The kidney of vertebrates plays a major role in the homeostasis of the extracellular fluid. Despite large changes in water and salt intake, the kidney is able to maintain the extracellular osmolarity and volume within very narrow margins. Such fine control requires specific factors or hormones. In 1952, Simpson and Tait identified aldosterone as the most potent Na + -retaining factor in mammals. When the Na + -retaining activity of the newly identified steroid hormone was compared to that of cortisol (or corticosterone), aldosterone was found to be 3000-fold more potent in vivo . Aldosterone differs from cortisol (or corticosterone) by the presence of an aldehyde group in position 18 on the second ring. Thus, an apparently minimal change in the structure of the steroid confers on the molecule a strikingly different biological activity (see review in ). During the evolution of vertebrates, aldosterone appeared about 300 million years ago with amphibians, which were the first vertebrates to adapt to a dry, terrestrial environment. The three most important and conserved functions of aldosterone are to promote Na + reabsorption and K + and H + secretion across certain “tight” epithelia – that is, epithelia which display a high transepithelial electrical resistance and an amiloride-sensitive, electrogenic Na + transport. In mammals, such epithelia are found in the distal part of the nephron, the bladder, the distal part of the intestine (mainly the surface epithelium of distal colon and rectum), and the ducts of exocrine glands (salivary, mammary, and sweat glands). In the mammalian kidney, the Na + and K + responses are localized in the segment-specific cells of the aldosterone-sensitive distal nephron (ASDN), while acid secretion is mediated via intercalated cells. The main goal of this chapter is to provide an overview on the renal tubular sites that respond to aldosterone, and to highlight the underlying cellular and molecular mechanisms by which aldosterone controls sodium, potassium, and extracellular fluid homeostasis. The chapter is revised and updated from its previous version established by our colleagues François Verrey, Edith Hummler, Laurent Schild, and Bernard Rossier that appeared in the fourth edition of this book.
Introduction
The kidney of vertebrates plays a major role in the homeostasis of the extracellular fluid. Despite large changes in water and salt intake, the kidney is able to maintain the extracellular osmolarity and volume within very narrow margins. Such fine control requires specific factors or hormones. In 1952, Simpson and Tait identified aldosterone as the most potent Na + -retaining factor in mammals. When the Na + -retaining activity of the newly identified steroid hormone was compared to that of cortisol (or corticosterone), aldosterone was found to be 3000-fold more potent in vivo . Aldosterone differs from cortisol (or corticosterone) by the presence of an aldehyde group in position 18 on the second ring. Thus, an apparently minimal change in the structure of the steroid confers on the molecule a strikingly different biological activity (see review in ). During the evolution of vertebrates, aldosterone appeared about 300 million years ago with amphibians, which were the first vertebrates to adapt to a dry, terrestrial environment. The three most important and conserved functions of aldosterone are to promote Na + reabsorption and K + and H + secretion across certain “tight” epithelia – that is, epithelia which display a high transepithelial electrical resistance and an amiloride-sensitive, electrogenic Na + transport. In mammals, such epithelia are found in the distal part of the nephron, the bladder, the distal part of the intestine (mainly the surface epithelium of distal colon and rectum), and the ducts of exocrine glands (salivary, mammary, and sweat glands). In the mammalian kidney, the Na + and K + responses are localized in the segment-specific cells of the aldosterone-sensitive distal nephron (ASDN), while acid secretion is mediated via intercalated cells. The main goal of this chapter is to provide an overview on the renal tubular sites that respond to aldosterone, and to highlight the underlying cellular and molecular mechanisms by which aldosterone controls sodium, potassium, and extracellular fluid homeostasis. The chapter is revised and updated from its previous version established by our colleagues François Verrey, Edith Hummler, Laurent Schild, and Bernard Rossier that appeared in the fourth edition of this book.
The Aldosterone Sensitive Distal Nephron (ASDN)
Each day, the glomeruli of the human kidney filter about 1.5 kg of NaCl out of the blood into the primary urine. However, usually less than 1% of the filtered NaCl is finally excreted. Thus, most of it is reabsorbed along the renal tubular system, which is composed by serial arrangement of the proximal tubule (PCT and PST), the thin limb (TL), the thick ascending limb (TAL), the distal convoluted tubule (DCT), the connecting tubule (CNT), and the collecting duct (CD) ( Figure 35.1 ). While the bulk of Na + reabsorption occurs in the proximal tubule (PT) and the TAL, the final regulation of Na + excretion takes place in the so-called Aldosterone-Sensitive Distal Nephron (ASDN) which extends from the late portion of the DCT (also called DCT-2) to the end of the collecting duct (IMCD) in the renal papilla. The three ASDN segments differ in their histotopographical localization and epithelial structure. The DCT and CNT are located in the cortical labyrinth, while the CD extends from the medullary rays in the renal cortex down to the renal medulla until its opening on the tip of the renal papilla. DCT cells have an apical localized cell nucleus and numerous mitochondria that are densely stuffed into the interdigitating basolateral membrane infoldings. The CNT cells are usually less tall than the DCT cells, have less mitochondria and a basal membranous labyrinth that is predominantly formed by membrane infoldings. The CCD cells are even smaller, and are characterized by a basal thin rim of mitochondria-free membrane infoldings. The medullary CD is formed by cuboidal cells that have few cell organelles ( Figure 35.1 ). Membrane infoldings and mitochondrial density progressively decrease from DCT to CCD, in parallel with a reduction in the abundance and activity of the Na + ,K + -ATPase, suggesting different ion transport rates along the ASDN. In fact, recordings of transepithelial Na + fluxes in isolated rabbit CNTs and rat CCDs, indicate at least a 10-fold higher Na + transport rate in the CNT than in the CCD (600–120 versus 0.2 to 24 pmoles Na + /min/mm). The rates of Na + transport in OMCD and IMCD are generally low, but Na + channels are also expressed in the OMCD and IMCD, and can be activated, at least to some extent, by aldosterone.

Although the sequence of tubule portions appears to be quite similar in mammals, subtle species differences in the structural and functional organization of the ASDN have to be taken into account if functional data from different nephron portions and species are compared. For example, rabbits have morphologically well-defined sharp transitions from DCT to CNT and from CNT to CD, while the segment transitions in rats, mice, and humans are gradual. These morphological differences are reflected by the distribution pattern of ion- and water-transporting proteins. In rabbits, the sharp transitions from DCT to CNT and from CNT to CCD coincide with the immediate replacement of the DCT-specific NaCl co-transporter (NCC) by the epithelial Na + channel (ENaC), and the abrupt onset of aquaporin-2 (AQP2) expression. In contrast, in rats, mice, and humans NCC and ENaC overlap in the late DCT (DCT2), and AQP2 extends into the connecting tubule.
