Internal potassium homeostasis is defined as the regulation of potassium distribution between the intracellular and extracellular fluid compartments, as distinct from the net gain or loss of potassium from the body. While the kidney plays the predominant role in maintaining external potassium balance, nonrenal tissues, especially muscle and liver, are quantitatively the most important organs involved in the regulation of internal potassium balance.
The ratio of potassium between intracellular and extracellular fluids is critically important, not only to the behavior of electrically excitable cells, such as muscle and nerve, but also to the vital processes of all living cells. The reason for this is that a major regulator of cell function is the transmembrane potential. The determinants of this membrane potential are described by the Goldman–Hodgkin–Katz equation, the most important term of which is the logarithm of the ratio of internal to external ionic activity of potassium.
Of the 3500 mEq of potassium found in the body of a 70 kg human, about 98% is confined to intracellular water ( Figure 48.1 ). Of this, 80% is contained in muscle cells, at a concentration of about 150 mEq/liter. The remaining 2% of total body potassium (about 70 mEq) is located in the extracellular fluid (about 14 liters), where the normal concentration is 3.5–5.5 mEq/liter. The chief biological mechanism responsible for maintaining this 30-fold potassium gradient between cell water and extracellular fluid is the Na,K-ATPase pump, situated in the plasma membrane of all animal cells. A minor role is played by the inward transport of potassium coupled with sodium and chloride, via the Na K 2Cl transporter in the plasma membrane of some cells. Transcellular distribution of potassium is also modulated by hormonal factors, such as insulin and catecholamines, by hydrogen ion balance, plasma osmolality, intracellular potassium content, and by factors that affect the passive movement of potassium through membrane channels, such as the level of intracellular calcium and pH ( Table 48.1 ). Some of these factors, such as the activity of Na,K-ATPase and the distribution of hydrogen ions, may concurrently affect the potassium content of cells of the distal nephron, and thereby influence the external balance of potassium.

Factor | Effect on Potassium |
---|---|
Insulin | Enhanced cell uptake |
β-Catecholamines | Enhanced cell uptake |
α-Catecholamines | Impaired cell uptake |
Acidosis | Impaired cell uptake and enhanced efflux a |
Alkalosis | Enhanced cell uptake and reduced efflux a |
External potassium balance | Loose correlation |
Drugs | See text |
Hyperosmolality | Enhance cell efflux |
From a practical standpoint, a key determinant of transmembrane potential is the plasma potassium. Since the concentration of potassium inside cells far exceeds extracellular concentration, percentile changes in intracellular potassium are relatively small, even during extreme degrees of total body potassium surfeit, deficit or internal redistribution. The changes in extracellular potassium seen in diseased states are therefore much more likely to alter the membrane potential of cells than are concomitant changes in intracellular potassium. For this reason, a variety of mechanisms have evolved to preserve the extracellular concentration of potassium within the normal range.
If a moderate load of potassium (0.5 mEq/kg) is administered intravenously over 1 hour, about 40% of it is excreted into the urine at the end of that time. Within 3 hours, renal excretion is complete and the serum potassium, which initially increases by about 0.6 mEq/liter, returns to baseline. The ability of the normal human kidney to excrete all of an oral load of potassium is more sluggish; while potassium excretion increases 6-10-fold within a few hours, only about half of the load is excreted during the first 3–6 hours after it is ingested. Of considerable interest, however, is recent evidence that is consistent with the existence of an unidentified “gut” and/or “hepatoportal factor” that senses potassium ingestion, and rapidly initiates the process of renal potassium excretion in the absence of a significant change in plasma potassium concentration.
Consumption of only 35 mEq of potassium by a 70 kg adult during an average meal (an amount equivalent to 1% of total body potassium) would, if confined exclusively to the extracellular space, raise the plasma potassium by 2.5 mEq/liter – enough to have pronounced effects on neuromuscular function. It is well-established, however, that a potassium load given to a normal human or dog has an apparent volume of distribution of 70–80% of body weight, somewhat greater than total body water, instead of the 20% that represents extracellular fluid. In other words, only a small portion (about one quarter) of the 35 mEq of ingested potassium will normally remain in the extracellular compartment, raising the concentration of potassium in plasma by only about 0.6 mEq/liter. In contrast, a similar load of potassium administered to patients with deranged extrarenal potassium homeostasis may produce serious hyperkalemia.
