DEFINITIONS: HIGH-EFFICIENCY, HIGH-FLUX, AND PERMEABILITY
It is important to conceptualize and understand the basics of high-efficiency (HE) and high-flux (HF) dialyzer devices in order to compare the uses, differences, and technical needs of each. TABLE 7.2 provides a comparison of the various characteristics of conventional, HE, and HF dialyzers. The definitions of HE and HF procedures were formed primarily from the historical development of HD and the technical needs of each treatment. The primary operating characteristics of HD are blood-flow rate (Qb), dialysate flow rate (Qd), duration and frequency of treatment, and dialyzer characteristics such as membrane surface area and composition, which affects solute dialysance and water permeability. By convention, “small” molecular weight solutes are <500 Da, “middle” molecular weight solutes are 500 to 5,000 Da, and “large” molecular weight solutes are >5,000 Da. Herein, we use urea as the surrogate molecule for “small” solutes and its clearance to define small molecular weight solute clearance. The term efficiency has been used with reference to the small solute or urea clearance. Flux refers to membrane hydraulic (water) permeability. Solute transfer across the membrane is calculated as the urea mass transfer area coefficient (KoAurea), the theoretical maximum urea clearance at infinite Qb and Qd, and is an intrinsic characteristic of the membrane thickness, composition, and surface area. Obviously Qb and Qd are not infinite, but the greater they are, the greater the clearance. In typical HD, urea clearance is much more highly dependent on Qb than on Qd. However, possibly because of internal filtration/convection, dialyzers with higher flux membranes demonstrate a greater dependence on Qd than dialyzers with low-flux membranes (2). In any case, if 100% of a solute is extracted across the dialyzer, the clearance is equal to the Qb. If the dialysate is 100% saturated with the solute, the clearance is equal to the Qd. So clearance can never exceed either the Qb or the Qd. Thus, for practical purposes, the “efficiency” of a dialyzer is dependent on its surface area, membrane solute permeability, and the operational conditions. Once the KoAurea for a given dialyzer is known, the urea clearance under any given Qb or Qd can be calculated or located on a nomogram (3) and used to compare dialyzers. HEHD is achieved primarily by increasing surface area, Qb, and Qd. By most conventions, HE dialyzers have a KoAurea >450 mL/min, which translates to in vivo urea clearance rates >200 mL/min at Qb 300 mL/min and Qd 500 mL/min. This can then be extrapolated to say that dialyzers can be compared and classified based on urea clearance at a fixed Qb and Qd (TABLE 7.2).
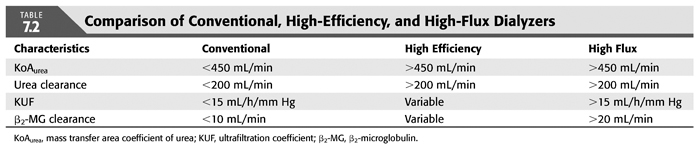
FIGURE 7.1 demonstrates the differences in urea clearances between higher efficiency dialyzers (higher KoA, upper curve) and lower efficiency dialyzers (lower KoA, lower curve) at varying Qb. In lower efficiency dialyzers, urea clearance tends to plateau at lower Qb. By contrast, urea clearance in higher efficiency dialyzers continues to rise with higher Qb and does not plateau until Qb exceeds 400 mL/min. Therefore, an access that can deliver such a Qb is important to take full advantage of HE dialyzers.