Despite the above-mentioned segmental and species differences, all ASDN cells in common express high levels of the mineralocorticoid receptor (MR), the glucocorticoid receptor (GR), the enzyme 11-beta-hydroxysteroid-dehydrogenase type 2 (11β-HSD2), and the epithelial Na + channel (ENaC) ( Figure 35.2 ). The MR and the GR are hormone-activated transcription factors that can bind both mineralocorticoid and glucocorticoid hormones, although with different affinities. While the MR is a high-affinity receptor (K d for aldosterone and cortisol in the range from 0.5 to 3 nM), the GR has a more than 10 times lower affinity for these steroids (K d in the range from 20 to 65 nM). The corticosteroid levels in the plasma (cortisol in humans and corticosterone in rats) are usually far above these K d values, and exceed the plasma concentrations of aldosterone 100–1000-fold. Under these conditions, mineralocorticoid specificity is thought to be conferred to the ASDN cells by their high expression of 11β-HSD2 that rapidly hydrolyzes the physiological corticosteroids to inactive metabolites. Upon hormone binding, the receptors are thought to homo- or heterodimerize and to translocate to the cell nucleus, where they modulate a transcriptional program that modulates the expression of ENaC, the Na + ,K + -ATPase, and of regulatory proteins that control the activity of these Na + -reabsorbing proteins.

Na + transport across the ASDN cells occurs in two steps. Na + uptake from the lumen into the cells is mediated by ENaC, while Na + exit across the basolateral plasma membrane depends on the Na + ,K + -ATPase. The activity of ENaC and the Na + ,K + -ATPase is electrogenic, and generates a transepithelial electrochemical gradient that drives paracellular Cl − reabsorption and transcellular K + secretion. The apical K + secretion is thought to occur via secretory K + channels such as the renal outer medulla K + channel (ROMK) and the flow-dependent maxi-K (BK) channel. Immunohistochemical studies revealed that ROMK is highly abundant in the DCT, CNT, and CD cells, where it becomes activated and redistributed to the apical plasma membrane in response to an increased dietary K + intake. Likewise, a high-K + diet appears to stimulate the BK channel. In rabbits, it increases the mRNA expression of the α-, β2-, and β4-subunits, and in rats it elevates the electrical activity in CCD cells. In contrast to ROMK, the BK channel is not only present in the segment-specific ASDN cells, but also in intercalated cells. Intercalated cells may not only secrete but also reabsorb K + via their apical H + ,K + -ATPase. Thereby, intercalated cells may help to modulate the interdependence of ENaC-mediated Na + reabsorption and K + secretion via apical K + channels. A close link between the function of intercalated and segment-specific ASDN cells is also indicated by the fact that, in all species investigated so far, the appearance of intercalated cells in the early ASDN coincides precisely with the onset of functional ENaC expression. Moreover, several recent studies on various rodent models further supported the idea of a functional cooperation between ASDN and intercalated cells. The ASDN has at least two types of intercalated cells. The acid-secreting type-A intercalated cells have the vacuolar H + -ATPase in the apical plasma membrane and the Cl − /HCO 3 − anion exchanger AE1 in the basolateral plasma membrane, whereas the bicarbonate-excreting type-B intercalated cells have the H + -ATPase in the basolateral plasma membrane, and are characterized by the abundance of the Cl − /HCO 3 − exchanger pendrin in the apical membrane, which may contribute to a combined transcellular reabsorption of Na + (via ENaC) and Cl − (via pendrin). In fact, experiments in pendrin-deficient mice indicated that bicarbonate secretion via the type-B intercalated cells is necessary for normal ENaC expression and activity. Consistent with this view, the mice are prone to develop arterial hypotension when placed on an NaCl-deficient diet, and they are protected from hypertension under mineralocorticoid treatment. Pendrin may not only cooperate with ENaC, but also with the Na + -dependent chloride-bicarbonate exchanger SLC4A8, which may account for the previously reported thiazide-sensitive NaCl reabsorption in the renal collecting system. Experiments in SLC4A8- and NCC-deficient mice indicated that about 40–70% of collecting duct Na + -absorption involve SLC4A8. Similar to ENaC, the thiazide-sensitive electroneutral Na + reabsorption, as well as SLC4A8, appear to be stimulated by aldosterone. Na + reabsorption via ENaC may not only interfere with K + , H + , and Cl − handling of the ASDN cells, but also with transepithelial water reabsorption. The ENaC activity generates an osmotic gradient that likely favors the vasopressin-dependent transepithelial water reabsorption via the water channels AQP2 in the apical and AQP3 and AQP4 in the basolateral plasma membrane of the segment-specific ASDN cells. Although there are some indications that aldosterone directly controls the expression and activity of ROMK, BK, pendrin, SLC4A8, AQP2, and AQP3, the functional significance of these regulations compared with the indirect effects mediated via enhanced activity of ENaC and Na + ,K + -ATPase are still unclear. Nevertheless, the various cell types and ion transport pathways with manifold possible interactions likely contribute significantly to the complexity of ion handling along the ASDN which may allow adaptation of urinary ion excretion precisely to homeostatic needs. Details on the regulation of K + channels, pendrin, SLC4A8, and aquaporins are given in several excellent recent reviews.
Aldosterone Action in Non-ASDN Cells
A huge variety of studies have been aimed at localizing MR and GR along the mammalian nephron. Ligand-binding studies and RNAase protection assays on microdissected nephron portions, as well as autoradiography, in situ hybridization and immunohistochemistry on kidney sections, revealed a very high abundance of MR and GR along the classical ASDN. Moreover, these studies indicated that the MR, the GR, and 11β-HSD2 are expressed in nephron segments and cell types that are considered not to be classical targets for aldosterone, including glomerular mesangial cells, podocytes, and proximal tubules (reviewed in ). Using a set of highly specific MR antibodies, Ackerman et al. recently confirmed that the MR is expressed along the entire distal tubule, including the TAL and the entire DCT. MR expression in the TAL is in line with studies describing a profound stimulatory effect of aldosterone on TAL Na + transport, but contrasts with other reports that did not reveal any effect of the mineralocorticoid on the expression and activity of the Na + ,K + -ATPase and K + transport in the TAL of adrenalectomized rabbits and rats, respectively. Likewise, there is considerable evidence that aldosterone stimulates NaCl co-transport in the DCT. Velazquez et al . demonstrated that adrenalectomy lowers the electroneutral Na + transport activity (corresponding to NCC activity) in the DCT, and that this effect can be fully restored to normal when aldosterone and/or glucocorticoids are replaced. Consistent with a stimulatory role of aldosterone on the DCT, immunoblotting and immunohistochemistry on rat kidneys showed that dietary Na + restriction, which stimulates endogenous aldosterone production, as well as exogenous aldosterone application, increases the abundance and phosphorylation of NCC in the DCT. Frindt and Palmer used in vivo cell surface biotinylation to show in the rat that dietary Na + restriction does also increase the cell surface abundance of NCC. Conversely, dietary Na + -loading removes NCC from the plasma membrane, indicating that aldosterone may affect the trafficking of NCC as well. Interestingly, the increase in NCC protein abundance and phosphorylation was significantly lower when MR was blocked by spironolactone. Although the effect of spironolactone points to an MR-mediated transcriptional response, changes in plasma aldosterone do not appear to directly affect NCC mRNA expression, indicating that mineralocorticoids elicit their effects on NCC abundance via enhanced translation and/or reduced degradation of the protein. In fact, there is increasing evidence that NCC protein abundance might be controlled by ubiquitylation, and hence by altered protein stability. Aldosterone is also known to stimulate renal H + excretion. The intercalated cells in the ASDN play an important role for renal acid–base handling. However, so far it is unclear whether aldosterone directly affects renal acid secretion via activation of the H + -ATPase in intercalated cells or indirectly via activation of ENaC-dependent Na + transport in principal cells, which increases the electrochemical driving force for H + secretion by intercalated cells. Functional studies on isolated collecting ducts indicated that aldosterone rapidly stimulates intercalated cells via non-genomic pathways that may involve PKC and PKA signaling, and perhaps require the vasopressin V1 receptor. Nevertheless, it is uncertain whether the observed effects depend on a yet-unknown putative membrane-bound corticosteroid-binding receptor or the classical MR. Although expression of the MR in intercalated cells was long disputed, a recent immunohistochemical study established rather strong expression of the MR, not only in the ASDN segment-specific cells, but also in all subtypes of intercalated cells. However, this study did also provide evidence that the rather low expression levels of the 11β-HSD2 in TAL, early DCT, and intercalated cells, makes it unlikely that aldosterone has significant direct genomic effects on these cell types in the physiological context of several-fold higher plasma levels of corticosterone than aldosterone. Nevertheless, mineralocorticoid-selectivity might be conferred to the MR also in an 11β-HSD2-independent way by a functional preference of the receptor to aldosterone, as indicated in cell culture assays and in vivo experiments. Moreover, MR expression in TAL, early DCT, and intercalated cells may become relevant in patients with pharmacological (e.g., by spironolactone) or genetic (i.e., PHA-1) MR inactivation. Under these conditions, the loss of MR function in these cells may contribute to the salt-losing phenotype with hyperkalemia and metabolic acidosis.