The cells also buffer plasma potassium during potassium depletion. In states of progressive potassium deficiency, as depletion worsens, a greater amount of potassium is lost from within cells to lessen the fall in external concentration, and to minimize the alteration in its intracellular to extracellular ratio.
These examples of potassium surfeit or deficit emphasize the critical role of internal potassium homeostasis in mitigating potentially dangerous changes in the plasma potassium. Disorders of the factors that mediate this adjustment thus may have substantial clinical importance and are the primary topic of this chapter.
Potassium Depletion and Repletion
In many conditions, such as vomiting, diabetic ketoacidosis, and chronic renal failure, abnormalities of internal and external potassium homeostasis coexist. Just as internal potassium homeostasis can affect potassium uptake and excretion by the kidney, so changes in external potassium balance, by altering cellular potassium content, can independently influence internal potassium homeostasis.
Potassium Depletion
The idealized curvilinear relationship between total body potassium and the serum potassium concentration illustrated in Figure 48.2 is derived from several measurements in hypokalemic patients with positive potassium balance during replacement therapy, and from unpublished data on hyperkalemic humans and animals. In the early stages of depletion, extracellular potassium loss is proportionately greater than the loss of cellular potassium. Nonetheless, since only a small fraction of total body potassium is extracellular, the quantity of potassium lost from the extracellular compartment is much smaller than that lost from inside cells.

In the early phases of hypokalemia (>2.5 mEq/liter), patients tend to display an almost linear relationship between total body potassium and the serum potassium concentration. It has been observed that a change of 100–200 mEq in total body potassium (about 5%) is required to lower the serum potassium by 1.0 mEq/liter. In such a situation, the extracellular potassium concentration would be expected to fall proportionately more (e.g., 4.0 to 3.0 mEq/liter) than the intracellular concentration (e.g., 140 to 133 mEq/liter). Because of the relationship of cell membrane potentials to the ratio of internal to external ionic activity of potassium, excessive extracellular potassium loss would be expected to hyperpolarize cells (resting membrane potential is increased). This expectation has been confirmed in studies of early potassium deficiency in both dogs and humans.
When potassium depletion becomes more severe, so that serum levels fall below about 2.5 mEq/liter, a further 1.0 mEq/liter fall will represent a much larger 200–400 mEq decrement in total body potassium (greater than 10%), reflecting a greater degree of potassium loss from within the cells than occurred in the early phases of depletion. Decreased cell potassium content has, in fact, been observed in several tissues during severe hypokalemia.
In severe potassium depletion cells tend to depolarize (resting membrane potential is decreased), at least in dogs which, like humans, then develop weakness and muscle paralysis. Under conditions of chronic hypokalemia, however, there is also upregulation of the colonic form of H,K-ATPase (HKα2), which leads to enhanced potassium reabsorption from the gut.
Potassium Repletion
During potassium repletion for severe hypokalemia, cellular potassium uptake is enhanced both in animals and in humans ; that is, the administered potassium has an increased volume of distribution; as potassium is gained by the body and the stores become higher, the cellular uptake of potassium decreases. In anuric humans, for example, the cellular uptake of a potassium-load decreases as total body potassium increases. Extracellular potassium then tends to rise and membrane potential decreases. The important therapeutic caveat in the late phases of correction of potassium depletion is that less potassium administration is required than in earlier phases to increase serum potassium, which may rise suddenly to unexpected, dangerously hyperkalemic levels.
As reviewed extensively by Sterns et al., the serum potassium alone is, at best, an extremely rough guide for estimating potassium replacement therapy, presumably because other factors, such as acid–base status, influence it. A low serum potassium value (e.g., 3.0 mEq/liter) may be associated with a range of total body deficits spanning a few hundred millequivalents ( Figure 48.2 ).
The exact mechanisms that produce this curvilinear relationship are uncertain. They may stem in part from impairment of the electrogenic sodium pump. During potassium depletion in rats, skeletal muscle potassium loss is associated with a reduced capacity for Na-K pumping and a reversible decrease in the number of [ 3 H] ouabain-binding sites. A possible mechanism for suppression of the Na-K pump during potassium depletion is enhanced stimulation of alpha-adrenoreceptors (see “Catecholamines”). In addition, even modest dietary potassium restriction provokes resistance to insulin-mediated cellular potassium uptake (see “Insulin”).