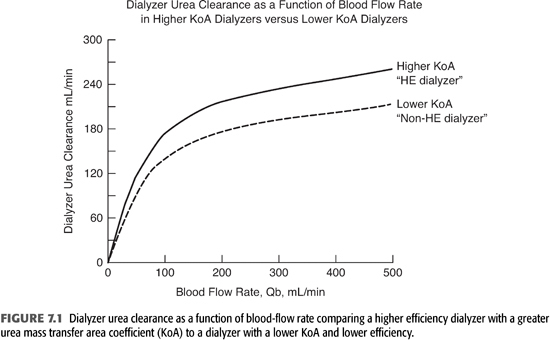
“Flux” refers to the hydraulic (water) permeability of the dialyzer membrane and can be quantified by the ultrafiltration coefficient (KUF) and sometimes additionally by β2-microglobulin (β2-MG) clearance. β2-MG is an 11.8-kDa molecule implicated in the development of dialysis-related amyloidosis (DRA) and is used as a surrogate representation for large molecules. KUF is defined as the ultrafiltration rate (UFR) divided by the transmembrane pressure (TMP) and the units are milliliters per hour per millimeter of Hg of applied TMP (mL/h/mm Hg). TABLE 7.3 lists technical specifications of selected dialyzers commonly in use by the two largest dialysis provider groups in the United States, as examples of how to use the concepts described above.
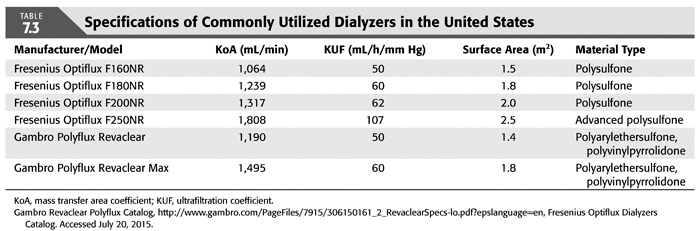
As mentioned, another way to define flux relates to membrane permeability defined by the clearance of substances with a molecular weight greater than 10 kDa. This definition owes its origin to the Hemodialysis (HEMO) trial, where HF membranes were defined by KUF >14 mL/h/mm Hg with a mean β2-MG clearance >20 mL/min (4). The first application of HEHD was characterized by high urea clearance, using cellulosic large surface area membranes, and high KUF in the range of 10 to 20 (5). The high KUF was largely the consequence of the membrane surface area. Cellulosic materials were utilized because of their great tensile strength, which was needed because of the high applied TMP. As membrane synthesis became less costly, the cellulosic membranes were replaced with HF synthetic materials, which had greater KUF, and required less TMP to achieve the same UFR. Hence, dialyzer membranes should ideally be compared under equal operating conditions on the basis of KOAurea, KUF, β2-MG clearance, and surface area, as well as other factors not discussed here such as extracorporeal blood commitment, biocompatibility, and reuse potential.
TECHNICAL ASPECTS
With the increased popularity of HEHFHD in the United States, standard requirements and features must be met in the current dialysis machines and facilities. Precise ultrafiltration control is a standard feature in most currently available dialysis machines, achieved with varying methods. This section is intended to help clinicians ask specific questions of manufacturers and dialysis providers to investigate which systems are best for a particular facility.
Ultrafiltration Control Systems
Precise ultrafiltration (UF) control is mandatory because of the high KUF of these dialyzers, which actually began as hemofiltration cartridges with KUF of >100 mL/h/mm Hg. Control of fluid balance was best achieved with sophisticated computerized UF controllers to match UF and reinfusion of fluid, thereby maintaining hemodynamic stability. This historical perspective is important, since the outgrowth of HE and HF treatments came from the clinical comparison of HEHD and hemofiltration during a short TT therapy. The initial UF control systems applied during the era of hemofiltration were the forerunners of the systems applied today. The original microprocessor-based hemofiltration equipment, produced by Gambro Healthcare (Lakewood, Colorado), utilized a sophisticated strain gauge attached to a load cell that measured the rate of weight change from the 35-L containers used to collect ultrafiltrate and provide reinfusion fluid. This was, in fact, the first true volumetric UF control system. But this system was not directly applicable to an on-line, single-pass dialysis system in which approximately 100 to 160 L of fluid had to be accurately measured. Further improvements in the UF control systems for hemofiltration led to a continuous on-line system that used electromagnetic flow sensors to measure dialysate inflow and outflow. This system was microprocessor controlled and had no mechanical flow components. This is classified as the first microprocessor-based electronic UF control system and is still used in some HD machines. A secondary application of these microprocessor-based electronic UF control systems centered on the development of a bearingless rotor placed in a centrifugal flow path, which spun a ring where the optical characteristics were sensed through a fiber-optic network that then determined flow rates. Instead of being electromagnetically based, they are a combination of mechanical and electronic sensing. Typical examples of this type of equipment were produced by Baxter Healthcare Corporation (Deerfield, Illinois) in their 1550 dialysis machines.