Sodium Transport Regulation by Aldosterone: Physiological and Biophysical Mechanisms
Epithelial Na + transport is a two-step process that comprises: (1) facilitated transport – driven by an electrochemical potential difference – across the apical membrane (from urine to cell); and (2) active transport – driven by metabolic energy – across the basolateral membrane (from cell to interstitium). In the ASDN, the apical-membrane entry step is mediated by Na + -selective amiloride-sensitive ion channels, while the exit step is catalyzed by Na + ,K + -ATPase, the ouabain-sensitive Na + pump. The aldosterone-dependent electrogenic transepithelial Na + transport has been studied in a great variety of in vitro , ex vivo , and in vivo models. The different models revealed different baseline and maximal transport rates in response to aldosterone. In the toad urinary bladder , “basal” transport rates, measured by the short-circuit current method, are around 5–10 µA/cm 2 and aldosterone produces a 2- to 5-fold increase in transport. In the rat colon , basal transport rates through amiloride-sensitive channels are very low, while tissues stimulated in vivo by adrenal steroids can have rates of 500–1000 µA/cm 2 . Isolated , perfused rat cortical collecting ducts express no measurable net Na + fluxes when the animals are maintained on normal diets. When animals are placed on a low-Na + diet (to elevate endogenous aldosterone levels) or injected with mineralocorticoids, Na + reabsorption is as high as 30 pmoles/min-mm, which corresponds to ~100 µA/cm 2 . These values match well the amiloride-sensitive current measured by cell attached patches of mouse CCD cells.
Aldosterone and the Epithelial Sodium Channel ENaC
The apical entry of Na + involves the low conductance, highly selective (Na + over K + ), and amiloride-sensitive epithelial Na + channel (ENaC). ENaC was cloned from rat distal colon by functional expression in Xenopus oocytes. The channel is composed of three homologous subunits denoted α-, β-, and γ-EnaC, which share 30 to 40% identity at the level of their amino acid sequences. In addition to these three well-characterized ENaC subunits, a fourth EnaC subunit, δ-ENaC, has been cloned from a human kidney cDNA library. However, this subunit has been described so far only in humans, and its relevance for transepithelial Na + reabsorption in the ASDN is unclear. When the three classical α-, β-, and γ-ENaC subunits are co-expressed in Xenopus laevis oocytes, the subunits form a channel with functional characteristics similar to the channel identified by Palmer in the rat CCD, with a low 5 pS conductance (for Na + ), a high selectivity ratio of Na + over K + (>20), and a high sensitivity for amiloride with a K i in the submicromolar range. In the Xenopus oocyte expression system, the co-expression of all three ENaC subunits is required for maximal cell surface abundance and activity of the channel. The subunit stoichiometry of the active channel is not finally resolved. Various technical approaches suggested models with four, eight or nine subunits. Recently, the crystal structure of the closely related acid-sensing ion channel isoform ASIC1 A has been reported, and points to a trimeric oligomerization of the channel. However, this trimeric model still awaits further confirmation by establishing the crystal structure of ENaC itself.
ENaC is expressed in several organs including the kidney, the colon, the salivary and sweat glands. ENaC-mediated Na + transport helps to adjust Na + excretion in the urine, feces, and sweat. In lung and airways, ENaC activity is important for alveolar liquid clearance and regulation of mucous fluidity. In the taste buds of the tongue, ENaC is likely involved in salt tasting, whereas ENaC expression in the eye and inner ear may help to control the ionic composition of the aqueous humor and the endolymph, respectively. In the kidney the distribution of ENaC is fully compatible with its involvement in aldosterone-dependent Na + transport. Morphological and functional studies on rodent and human kidneys clearly showed that the ENaC subunits are highly abundant along the entire ASDN, starting in the late DCT (DCT2), and extending through the CNT down to the medullary CD. In the ASDN cells, increased plasma aldosterone levels induced either by dietary Na + restriction or by exogenous application appear to stimulate ENaC mainly by three mechanisms. First, aldosterone increases the abundance of the α-ENaC mRNA and protein, without much affecting the mRNAs encoding for the β- and γ-subunits. In contrast, the β- and γ-subunit mRNAs are upregulated in the colon by aldosterone, while the α-subunit mRNA is expressed constitutively. Second, aldosterone induces the appearance of low molecular weight forms of α- and γ-ENaC, which are thought to represent proteolytically activated channel subunits. Recently, Frindt and co-workers performed cell surface biotinylation experiments on rat kidneys perfused with biotin in situ . These experiments showed that feeding a low-Na + diet or the infusion of aldosterone for one week increases the cell surface pool of cleaved α- and γ-ENaC, but not β-ENaC subunits at the cell surface. Third, aldosterone causes a redistribution of ENaC channel subunits from intracellular storage compartments to the apical plasma membrane. In fact, the subcellular localization of ENaC along the axis of the ASDN changes drastically with altered plasma aldosterone levels in response to altered dietary Na + intake. In rodents kept under a high dietary Na + intake with low plasma aldosterone levels, ENaC subunits are barely detectable at the luminal membrane, and are found almost exclusively at intracellular sites. On a standard dietary Na + intake with moderate plasma aldosterone levels, ENaC subunits are traceable at the luminal membrane in the late DCT and the early CNT, but in downstream segments (i.e., late CNT and CD), particularly β- and γ-ENaC are found almost exclusively at intracellular sites. Only the α-EnaC-subunit was reported to have a more pronounced apical localization and can be found at the apical plasma membrane in CNT and CD. In response to dietary Na + restriction, the apical abundance of ENaC subunits drastically increases due to a