from the gut was under a tonic inhibitory influence from both the low and high pressure cardiovascular baroreceptor.
Chemo- and mechanosensory nerves are also present within the liver, where they modulate renal sympathetic nerve activity and renal nerve mediated fluid excretion. A reduction in plasma sodium concentration in the hepatic portal vein, but not hepatic artery, resulted in increased adrenaline secretion, indicative of a sympatho-excitation, which was blocked by denervation of the hepatic portal vein. Moreover, infusion of a hypertonic solution in the hepatic portal vein caused a renal sympatho-inhibition and a renal nerve-dependent increase in sodium and water excretion. Taken together, these observations lay the foundation of important reflexes whereby food and fluid absorption from the gut initiates an appropriate neural regulation of kidney function to ensure that sodium and water homeostasis is maintained.
The spleen has also been found to be a source of sensory information which can modulate renal sympathetic nerve activity and its functional end-points. Hamza and Kaufman reported that an increase in splenic venous pressure resulted in small rises in blood pressure and reductions in renal blood flow, with the latter being prevented following surgical section of the renal sympathetic nerves. It can be speculated that this relationship becomes important in portal hypertension and liver cirrhosis in eliciting a reflex renal sympatho-excitation which will contribute to the sodium retention associated with these disease states.
Higher Cortical Centers
The role of psychological stress in regulating renal sympathetic nerve outflow and renal function is less clear-cut and far more difficult to study. Nonetheless, chronically instrumented rats have been used and subjected to a range of challenges to engage the higher cortical centers. An arousal stimulus of an air jet stress has been found to reflexly increase renal sympathetic nerve activity along with a renal nerve-dependent antinatriuresis and antidiuresis. However, studies to determine potential control by the cardiovascular baroreceptors of physiological stresses have been difficult to achieve in conscious studies. Nonetheless, the recent observations of Miki and his group have revealed a number of important findings. First, it was apparent that renal sympathetic nerve activity was lower during rapid eye movement sleep than in non-rapid eye movement sleep and became higher as the level of physical activity rose, from quiet awake to grooming. At the same time, there was an adaptation of the baroreflex to function over a higher blood pressure range as the activity of the animal increased. Second, during treadmill exercise, there was an increased sensitivity of the baroreflex relationship, which they interpreted as important in buffering the raised blood pressure under conditions of increased muscular activity. This group has also evaluated the cardiovascular changes induced during “freezing” behavior, when conscious rats are exposed to a high level of white noise. Under these conditions, there was a minimal change in blood pressure, but heart rate decreased while renal sympathetic nerve activity increased, and lumbar sympathetic nerve activity did not change. The alterations in renal sympathetic nerve activity and heart rate during the freezing behavior were largely prevented by prior sino-aortic denervation, which indicated their important contribution to this pattern of responses. Thus, the cardiovascular and sympathetically-mediated responses during the freezing behavior prepare the animal for an active response to the threatening situation. Taken together, it is apparent that in all these different behavioral states central command exerts an important differential regulatory influence on the baroreflex control of sympathetic outflow to many organs, including that to the kidney.
Reflex Control of Selective Functions
Attention has been focused on whether the renal innervation could selectively regulate either renal hemodynamics or tubular fluid reabsorption or renin secretion. The findings of Luff et al. in the rabbit and DiBona and Sawin in the rat demonstrated that two types of structurally different nerve fibers exist within the nerve bundles and within the kidney itself. Evidence has been produced in the rabbit, where glomerular capillary pressure was measured as an indirect estimate of pre- and post-glomerular resistances. Using an angiotensin II clamp, to remove any confounding influences of changes in endogeneous angiotensin II levels, a hypoxic challenge increased glomerular filtration pressure. The authors’ interpreted these observations as reflecting a selective neural regulation post-glomerular vascular resistance, possibly via one of the subtypes of nerve fiber, independent of the renin–angiotensin system.
DiBona and colleagues using the rat analyzed the strength-duration relationship during direct electrical renal nerve stimulation in relation to renal blood flow, urine flow, and sodium excretion. They found a higher stimulation threshold for the nerve fibers involved in regulating renal blood flow than for those involved in regulating fluid excretion. Moreover, activity in single sympathetic fibers innervating the kidney could be selectively modified by different reflexes. Thus, stimulation of arterial baroreceptors, central chemoreceptors, and thermoreceptors activated a large proportion (88%) of spontaneously active fibers, whereas thermoreceptors only increased the firing rate of those fibers which had no spontaneous activity. In a different series of studies, examining patterns of renal sympathetic nerve activity by evaluating the peaks of activity and time between peaks, DiBona and co-workers were able to show that although somatosensory (pinch) and heat stimuli resulted in similar increases in total renal sympathetic nerve activity, it was only the heat stimulus that caused a decrease in renal blood flow. The authors took these responses to indicate that two different stimuli could result in a distinctly different control of sympathetic outflow regulating renal hemodynamics.