Insulin
The effect of insulin on potassium homeostasis was first demonstrated two years after its purification by Banting and Best. Harrop and Benedict, and Briggs and Koechig described the fall in serum potassium coincident with lowering of blood sugar when insulin was administered to diabetic patients, as well as in the non-diabetic human, dog, and rabbit. Later, there were reports of severe hypokalemia in insulin-treated patients with ketoacidosis who developed paralysis.
Cellular Mechanism
The hypokalemic action of insulin derives from its capacity to cause net potassium uptake in skeletal muscle, adipose tissue, and hepatic cells, as well as other extrarenal sites. This effect was formerly assumed to occur to preserve electrical neutrality when insulin-mediated glucose uptake produced intracellular anionic sugars and deposited potassium as an accompaniment of glycogen in the liver.
This classical hypothesis did not explain the clinical observation that sudden lowering of serum potassium could precede the fall in blood sugar in insulin-treated diabetic coma. Zierler first noted that insulin’s effect on potassium movement in rat muscle occurred even in the absence of glucose; its known effect to increase sodium efflux in vitro also occurred without glucose. Furthermore, enhancement of potassium disposal in the intact animal was separable temporally from glucose uptake and occurred at plasma insulin concentrations having no measurable influence on uptake of glucose in vivo . Different receptor mechanisms for potassium and glucose transport appear to exist.
In vitro , insulin is known to stimulate both potassium uptake and sodium efflux in frog and rat muscle preparations. Similar effects have been reported in rat adipose tissue, hepatocytes, and other cells. Considerable evidence suggests that following binding to cell surface receptors, insulin accelerates monovalent cation transport by stimulating Na,K-ATPase, the sodium-potassium pump. Most convincing is the fact that both insulin-stimulated net sodium efflux and potassium influx are blocked by ouabain. In vitro , addition of insulin to purified plasma membrane of skeletal muscle increases the activity of the Na,K-ATPase. Little evidence is currently available on whether insulin affects potassium permeability or potassium channels in skeletal muscle cells. Insulin activation of sodium–hydrogen exchange sensitive to amiloride does not appear to be important to sodium-pump-mediated potassium uptake. In adipocytes, insulin-stimulated uptake of K + and Rb + is inhibited by bumetanide and by removal of Cl − from the extracellular fluid, suggesting a primary action of insulin on the Na K 2Cl co-transporter. On the other hand, in skeletal muscle, insulin does not appear to activate the co-transport of K + with Na + and Cl − . There is evidence, however, that the serum and glucocorticoid-inducible kinase SGK1 participates in the different signaling pathways that mediate the hypokalemic effect of insulin.
Stimulation of active sodium–potassium transport by Na,K-ATPase could be due to a de novo increase in the number of sodium pump sites or to allosteric activation of existing sites. The latter theory is consistent with the rapid activation of transport that occurs, as well as with the lack of new ouabain-binding sites after exposure to insulin in vitro .
At least three molecular forms of the Na,K-ATPase catalytic subunit have been identified, designated alpha1, alpha2, and alpha3. In both rat and human skeletal muscles, insulin mediates the Na,K-ATPase alpha1- and alpha2-subunit translocation into the plasma membrane via a phosphatidylinositol 3-kinase-dependent mechanism. In liver, the effect of insulin can be accounted for by increased intracellular sodium concentration. Insulin also activates a KCl co-transporter uptake system in a cultured cell line resembling skeletal muscle.
It should be noted that insulin is known to produce hyperpolarization of cellular membranes, not only in skeletal muscle, but in a variety of other tissues. This rapid effect appears to precede measurable increases in intracellular potassium. Although stimulation of Na,K-ATPase could account for insulin’s hyperpolarizing effect, failure of ouabain to block it, at least in some studies, suggests that changes in ion permeability may be responsible. The role of hyperpolarization in mediating insulin effects on cation transport is uncertain. The effect of insulin to stimulate active sodium and potassium transport in skeletal muscle is mimicked by insulin-like growth factor I (IGF-I).
In Vivo Effects
Abundant evidence therefore exists that insulin increases net uptake of potassium ions by several tissues in vitro . Since skeletal muscle is well-documented to respond to insulin and is the major body reservoir for potassium, it is most likely the dominant site for insulin-stimulated extrarenal potassium disposal in vivo . Human forearm muscle (and adipose tissue) increases potassium uptake during arterial infusion of insulin.