translocation of channel subunits from intracellular compartments to the apical plasma membrane. Nevertheless, the axial gradient for apical ENaC prevails, and the apical localization of ENaC subunits remains more prominent in early ASDN than in late ASDN. The activation and apical translocation of ENaC occurs rapidly (within hours), suggesting that they are relevant for the renal adaptation to day-to-day variations of Na + intake. Likewise, injection of aldosterone in adrenalectomized rats induces a rapid (within 2 hours) induction of α-ENaC-subunit expression along the ASDN which is followed by a translocation of all three ENaC subunits to the apical plasma membrane ( Figure 35.3 ). Again, the apical shift occurs predominantly in the early and not in the late ASDN, although the induction of α-ENaC was seen to be similar along the entire segment. Based on previous studies in Xenopus laevis A6 cells, it has been proposed that the induction of α-ENaC might be a prerequisite for full assembly of ENaC in the ER and its subsequent delivery to the cell surface. However, in the kidney in vivo the induction of α-ENaC protein by aldosterone is rather small and, at least in the CCD, not necessarily followed by an apical redistribution of all three ENaC subunits. Moreover, apical targeting of ENaC apparently occurs even without previous α-ENaC induction. Likewise, when ENaC activity was assessed as whole-cell amiloride-sensitive current, ENaC activity in rat CCD was drastically increased within 15 hours of salt restriction, and thus in a timeframe in which no changes in the abundance of ENaC mRNA or protein could be detected. Thus, the induction of α-ENaC alone cannot account for the apical targeting of ENaC. Other co-stimulatory factors are needed. One of these factors was proposed to be the serum- and glucocorticoid-regulated kinase (Sgk1). Sgk1 is a member of the PKB/Akt family of serine/threonine kinases, and is rapidly induced by aldosterone in ASDN model epithelia in vitro , as well as in the kidney in vivo . Likewise, prolonged dietary Na + restriction increases Sgk1 mRNA expression in the kidney. Co-expression of ENaC with Sgk1 in heterologous expression systems profoundly increases ENaC-mediated Na + -currents, most likely by an accelerated insertion-rate of ENaC into the plasma membrane. Consistently, the induction of Sgk1 by aldosterone precedes the apical translocation of ENaC in ASDN cells in vitro and Sgk1-deficient mice ( Table 35.1 ) show mild urinary Na + -wasting that becomes aggravated on dietary Na + restriction. At least part of the effects of Sgk1 on ENaC cell surface abundance is mediated by an Sgk1-dependent phosphorylation of the ubiquitin-protein ligase Nedd4-2. Nedd4-2 phosphorylation blocks the interaction of this ligase with the C-terminus of ENaC subunits, and thereby presumably prevents Nedd4-2-induced ubiquitylation and subsequent endocytosis and degradation of ENaC subunits. Interestingly, immunohistochemical studies showed that the Nedd4-2 abundance increases along the ASDN, which is inverse to the axial gradient of apical ENaC abundance, and further supports the idea that Nedd4-2 is a negative regulator of ENaC cell surface abundance.

Strain | Phenotype | References |
---|---|---|
Signaling Cascade | ||
MR−/− | Severe PHA-1 | |
MR−/−, CNT/CD-specific (MRlox/lox AQP2Cre) | Mild PHA-1 | |
MR−/−, CNT/CD-specific, inducible (MRlox/lox AQP2CreERT2) | Mild PHA-1 | |
GR−/− | Not viable due to perturbed lung development | |
GR−/−, distal tubule-specific (GRlox/lox KspCre) | Slightly elevated blood pressure | |
GR overexpression, CD-specific (hGR Hoxb7-tetON2) | Mild renal sodium retention | |
Mutation GR knockin (hGRM604L) | Increased sensitivity to glucocorticoids | |
11-b HSD2−/− | Severe AME | |
11-b HSD2+/− | Salt-sensitive hypertension | |
Sgk1−/− | Mild PHA1 | |
Sgk1−/− (Sgk1lox/lox c-TgCMVcre) | Mild PHA1 | |
Sgk1−/−, renal tubule-specific (Sgk1lox/lox Pax8/LC1) | Mild PHA1 | |
Nedd4-2−/− | Salt-sensitive hypertension | |
Nedd4-2−/− | Not viable due to respiratory distress | |
Nedd4-2−/− | Cystic fibrosis-like lung phenotype | |
Nedd4-2−/−, renal tubule-specific (Nedd4-2lox/lox Pax8/LC1) | NCC upregulation | |
CHIF−/− | Mild renal and colon defect in Na/K transport |
ENaC | ||
---|---|---|
α-ENaC−/− | Not viable due to respiratory distress | |
α-ENaC−/−, CD-specific (α-ENaClox/lox Hoxb7Cre) | No obvious phenotype | |
α-ENaC−/−, CNT/CD-specific (α-ENaClox/lox AQP2Cre) | Mild PHA-1 | |
Transgenic α-ENaC−/− Tg | Mild PHA-1 | |
Mutation β-ENaCm/m | Mild PHA-1 | |
Mutation γ-ENaCL/L | Liddle’s syndrome, salt-sensitive hypertension | |
β-ENaC−/− | Severe PHA-1 | |
β-ENaC+/− | Very mild PHA-1 | |
γ-ENaC−/− | Severe PHA-1 |
Patch-clamp studies corroborated the immunohistochemically traceable axial gradient of the apical localization of ENaC. The ASDN segments isolated from rats kept on a standard lab chow exhibited no amiloride-sensitive currents at the single channel level, whereas CNTs and CDs isolated from rats with elevated plasma aldosterone levels revealed significant single channel ENaC currents ( Figure 35.4 ). Mice appear to have sizeable ENaC whole-cell currents already on a standard diet, which further increase when animals are kept on low dietary Na + intake. In general, amiloride-sensitive currents decrease in the following order: CNT > initial CD > CD. These findings on apical ENaC localization and activity are consistent with previous studies on microperfused rat tubules, and on isolated rabbit tubules that established several times higher Na + transport rates in early ASDN (i.e., DCT and CNT) than in further downstream ASDN segments (i.e., CD). The axial gradient of ENaC also parallels the progressive decrease of the basolateral Na + ,K + -ATPase activity along the ASDN. Taken together, these data clearly indicate that the aldosterone-dependent adaptation of renal Na + excretion to dietary Na + intake occurs through ENaC, predominantly in the early ASDN, while the late ASDN gets recruited only under high plasma aldosterone levels. Using whole-cell current data, and considering the anatomical length of the two segments, Frindt and Palmer estimated that the Na + transport capacity of the CNT is at least 10 times higher than that of the CD.