The third major type of UF control system, developed in the early 1980s, utilized a mechanical-based system to control the very high KUF of hemofilters (100 to 200 mL/h/mm Hg) and hemodiafilters. These so-called volumetric control systems are based on the creation of a closed inflow and outflow loop by multiple-valve isolation systems, with a secondary UF pump that removed fluid from the relatively closed circuit at a fixed rate. The closed-circuit system matched dialysis inflow and outflow by bellows displacement pumps as the UF control pump generated the appropriate TMP needed to limit UF during the dialysis treatment. Examples of this type of equipment were the Cobe Century III (Cobe Renal Intensive Care Division of Gambro Healthcare, Lakewood, Colorado), the Fresenius series of HD machines (Fresenius Medical Care, Lexington, Massachusetts), the System 1000 by Althin Medical, Inc (Miami, Florida), the Toray UF control system (Houston, Texas), and the Italian Bellco machine (Bellco S.P.A., Saluggia, Vercelli, Italy). The UF control achieved by these mechanical bellows systems was compatible with both HE and HF dialyzers. Some of these systems may still be available on secondary markets. The current mainstream equipment that meets these requirements include the Fresenius 2008K/K2 machine series.
Bicarbonate Dialysate
Bicarbonate dialysate has become the predominant buffer in the era of HEHFHD. When acetate was the buffering system in the 1980s, the use of more efficient dialyzers with KoAurea >450 mL/min was associated with more frequent intradialytic symptoms and complications. Because of their more rapid diffusion of small solutes, HE dialyzers allowed acetate from the dialysate to accumulate in the patient faster than it could be metabolized (6). High levels of acetate can have a negative inotrope effect and can be vasodilatory peripherally, thus contributing to hypotension (7,8). In addition, acetate dialysate has a low partial pressure of carbon dioxide, which leads to net carbon dioxide removal. By removing the respiratory drive of carbon dioxide, patients were found to hypoventilate, and hypoxemia was a common problem that may have contributed to acetate intolerance (9). Thus, use of acetate contributed to hypoxemia, decreased cardiac output, and hypotension (10). These complications helped to usher in bicarbonate as the dialysate buffer of choice while using the new HE dialyzers.
Bicarbonate dialysis is not without its own complications and technical difficulties. In contrast to acetate dialysate, which is prepared from a single concentrate that inhibits bacterial growth, bicarbonate dialysate must be prepared from two concentrates to prevent precipitation when stored as concentrate containing calcium and magnesium. One of these concentrates is pH neutral and supports bacterial growth, which can lead to contamination. Solutions are buffered to pH of 5.2 to 5.5 to delay bacterial growth during storage.
SOLUTE CLEARANCE
Defining Uremic Toxins
While many “uremic toxins” remain unidentified and unmeasured, the current measurable solutes have been divided based on molecular weight, as mentioned above. While some small molecular weight substances are easily removed and measurable, there are significant implications of the presence of middle molecules on both morbidity and mortality, including their impact on cardiovascular morbidity. To date, there has been suggestion of approximately 40 groups or categories of middle molecules that may have been identified; these are often thought to be proinflammatory (11).