It would seem that there is a small, but persuasive, body of evidence for the view that there may be a degree of selectivity of functional control in the neural control of the kidney. There is a need for more investigation of this particular topic.
Central Nervous System
Central Processing
The processing of this sensory information within the central nervous system to generate an appropriate level of sympathetic outflow, not only to the kidney but to other organs, is complex and attention has been directed towards the cardiovascular baroreceptors which exert a major influence on autonomic control. The sensory information from both the high and low pressure baroreceptors pass to the nucleus tractus solitarius (NTS) for initial processing, and subsequently via multiple pathways to the caudal and rostral ventrolateral medulla (CVLM and RVLM) where a complex interaction takes place before the pre-ganglionic fibers are stimulated. The way in which the major sensory inputs feed into these pathways is illustrated and summarized in Figure 16.9 .

The situation regarding the somatosensory and visceral systems and their inputs to the areas regulating the autonomic nervous system are much less defined. The somatosensory system appears to have a direct input at three levels, NTS, CVLM, and RVLM, but the actions exerted at each of these sites, whether excitatory or inhibitory, and the neurotransmitters involved, have not been resolved.
There is now a body of evidence that autonomic control is organized in a patterned manner. Micro-injection of glutamate onto the RVLM elicited an increase in cardiac, splenic, and renal sympathetic nerve activities that were very different in magnitude. In conscious rat studies there is a relatively low degree of autonomic control of lumbar sympathetic nerve activity during treadmill exercise or in the freezing behavior, whereas there are large dynamic responses in renal sympathetic nerve activity. There is also a clear differential control of cardiac and renal sympathetic outflow in the conscious sheep. Thus, following an infusion of hypertonic saline icv, renal sympathetic nerve activity markedly decreased, whereas cardiac sympathetic nerve activity did not change. By contrast, in a model of pacing-induced heart failure, there was a large elevation in cardiac sympathetic, but not renal sympathetic, nerve activity. It was also reported in conscious rats that icv infusion of angiotensin II reduced renal, but not lumbar, sympathetic nerve activity. A fuller analysis of the evidence has been presented by Malpas in a recent review. The exact way in which information from each sensory system is integrated to provide an appropriate output of renal sympathetic nerve activity and neural control of renal function in order to ensure cardiovascular homeostasis remains to be explored.
Brain Angiotensin II
Angiotensin II and Autonomic Pathways
There is accumulating evidence that the renin–angiotensin system and angiotensin II can influence the reflex responses in sympathetic nerve activity generated by the central nervous system. This can arise in two ways, either via circulating angiotensin II or by means of angiotensin II generated locally within the brain at specific nuclei. Moreover, angiotensin II receptors have been demonstrated in many regions of the brain, and particularly at those nuclei involved with cardiovascular control. It is now accepted that in the areas of the circumventricular organs the blood–brain barrier is leaky, enabling angiotensin II to act on nuclei within this region which have been shown to contain a high density of angiotensin II receptors. At these sites the peptide is able to influence fluid balance; it stimulates drinking, induces ADH release, and causes increased sodium reabsorption and renin release. The second route whereby angiotensin II may influence autonomic control is that generated within the brain itself, as all components of the renin–angiotensin system are present, that is renin, angiotensinogen, converting enzyme, and angiotensin II receptors. It is also necessary to emphasize that immunocytochemical, in situ hybridization studies, and mRNA measurements of angiotensin II receptors and angiotensinogen have shown them to be present or their genes expressed at those nuclei involved with autonomic control, that is the NTS, RVLM, and CVLM, and the paraventricular nucleus (PVN), as well as those other nuclei of the subfornical organ and the area postrema which are most likely subject to the action of circulating angiotensin II. Neurophysiological studies have shown that local administration of angiotensin II onto the NTS and RVLM is excitatory and produces a sympathetically-mediated pressor response, but when applied to the CVLM it causes a sympatho-inhibition ; thus, angiotensin II may have different actions and consequences depending on its site of production and action.