Wilde noted over half-a-century ago that injected potassium rapidly disappeared from the blood of cats, but was followed by a secondary rise in serum potassium. Recent investigation suggests that, at least during insulin infusion, hepatic disposal plays an important role in potassium homeostasis in the first hour of exposure to insulin in humans. Splanchnic uptake accounted for two-thirds of the fall in plasma potassium during euglycemic hyperinsulinemia. In the second hour, net splanchnic uptake reversed, and peripheral tissues became the dominant site of potassium disposal.
That the effect of insulin on extrarenal potassium homeostasis is dose related is well-established ( Figure 48.3 ). In normal subjects, neither intramuscular nor subcutaneous administration of insulin, which achieved plasma insulin levels of about 50 µU/ml, decreased the plasma potassium. Intravenous insulin injection, by comparison, produced 40-fold greater insulin levels, which were accompanied by a steady-state reduction in plasma potassium, with a maximal effect of about 30% occurring 50 minutes after insulin injection. On the other hand, much smaller increments of insulin, about three-fold above basal values, either during constant venous or intra-arterial infusion also appear capable of augmenting potassium uptake in vivo . Under conditions of prolonged potassium depletion, however, the expression of skeletal muscle Na,K-ATPase α2 isoform is decreased, which allows for enhanced efflux of potassium from muscle to the extracellular space. Of particular interest is the observation that even modest dietary potassium restriction leads to a decrease in insulin-mediated cellular potassium uptake in rats in the absence of a fall in plasma potassium concentration.

Clinical Implications
The relevance of these findings to a given clinical situation of exogenous potassium challenge will depend on the magnitude of potassium-load requiring disposal, and the elevation of insulin accompanying it. Following carbohydrate feeding, for example, increased liver uptake of potassium occurs. Since peripheral venous insulin levels for 2 hours following oral glucose loading are elevated five-fold, well within the range capable of augmenting potassium uptake, it seems likely that insulin contributes to the transient decrease in potassium that occurs after feeding. Even basal circulating insulin levels may be essential to disposal of an acute potassium-load, since disposal is impaired when basal levels are decreased 50% by somatostatin. The effect of carbohydrate meals to blunt or prevent hyperkalemia may be particularly important in anuric patients dependent on extracorporeal dialysis.
During exogenous potassium challenge, the importance of insulin to potassium disposal by the intact organism deprived of endogenous insulin or resistant to the actions of insulin is well-established. As expected, supraphysiologic doses of exogenous insulin are capable of improving potassium tolerance. Thus, for emergency treatment of hyperkalemia, the intravenous administration of insulin together with glucose is indicated, unless the patient is already hyperglycemic from diabetes in which case additional glucose is not warranted.
The ability of potassium-loading to stimulate the release of pancreatic insulin directly, in amounts sufficient to contribute to disposal of that potassium, is less clear. In pancreatic B-cells, ATP-sensitive K channels link cellular membrane potential to hormone secretion. These channels control the transmembrane potential, and thereby the calcium channels that trigger glucose-induced insulin secretion. Depolarization of the cell membrane (as expected with hyperkalemia) induces an increase in insulin secretion. In humans and intact dogs, minor increments in blood potassium appear capable of triggering pancreatic insulin secretion. Since elevations in portal venous insulin far exceed those in the peripheral circulation when insulin release is stimulated, it seems reasonable to conclude that, under conditions of significant hyperkalemia, induction of insulin release to promote potassium uptake does constitute a homeostatic feedback control system.
Glucagon
The effect of glucagon on extrarenal potassium disposal has been difficult to isolate, because the hormone also influences the secretion of insulin, epinephrine, and aldosterone. Administration of glucagon to cats does appear to mobilize potassium from the liver and produce a transient rise in arterial potassium levels. In humans, the hyperkalemic response to the hormone appears to be only partly due to an epinephrine-like effect of glucagon to increase liver glycogenolysis.
For example, aortic injection of glucagon in humans, which results in hepatic vein glucagon levels within the pathophysiologic range, causes a transient increment in hepatic vein potassium concentrations, but this precedes the slow rise in glucose in hepatic venous blood. The specific source of the modest rise in hepatic venous potassium under these conditions has not been determined. Previous in vitro investigations using perfused rat liver suggest that glucagon releases potassium directly from the liver. The effect would not appear to be due to diminished Na K pump-mediated potassium uptake, since isolated rat hepatocytes exposed to glucagon actually undergo stimulation of this cation pump.