The importance of the early ASDN versus late ASDN for the maintenance of Na + balance was highlighted by the development of a mouse model with targeted inactivation of α-ENaC in the collecting duct. These mice survive well and are able to maintain Na + and K + balance, even when challenged by salt restriction or K + -loading ( Figure 35.5 a). Moreover, these mice show a normal urinary acidification in response to furosemide and thiazide treatment, suggesting that functional expression of ENaC in the CNT is sufficient for maintaining ion homeostasis. In contrast, mice with targeted inactivation of α-ENaC in CD and CNT show symptoms of renal salt-wasting ( Figure 35.5 b). Already under control conditions, the mice have significantly higher urinary Na + excretion and increased plasma aldosterone levels. With dietary Na + restriction, the mice start to decompensate and exhibited at day 15 a more than 12% reduction in body weight and exceedingly high plasma aldosterone levels (~20,000 pg/ml in knockout mice versus ~1200 pg/ml in control mice). Moreover, the mice show hyperkalemia, that worsens with dietary K + -loading. Furthermore, the mice are polyuric, consistent with the idea that ENaC is important not only for renal Na + and K + handling, but also for the control of water reabsorption.

Similar to this CNT and CD α-ENaC knockout model, mice with targeted deletion of the MR in the CD and CNT, but intact MR expression and ENaC regulation in early CNT and DCT2, show impaired Na + balance ( Figure 35.6 ). Although these mice develop normally under a standard diet, they continuously lose body weight and show signs of severe extracellular volume contraction under a low-Na + diet. These studies did also show that the MR is crucial for the increased expression of αENaC and apical targeting of all three ENaC subunits in response to dietary Na + restriction. Interestingly, these observations in mice with genetic deletion of the MR contrast with findings in rodents with pharmacological inhibition of the MR. In rats, blockade of the MR by spironolactone does not prevent the apical translocation of ENaC in response to dietary Na + restriction. Likewise, the combined inhibition of the MR and GR by spironolactone and RU486 does not block the induction of αENaC and ENaC trafficking induced by aldosterone-infusion. Together with the observation that the severe symptoms of pseudohypoaldosteronism in MR-deficient mice can be improved by glucocorticoid treatment, and that mice with CNT/CD-specific MR-deficiency have a milder phenotype than mice with CNT/CD-specific deletion of αENaC, the data point to some redundancies in the MR and GR-dependent signaling pathways that control ENaC function.

ENaC-Related Genetic Diseases Underline Pathophysiological Relevance
The discovery that rare monogenic diseases caused by mutations in the genes encoding the ENaC subunits cause defects in the control of Na + balance and blood pressure provided direct evidence for the implication of this channel in these processes. Remarkably, these diseases represent on the one hand, a gain-of-function phenotype (Liddle’s syndrome), and on the other hand a loss-of-function disease (pseudohypoaldosteronism type I or PHAI).
Liddle’S Syndrome
Liddle’s syndrome is a rare disorder (pseudoaldosteronism) described first by G. W. Liddle in a family in which multiple siblings had early onset of severe hypertension associated with hypokalemia. Urinary excretion of aldosterone was low in these patients, and no effects of spironolactone on blood pressure could be demonstrated. By contrast, administration of the Na + channel-blocker triamterene, together with restriction of salt intake, tended to normalize blood pressure. These clinical features suggested that hypertension was due to excessive aldosterone-independent Na + reabsorption in the distal nephron, due to the constitutive activation of ENaC. Despite the low plasma aldosterone in these patients, they still responded to aldosterone injections by further decreasing their renal Na + excretion.
The pedigree of the original kindred described by Liddle was in the meantime extended, clearly demonstrating the autosomal dominant inheritance of the disease. A candidate gene approach was used to identify mutations causing this rare Mendelian form of hypertension. Complete linkage of the Liddle’s syndrome to a locus encoding the β-subunit of ENaC, and analysis of the β gene revealed various mutations in the cytoplasmic C-terminus: deletion or frameshift mutations leading to truncation of a major portion of the C-terminus; and missense mutations in a highly-conserved proline-rich domain. A deletion of the C-terminus in the γ-subunit has also been identified as a cause of Liddle’s syndrome, whereas no mutations in the α-subunit have been linked to this disorder. Expression of these β- and γ-ENaC variants, together with α-subunits in the Xenopus oocyte system, revealed a significant increase in ENaC channel activity at the cell surface, consistent with the initial postulate that these hyperactive channel mutants lead to an excessive Na + reabsorption and hypertension.
To elucidate the causal relationship between dietary salt intake, genetically-determined salt handling by the kidney, and hypertension, a mouse model for Liddle’s syndrome was developed by introducing the R566stop mutation at the β-ENaC allele (“knockin” strategy). Interestingly, with a normal-salt diet, mice carrying the β-ENaC mutated L/L allele remain normotensive, despite evidence of hypervolemia and increased Na + reabsorption in the large intestine. Moreover, plasma pH, Na + , K + , Cl − or HCO 3 − concentrations were not significantly affected. However, these mice manifested the classical Liddle’s phenotype with higher blood pressure, metabolic alkalosis, and hypokalemia, accompanied by cardiac and renal hypertrophy in response to a high-salt diet. Moreover, evidence for impaired ENaC internalization was demonstrated in vivo , as the increase in urinary Na + excretion upon short time (6–12 hours) salt repletion following 1 week of a low-salt diet is significantly delayed in Liddle mice, despite the presence of lower circulating aldosterone concentrations. Isolated perfused CCDs from Liddle mice exhibit higher transepithelial potential differences, and confluent primary cultures of CCDs microdissected from their kidneys exhibit significant lower transepithelial electrical resistance and higher negative potential differences, consistent with an overall increased ENaC activity. Interestingly, mineralocorticoid upregulation of ENaC expression and function is still maintained in Liddle mice that show a remarkable high sensitivity to aldosterone in vitro and in vivo . Renal CD cells from Liddle mice exhibit hyperactive apical vasopressin-regulated CFTR Cl − conductance that could contribute to the enhanced NaCl reabsorption observed in the distal nephron of patients with Liddle’s syndrome. In summary, in the Liddle mouse, dysfunction of ENaC in the kidney is demonstrated before onset of arterial hypertension that argues in favor of the kidney hypothesis proposed by Liddle.
Pseudohypoaldosteronism Type 1
Pseudohypoaldosteronism type 1 (PHA-1) is characterized by dehydration, hyponatremia, hyperkalemia and metabolic acidosis, despite elevated plasma aldosterone concentration and plasma renin activity. The association of renal Na + -wasting, hyperkalemia, and failure to respond to mineralocorticoids suggest a defective Na + reabsorption in the distal nephron. There are two forms of PHA-1, an inherited recessive disease characterized by a culminant clinical presentation in the neonatal period persisting in adulthood, and an autosomal dominant or sporadic form which is milder and remits with age. Genetic investigations of kindred with the recessive PHA-1 revealed deletion or missense mutations in each of the three ENaC subunits, resulting in decreased channel function when expressed in Xenopus oocytes. Linkage of PHA-1 to loss-of-function ENaC variants further supports the critical role ENaC in aldosterone regulation of Na + reabsorption.