Diffusive removal capacity of middle molecules is dependent on molecular weight, protein binding, properties of the membrane including porosity and surface area, and molecular kinetic behavior of the middle molecule including compartmental distribution (12). Furthermore, middle molecular removal is directly dependent on increased convective clearance strategies, the latter especially important for protein-bound solutes. HF dialyzers thus may confer additional benefits due to their porosity for middle and large molecular weight toxins, for example, β2-MG. Several studies have revealed significantly decreased plasma β2-MG levels in patients undergoing HF therapies (13–16). In a prospective, multicenter study of 380 patients randomized to four different dialysis techniques for 24 months, Locatelli et al. (16) demonstrated substantial reductions in pretreatment β2-MG levels in patients receiving HD (23% reduction) or hemodiafiltration (16% reduction) using HF polysulfone membranes. In contrast, there were no changes in β2-MG levels in the patients assigned to cuprophane and low-flux polysulfone HD (13). These results support the hypothesis that removal, as a consequence of membrane flux, may have a greater impact on β2-MG levels than a lower rate of generation due to biocompatibility. Although a direct relationship between predialysis β2-MG levels and DRA has not been established, Küchle et al. (17) noted a significantly lower incidence of carpal tunnel syndrome and/or osteoarticular lesions among 20 long-term dialysis patients randomized to HF polysulfone membranes versus low-flux cuprophane membranes after an 8-year follow-up. These findings could have important clinical implications, given the significant morbidity related to DRA in long-term dialysis patients.
Middle molecular removal is thus greatly time-dependent in part due to the rebound effect and mobilization from the multicompartmental distribution (4,12). Hence, clearance may be improved by increasing dialysis frequency or increasing session duration. This is clearly demonstrated by almost doubling of β2-MG clearance with