Brain Angiotensin II and Neural Control of the Kidney
The functional role played by angiotensin II within the brain in modulating autonomic control, both normally and in pathophysiological states, is only now being elucidated. There are now a number of reports showing that the baroreflex control of renal sympathetic nerve activity was suppressed by angiotensin II in conscious normotensive Wistar rats, and enhanced in the spontaneously hypertensive rat (SHR). In terms of the somatosensory reflex, angiotensin II within the central nervous system is important, in that losartan given icv blocked the renal nerve-mediated antinatriuresis and antidiuresis resulting from nociceptor stimulation and angiotensin II icv restored the renal nerve-dependent functional responses which could be correlated with marked changes in the pattern with which energy was distributed within the renal nerve signal. The central issue which arises is to understand which factors could affect the level of angiotensin II within the central nervous system which would then impact on the degree of autonomic control exerted peripherally and particularly at the kidney. Indeed, it is important to distinguish between acute bolus administration of angiotensin II administered systemically and the application of the peptide as an icv infusion which may be associated with increases in blood pressure and a renal sympatho-excitation.
The level of dietary sodium intake can modulate this very important link between the autonomic control of the cardiovascular system and brain angiotensin II. An early report by Huang and Leenen demonstrated that raised levels of dietary sodium intake in normotensive rats from four to eight weeks-of-age, led to an increase in baroreflex gain associated with a resetting to a higher pressure for renal nerve activity, but not for heart rate. The importance of dietary sodium intake during development has been reinforced by the studies of Osborn and his group, who showed that in rats fed a high-salt diet from four to eight weeks-of-age, low dose infusion of angiotensin II into the brain (icv) over five days caused a sustained increase in blood pressure associated with a renal nerve-dependent antinatriuresis. This was not observed in rats fed a normal or low-salt diet. Further evidence for this role of the renal sympathetic nerves was reported by Houghton and co-workers who found that a bolus injection of angiotensin II icv had vasopressor and renal sympatho-excitatory actions, the magnitudes of which were enhanced in rats fed a high-sodium diet over the period of growth and development that was in part mediated by vasopressin release both systemically and within the spinal cord.
This influence of dietary sodium intake on the contribution of angiotensin II in the brain on central pathways regulating renal sympathetic nerve activity has received some attention. DiBona and co-workers using rats subjected to a low, normal or high dietary sodium intake for two weeks, microinjected an AT-1 receptor antagonist onto the RVLM, and observed a decreased blood pressure and a shift in the baroreflex gain curve for renal nerve activity to a lower pressure in the animals fed a low-sodium diet, but not in those animals fed the normal or high-sodium intakes. Furthermore, it was evident that administration of an AT-1 receptor antagonist to the RVLM blunted the increases in blood pressure, heart rate, and renal sympathetic nerve activity produced by bicuculline, a GABA receptor antagonist, injected onto the PVN. Importantly, the renal sympathetic nerve responses to the bicuculline administration into the PVN were enhanced in the rats subjected to the low-sodium diet, but blunted in those fed the high salt intake.
Together, these findings highlight the importance of the brain renin–angiotensin system and the significance of angiotensin II in determining the sensitivity of neural pathways regulating sympathetic outflow to the kidney. They also emphasize how alterations in dietary sodium intake may impact on these neural control pathways, which may reset kidney function to a level which could predispose the individual to a hypertensive state.
Neural Regulation of the Kidney in Man
Knowledge of the sympathetic nerve control of the kidney in experimental animals under normal physiological conditions is now extensive in relation to the regulation of renal hemodynamics, renin secretion, and tubular sodium reabsorption. Indeed, although not touched upon in this chapter, there is an extensive body of work in relation to the role of the renal sympathetic nerves in pathophysiological states. However, knowledge of the importance and significance of the renal sympathetic nerves in man remains a relatively unexplored area, although their contribution to cardiovascular and renal diseases is increasingly recognized and alluded to above in different sections. The most striking demonstration of their importance in man has come in a report by Krum et al., who utilized an angioplasty catheter inserted into the renal arteries with a probe at the tip which emitted radiofrequencies with sufficient intensity to disrupt the renal nerves passing alongside the renal artery. In a group of patients with hypertension that was resistant to conventional drug based therapy, bilateral ablation of the renal nerves in this way resulted in a reduction in systolic blood pressure of some 30 mmHg which was maintained for at least 24 months after the manoeuvre. Currently, these initial observations are being further investigated in randomized multicenter trials. DiBona and Esler have emphasized that the knowledge gained from the experimental studies is now being successfully translated into therapeutic approaches, and that the role of the renal sympathetic nerves in heart failure, renal failure, and metabolic diseases of diabetes and obesity will now be explored.