Constitutive gene inactivation studies for all three subunits of ENaC (α-, β-, and γ-, encoded by Scnn1a , Scnnn1b , and Scnn1g , respectively) revealed a crucial role for each subunit in the survival of the animal (see for review ). Constitutive inactivation of the mouse αENaC gene locus leads to severe respiratory distress with neonatal death, demonstrating the crucial role of ENaC in lung liquid clearance at birth. The αENaC knockout neonates died so rapidly from their lung phenotype that they did not manifest any electrolyte disturbances. However, mice with a genetic rescue by reintroducing the αENaC-subunit under the control of a heterologous (cytomegalovirus) promoter demonstrated the importance of precise regulation of ENaC in the kidney. The transgenic rescued mice had sufficient basal Na + absorptive capacity to clear lung liquid and survive the early neonatal period, but developed a severe PHA-1 like phenotype with renal salt-wasting, metabolic acidosis, high aldosterone levels, growth retardation, and increased early mortality. In contrast to the αENaC knockout mice, mice with constitutive inactivation of βENaC and γENaC revealed severe early renal dysfunction. Urinary Na + -wasting, K + retention, and increased plasma aldosterone concentrations go along with failure to thrive and early postnatal deaths (see for review ). It seems that low residual ENaC activity in these mice is sufficient to circumvent the neonatal lung phenotype, consistent with the assumption that αβ- and αγ-subunit combinations can establish some ENaC activity in airway epithelia. Inactivation of the γ-ENaC-subunit in mice resulted in early death (at 36 hours after birth) of the mice, mainly due to disturbed total body electrolyte balance. In the course of generating a mouse model for Liddle’s syndrome by the insertion of a stop codon corresponding to residue R566 in human β-ENaC, together with the selection marker neomycin, mice were obtained with a partial disruption of the β-ENaC gene locus (β-ENaCm/m). Under a normal-salt diet, these mice show a mild PHA-1 phenotype with reduced ENaC activity and elevated plasma aldosterone levels, but develop an acute PHA-1 with continuous weight loss, hyperkalemia, and decreased blood pressure when kept under a low-salt diet.
In summary, although the onset and severity of PHA-1 symptoms vary in these models, the renal phenotype of these mice corresponds well to the human PHA-1 phenotype (with salt-wasting, hyperkalemia, and metabolic acidosis), revealing the important implication of ENaC in Na + , K + homeostasis.
Aldosterone and K + Secretion
The ASDN of mammals also plays a critical role in the maintenance of K + homeostasis. In this nephron segment, Na + reabsorption through ENaC is electrically coupled with K + secretion: increasing the Na + influx depolarizes the apical membrane and increases the driving force for K + secretion into the lumen, and vice versa (see Figure 35.2 ). Pathophysiological conditions where Na + absorption is increased in the ASDN (hyperaldosteronism, pseudoaldosteronism) are associated with hypokalemia; conversely, ENaC loss-of-function in PHA-1 results in a loss of Na + absorption in the ASDN and hyperkalemia (see above). Consistently, mice with constitutive inactivation of β- and γ-ENaC show renal Na + -wasting and elevated plasma aldosterone level that goes along with renal K + retention and hyperkalemia. While mice lacking the α-ENaC subunit die soon after birth due to respiratory insufficiency related to impaired alveolar fluid clearance, mice with targeted inactivation of α-ENaC in the CNT and CD survive, and show not only renal salt-wasting, but also hyperkalemia, further confirming the critical role of ENaC for Na + and K + homeostasis.
The K + secretion in the ASDN is mediated by a K + conductive pathway in the apical membrane of principal cells. Small conductance inwardly rectifying K + channels, termed SK channels, account for most of the apical conductance of principal cells. These SK channels have been identified at the molecular level and are the product of the ROMK channel gene. Moreover, the large, Ca + -activated K + channel (BK), present in intercalated and/or principal cells, is thought to contribute to K + secretion along the ASDN. Experiments in mice deficient for either ROMK or the α-subunit of BK suggest that the channels may compensate for each other. The functional role and the regulation of these channels in the kidney have been the topic of several recent reviews, and are also extensively addressed in a separate chapter in this book. Therefore, we will here only briefly touch the possible impact of aldosterone on renal K + secretion via these channels.
High dietary K + intake and elevated plasma K + concentrations increase aldosterone secretion by the zona glomerulosa of the adrenal gland and stimulate urinary K + excretion. This raises the question of whether aldosterone has a primary effect on K + secretion, via genomic effects on principal cells of the ASDN. However, although some studies indicated that aldosterone may directly affect the activity of the renal secretory K + channels, there is no strong evidence that aldosterone at physiological concentrations increases the apical K + conductance of principal cells in the CCD in vivo . In fact, rats fed with a low-Na + diet have a high plasma level of aldosterone and show a large increase in the density of active ENaC channels in the apical membrane of principal cells, but the density of ROMK channels remains unchanged. Recently, Fodstad and co-workers described that the established mouse CCD cell line (mCCD(cl1)) expresses ROMK mRNA and a robust Ba + – and tertiapin-Q sensitive K + conductance in the apical membrane. Overnight exposure to 100 nM aldosterone did not significantly change the K + conductance, but it increased the amiloride-sensitive Na + transport. Surprisingly, in this cell model the mRNA levels of all ROMK isoforms measured by qRT-PCR were even decreased by 15–45%, further supporting the idea that aldosterone does not directly stimulate ROMK.
Nevertheless, a high dietary K + intake increases both the activity of ENaC and of ROMK ( Figure 35.4 ). Interestingly, the kaliuretic response to dietary K + occurs very rapidly, and even before plasma K + and aldosterone levels are elevated. Moreover, renal kaliuresis can be clearly dissociated from renal Na + handling (for review see ). In a recent study, Frindt and Palmer addressed the question of whether the concomitant activation of ENaC is required for enhanced urinary K + excretion. Rats were kept on different K + diets, and then infused via osmotic minipumps with a constant dose of amiloride which was supposed to block more than 98% of Na + transport through ENaC. Measurements of urinary K + excretion indicated that on a control diet (0.6% KCl), distal nephron K + secretion completely depends on ENaC, while on a high-K + diet (10% KCl) an increasing fraction of K + excretion is independent of Na + channels.
Taken together, the current evidence suggests that the activation of ENaC by aldosterone is sufficient to increase the driving force for K + secretion, leading to an increase in kaliuresis, without upregulating K + channels. In contrast, a high-K + diet increases the density of active ROMK channels, suggesting the presence of kaliuretic factors that control K + secretion, independently of aldosterone.