increased dialysis time from 4 to 8 hours, despite a similar Kt/Vurea (12,18). While the primary analysis of the HEMO study demonstrated no significant effect of membrane flux on mortality, a subanalysis of the HEMO study demonstrates an inverse relationship between mortality and β2-MG clearance (19). Predialysis β2-MG concentrations fell over the course of the study in the HF arm patients far more than those in the low-flux arm. Similarly, in the Membrane Permeability Outcome (MPO) study, HF dialysis resulted in statistically significant lower β2-MG accumulation over a period of 36 months when compared to low-flux group (20). The MPO study further demonstrated a significant survival benefit with the use of HF dialysis membranes in the subgroup of patients with serum albumin ≤4, a relative risk (RR) reduction of 37% after adjusting for confounders.
As dialysis technology evolved, the required “adequacy goals” were achieved in less time. Hence, dialysis session duration was decreased. Incentives for the facility to shorten session duration were the ability to accommodate more patients per day, spreading costs over more patients, and for patients, less time at the facility. Shorter TTs used increased dialysate quantities with increased Qd, but this cost balanced out with the decreased time. And while increased Qb up to 400 mL/min may improve small molecular clearance, it translates into decreased middle molecular clearance due to decreased session time. We fully agree with Vanholder et al. (21) that the small solute definition of “adequacy” of HD, the concept of Kt/Vurea is too simple to explain or define the complexities of uremia and modern dialysis techniques.
POTENTIAL BENEFITS
Subgroup analysis of the HEMO study concluded an insignificant trend favoring mortality benefit for large-pore, HFHD membranes in achieving the 1.3 Kt/V goal (4,19
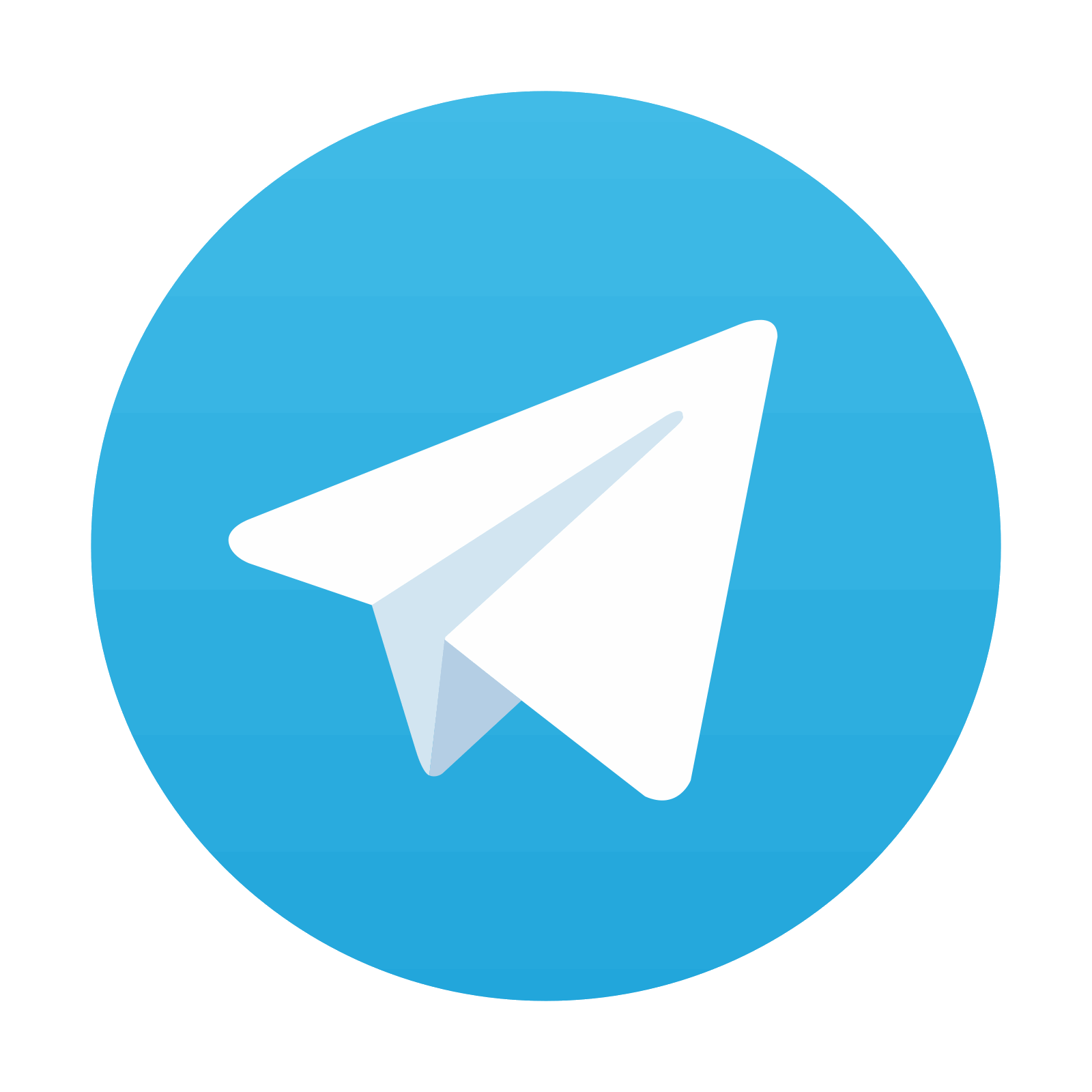
Stay updated, free articles. Join our Telegram channel
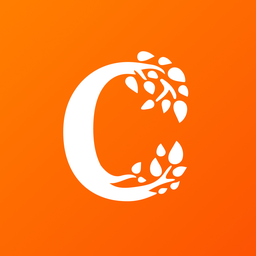
Full access? Get Clinical Tree
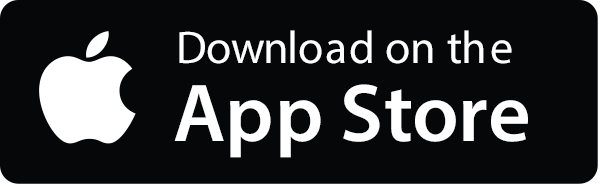
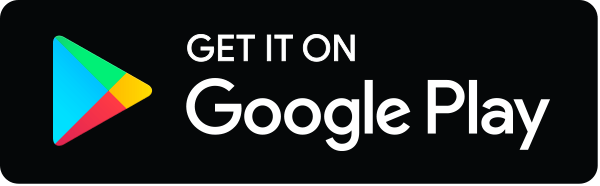