Systemic infusion of glucagon to physiologic levels tends to elevate plasma potassium slightly by an extrarenal mechanism, at least when glucagon-induced insulin secretion is suppressed by somatostatin, both in normal subjects and in diabetic subjects. It is unclear whether hyperglucagonemia, which occurs in decompensated diabetes mellitus, uremia, and exhausting exercise affect potassium homeostasis. In the dog, potassium-stimulated insulin release appears to be accompanied by a modest rise in circulating glucagon.
Catecholamines
The observation of D’Silva in 1934 that epinephrine lowers the serum potassium in cats has since acquired important physiologic and clinical relevance. Although D’Silva emphasized the rapid rise in the serum potassium that followed a bolus injection of epinephrine (now felt to be a consequence of transient hepatic discharge of potassium by alpha-adrenergic stimulation), of greater significance was the sustained “after-fall” in serum potassium that he observed. This secondary decrease in potassium was found to persist throughout a 1 hour infusion of epinephrine.
Since epinephrine inhibited the renal excretion of potassium, its late hypokalemic action was attributed to net uptake of potassium by extrarenal tissues. Indeed, in vivo limb studies in dogs and humans, as well as tissue analysis and ion flux studies, supported accelerated uptake of potassium, primarily in skeletal muscle, but also in liver and heart, in response to epinephrine. In vitro , epinephrine was demonstrated to stimulate potassium uptake, as well as sodium efflux, by isolated skeletal muscle in both rats and humans. A similar effect was present in rat diaphragm, cat cardiac muscle, and frog sartorius muscle.
Because epinephrine may influence insulin secretion, it was necessary to show that its hypokalemic effect was independent of insulin. Independence from renin-mediated aldosterone release was established by the lowering of potassium despite nephrectomy. Since extrarenal potassium disposal was impaired when nephrectomized rats were subjected to adrenalectomy or to chemical sympathectomy, both circulating adrenomedullary epinephrine, as well as peripheral sympathetic nervous activity, appeared to be important sources of sympathetic influence on potassium.
Beta-Adrenergic Effects
Epinephrine stimulates both alpha- and beta-adrenergic receptors. The conclusion that its hypokalemic action was a result of beta-adrenergic stimulation derived from experiments many years later employing beta-agonists and beta-antagonists. Isoproterenol, a nonspecific beta-agonist, reproduced the prolonged hypokalemia earlier observed by D’Silva, and this effect was reversed by the beta-adrenergic-antagonist propranolol; likewise, epinephrine’s effects on cation flux were blocked by beta-antagonists.
Beta-agonists and -antagonists have been used to establish that the stimulating effect of catecholamines on potassium uptake is mediated by the beta2-receptor subtype. Epinephrine’s effect on potassium is prevented by the presence of nonselective beta-antagonists such as propranolol and timolol, as well as by the nonspecific alpha-beta blocker labetalol. Less reversal occurs with the partially beta1-selective-antagonists metoprolol and atenolol. No effect on potassium appears to be produced by the more specific beta1-antagonist practolol. In addition, the selective beta2-antagonists butoxamine and ICI-118551 are able to block the hypokalemic action of beta-agonists.
Numerous studies of beta-agonists have revealed that several beta2-agonists, including salbutamol, terbutaline, fenoterol, and ritodrine lower potassium levels, unlike the beta1-agonist ITP, which has no effect. These pharmacologic studies therefore provide strong support for beta2 mediation of adrenergic effects to enhance potassium disposal.
Mechanism of Action
The specific cellular mechanism by which cell surface beta2-receptor stimulation augments transcellular potassium uptake in affected tissues has been evaluated in detail. Compelling evidence exists to support the proposal of Clausen that beta2-stimulation initiates cyclic AMP formation, which leads to activation of the sodium pump (Na,K-ATPase), and therefore electrogenic sodium efflux accompanied by potassium uptake. Beta receptors are known to stimulate adenylate cyclase, enhancing conversion of intracellular ATP to cyclic AMP. Linkage of beta receptors and cyclic AMP is supported by the ability of theophylline to potentiate the effect of epinephrine on cation transport and membrane potential, as well as by the increase in membrane potential that dibutyryl cyclic AMP and theophylline produce in rat diaphragm muscle. Epinephrine, in stimulating cation transport, is well known to produce hyperpolarization of membranes in skeletal muscle.