Aldosterone and the Na + ,K + -ATPase
For efficient transepithelial sodium reabsorption, apical Na + entry via ENaC has to be matched by Na + extrusion across the basolateral plasma membrane via the Na + ,K + -ATPase. Consistently, the activity of the Na + ,K + -ATPase is also regulated by aldosterone (for a review see ). Briefly, it was already shown 30 years ago that aldosterone induced augmentation of Na + ,K + -ATPase in the CCD of adrenalectomized rats after 1 to 3 hours of aldosterone infusion. In non-adrenalectomized animals, aldosterone also induced the Na + pump, but the response was delayed and observable between 2 days and 1 week. In the rabbit CCD, Sansom and O’Neil observed a doubling in the basolateral membrane conductance after 24 hours of the mineralocorticoid DOCA (deoxycorticosterone acetate) treatment, which could be accounted for by a rise in basolateral K + and Cl − conductance. Moreover, morphometric studies revealed a significant increase in the basal side surface area of the rabbit CCD principal cells, which can be correlated with an increase in single cell capacitance. However, these changes in cell surface area could only partially account for the increase in basolateral membrane conductance. It is not clear in these cases whether the mineralocorticoid-induced increase in basolateral membrane conductance is a direct effect of the hormone or secondary to an increase in Na + entry into the cell.
As discussed in, it is likely that the early effects involve activation and/or translocation to the basolateral membrane of pre-existing Na + ,K + -ATPases, whereas the late effects are caused by de novo synthesis of the Na + ,K + -ATPase. Indeed, it has been shown both in adrenalectomized rats and in mpkCCD cl4 cells that infusion or treatment for 2 to 3 hours with aldosterone strongly increases the cell surface abundance of Na + ,K + -ATPase, whereas the total cellular pool was only affected later. It was shown by the same researchers that Sgk1 is able to increase the cell surface expression of Na + ,K + -ATPase, providing a potential mechanism of how aldosterone may regulate the pump. However, it remains open if the kinase regulates Na + ,K + -ATPase by direct phosphorylation or not.
Hence, aldosterone is able to modify Na + ,K + -ATPase activity by increasing the number of Na + ,K + -ATPase at the basolateral membrane, likely by translocating pre-existing pumps to the surface (short-term regulation), and by increasing the de novo synthesis of Na + ,K + -ATPases. Moreover, aldosterone is also able to increase the basolateral membrane surface. The cellular and molecular mechanisms that are involved in this regulation are only vaguely known, and may involve Sgk1.
Sodium Transport Regulation by Aldosterone: Cellular and Molecular Mechanisms
Non-Genomic Actions of Aldosterone
Aldosterone, similar to many other steroid hormones, has two fundamentally different mechanisms of action, namely rapid, non-genomic effects that are different from the classical and delayed genomic effects, involving MR and GR and the induction of a complex translational/transcriptional response. The non-genomic effects of aldosterone on intracellular signaling pathways have been demonstrated in a variety of animal and human cell types, including those localized in the classical mineralocorticoid target organs. These effects are characterized by short latencies of seconds or minutes, and do not involve changes in gene expression mediated by the transcriptional role of the MR. They may involve MR itself and being sensitive to MR antagonists such as spironolactone or eplerenone or being independent of MR. The existence of an alternative receptor with high affinity for aldosterone has been postulated, which may be membrane-bound or representing a signaling molecule within the cell. Recently it has been shown that aldosterone signals independently of MR through GPR-30, an intracellular G-protein-coupled receptor localized in the endoplasmic reticulum, leading to extracellular related kinase (ERK) activation and apoptosis, and to myosin light chain phosphorylation. It will be important to determine the role of GPR-30 in the ASDN, where its presence has been reported.
The non-genomic effects involve the activation of protein kinase pathways, including mitogen activated protein (MAP) kinases, protein kinase D1 (PKD1), and protein kinase C (PKC). Interestingly, PKCα (a PKC isoform) directly binds in vitro aldosterone via its C2 domain, leading to its autophosphorylation. Hence, PKCα may represent an intracellular aldosterone receptor distinct from the classical MR. PKC signaling is an illustrative example of cross-talk between non-genomic and genomic pathways, as demonstrated in cultured rat cortical collecting duct cells, in which transepithelial Na + transport may be stimulated in the early phase by PKCα and independent of MR, whereas the late phase requires genomic action via MR. Also involved in short non-genomic activation of ENaC may be PKD1 that appears to regulate subcellular localization of αENaC in M1 CCD cells. Together, the number of different non-genomic effects of aldosterone that have been described, the lack of physiological targets in many cases, as well as the potentially diverse modes of action, do not allow us to draw a unifying conclusion. However, it is likely that non-genomic actions and genomic actions of aldosterone interfere in some instances with each other for the control of physiological functions.
Genomic Actions of Aldosterone
Mineralocorticoid Receptor (MR) and Glucocorticoid Receptor (GR)
As discussed above, both MR and GR act as aldosterone receptors, having different affinities to aldosterone. MR is the high-affinity type 1 receptor (K d approximately 0.5 to 2 nmol/l), and GR is the low-affinity type 2 receptor (K d approximately 14 to 60 nmol/l). On the other hand, the glucocorticosteroid hormone cortisol (corticosterone in rodents) binds to MR with high affinity similar to that of aldosterone, and with a higher affinity to GR. Cell-specific hormone and receptor specificity-conferring mechanisms discussed below are necessary to explain the differences in the response to mineralocorticoids and glucocorticoids at the systemic and organ levels. It is important to note that the precise role of these two receptors (MR versus GR), the extent of their functional overlap, and the role of specificity-conferring mechanism are not fully-understood, and the analysis of MR/GR-modified animals may help to clarify these issues.
The clinical importance of the MRs was demonstrated in patients carrying mutations in the gene leading to an autosomal dominant form of pseudohypoaldosteronism, a disease that remits with age. Moreover, various heterozygous loss-of-function mutations in the human MR (hMR) gene have been identified and characterized, including frameshift, nonsense, and missense mutations, and gene deletions. In contrast, a constitutive active mutation in MR (S810L) has been found in patients with an early-onset hypertension that is markedly exacerbated in females during pregnancy. This mutation alters the receptor specificity, and renders progesterone and other steroids lacking 21-hydroxyl groups, normally MR antagonists, to potent agonists of the receptor. Biochemical studies revealed that the S810L mutation induces a change in the receptor conformation, which increases the steroid–receptor complex stability, contributing to the agonistic action.
MR and GR belong to the nuclear receptor superfamily that includes other steroid hormone receptors, including the thyroid hormone, retinoic acid, vitamin D. These nuclear receptors are modulary proteins harboring different conserved domains. The N-terminal domain is the less conserved among nuclear receptors, both in size and sequence, and represents in the case of MR almost half of the protein. This region contains a ligand-independent activation function, which is important for interaction with transcriptional co-regulators, and for intramolecular interactions with the ligand-binding domain (LBD) that lies at the C-terminus. This latter domain is complex, as it harbors regions involved in formation of the ligand-binding pocket, interaction with heat shock proteins, dimerization, and a ligand-dependent activation function, which interacts with transcriptional co-regulators. The centrally located DNA-binding domain (DBD) is the most conserved region of the receptor. It folds into two zinc fingers, in which one zinc atom is tetra-coordinated by four cysteines. The core DBD contains two α-helices; the first one, or recognition helix, binds to the major groove of DNA making contacts with specific bases. This domain also contains segments involved in receptor homo- and heterodimerization. Putative nuclear localization signals are localized in the C-terminal part of the DBD and the beginning of the hinge region. Acting as enhancers or repressors of transcription, steroid receptors target specific DNA sequences; these hormone-responsive elements (HREs) then confer inducibility/repressibility to the genes by the corresponding hormone.