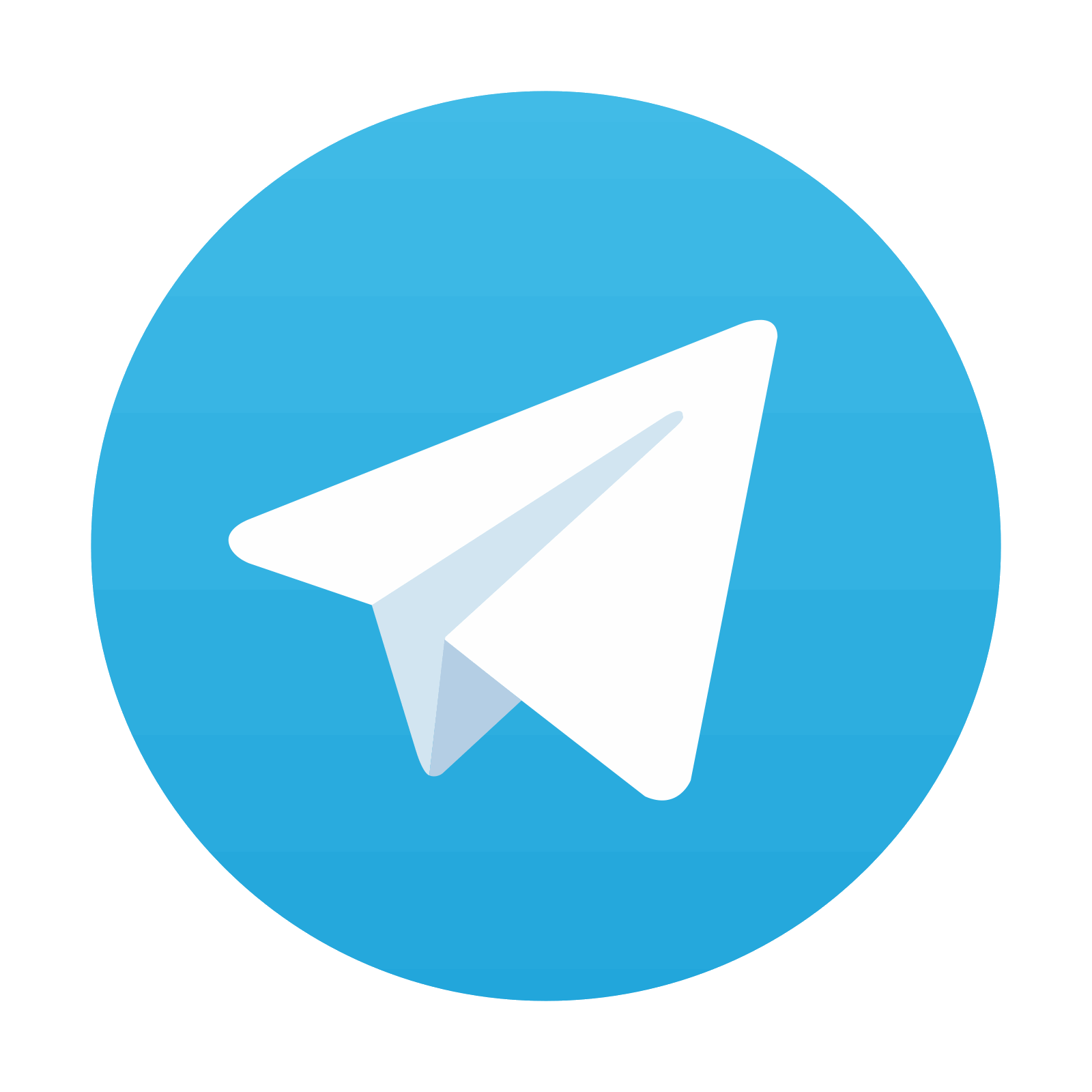
Stay updated, free articles. Join our Telegram channel
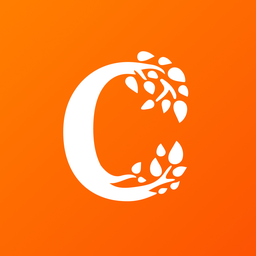
Full access? Get Clinical Tree
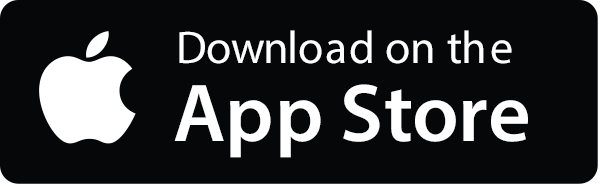
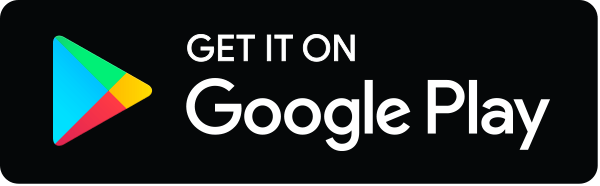