In the absence of a ligand, the corticosteroid receptors and possibly all other members of the steroid receptor gene family are primarily localized in the cytosol and associated to a heat shock protein complex, which includes Hsp90, Hsp70, p23, p48, FK-binding protein 51, 52, 59 immunophilins or cyclophillin 40 ( Figure 35.7 ). This maintains the receptors in an inactive, but ligand binding-competent and stable conformation. Recently it was suggested that this complex also binds via FKB52 to the motor protein dynein, promoting MR translocation into the nucleus. Thereby, the classical concept that MR dissociates from the complex in order to translocate through the nuclear pore was challenged by showing that MR-Hsp90 cross-linked complexes accumulate in the nucleus in an aldosterone-dependent fashion ( Figure 35.7 ). Hsp90 also stabilizes the MR, as inhibition of Hsp90 with the geldanamycin analog 17-AAG (17-Allylamino-17-demethoxygeldanamycin) promotes rapid ubiquitylation by the CHIP (carboxyl terminus of Hsc70-interacting protein) ubiquitin-protein ligase and consequent proteasomal degradation. It was suggested that treatment with 17-AAG may represent an interesting and alternative pharmacological tool to interfere with MR activation. Activation of the receptor by the binding of an agonist or some antagonists results in receptor dimerization. After nuclear translocation, the dimerized receptors will bind to the corresponding hormone-responsive elements and alter the transcription of target gene(s), as do classical sequence-specific transcription factors.

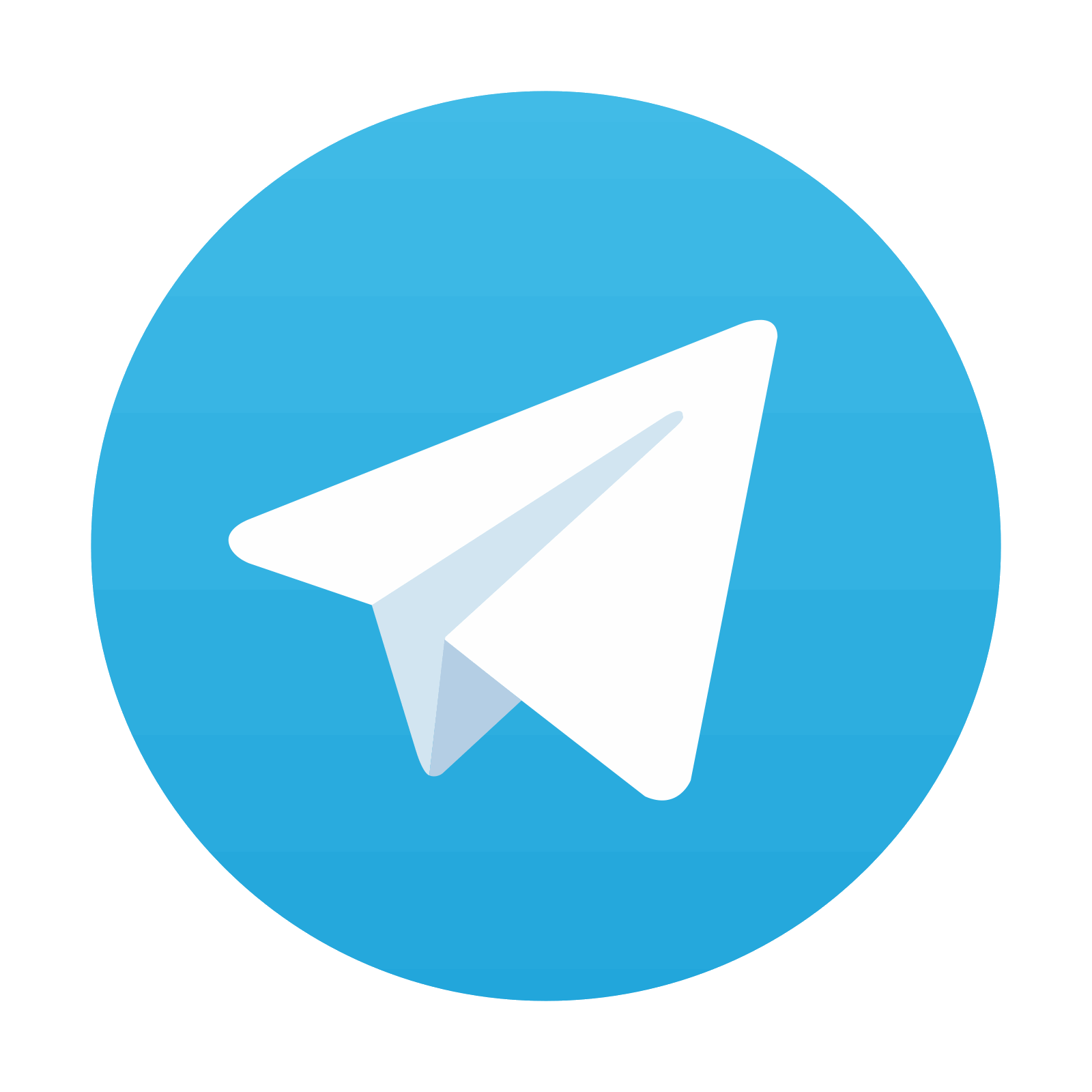
Stay updated, free articles. Join our Telegram channel
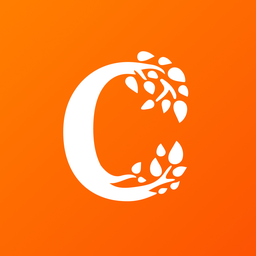
Full access? Get Clinical Tree
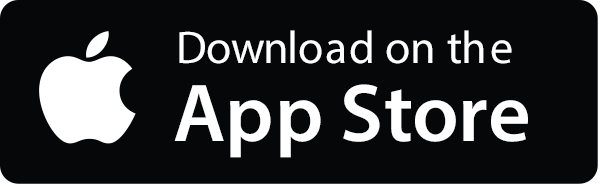
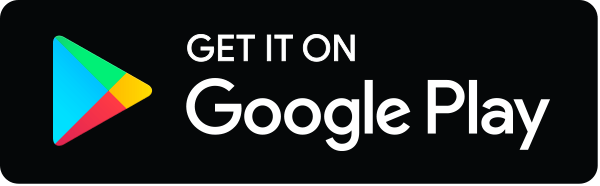
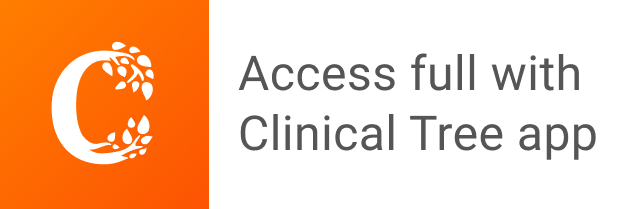