The sodium pump of muscle cells and lipocytes is activated by cyclic AMP. For example, Na,K-ATPase activity in smooth muscle membrane fragments is enhanced by exposure to cyclic AMP. The most compelling evidence that catecholamine-mediated potassium influx involves the beta-adrenergic system through activation of the sodium pump, however, are the demonstrations in a series of experiments that potassium influx occurs as active movement of the cation against its electrochemical gradient ; ouabain blocks the ability of epinephrine to promote potassium influx in rat soleus muscle ; epinephrine markedly increases the ouabain-sensitive 22Na efflux by stimulating the Na K pump in frog skeletal muscle, and this effect is blocked by propranolol ; epinephrine produces membrane hyperpolarization and transient decreases in extracellular potassium and intracellular sodium, which is blocked by ouabain, in isolated rat soleus muscle and human intercostal muscle ; and isoproterenol directly stimulates the Na K pump in isolated rabbit myocytes. While the specific sequence of events linking beta-adrenergic stimulation to sodium pump activity has not been delineated, phosphorylation of some portion of the sodium pump after beta2-receptor stimulation is presumably involved. Beta-adrenergic agents also stimulate the cellular uptake of potassium via the Na K 2Cl transporter in the skeletal muscle membrane of rats.
There are numerous clinical examples showing that enhanced exogenous or endogenous beta-adrenergic stimulation augments extrarenal potassium uptake in humans. Whether high potassium levels can stimulate secretion of endogenous adrenomedullary epinephrine, and thereby form a homeostatic feedback loop, remains unresolved. Supraphysiologic levels of potassium in vitro can cause the release of catecholamines from isolated chromaffin cells and perfused adrenal glands, possibly related to the membrane depolarizing effect of a high potassium level. Induction of tyrosine hydroxylase, the rate-limiting enzyme in catecholamine biosynthesis, by extremely high levels of potassium has also been observed. Reports that intra-arterial injections of KCl augment catecholamine release in cats and in the dogfish shark suggest a potential role for potassium as a catecholamine secretagogue in vivo .
Although specific stimulation of catecholamine release by potassium is not yet established, it is clear that physiologic elevations of endogenous catecholamines do enhance potassium uptake. Similar to the pharmacologic doses of epinephrine used in earlier animal reports of its protective effect during potassium-loading, as well as its ability to lower basal potassium, relatively small doses have also been shown to enhance extrarenal disposal of an acute potassium-load. It has also been established that ambient potassium may be lowered when sustained epinephrine infusions ( Figure 48.4 ) elevate plasma concentrations of epinephrine to levels no higher than those observed in stressful conditions, such as myocardial infarction, surgical stress, and diabetic ketoacidosis. By comparison, acute beta blockade does not appear to elevate fasting potassium levels, suggesting that basal beta-adrenergic tone plays a limited role in potassium homeostasis in normal fasted individuals at rest.

It has recently been appreciated, however, that there are two common physiologic circumstances in which endogenous catecholamines could act to defend against increments in extracellular potassium concentration. The first of these is postprandial disposal of dietary potassium. Feeding is now known to be associated with stimulation of the sympathetic nervous system. Since only half of the potassium ingested in a meal is normally excreted within 6 hours, enhanced beta-adrenergic-mediated extrarenal potassium disposal may help to limit elevations of serum potassium in the immediate postprandial period. In conjunction with enhanced potassium uptake due to insulin release, this mechanism would be particularly important in subjects at risk for hyperkalemia for any reason.
The second circumstance is the dramatic effect of catecholamine release during vigorous exertion to moderate the acute physiological hyperkalemia of exercise. Catecholamines circulate at high levels during vigorous exercise, and the associated short-term elevation of potassium that is released into the circulation from working muscles is exaggerated by beta blockade ( Figure 48.5 ), suggesting that endogenous beta-adrenergic activity does protect against extreme hyperkalemia during exhaustive exercise. In this context, it is of particular interest that training leads to upregulation of the content of the Na,K-ATPase, which serves to mitigate the rise in extracellular potassium concentration relative to the work performed. Another mechanism that might mitigate the rise in extracellular potassium seen during exercise is AMP-activated protein kinase. This cellular enzyme, which is normally stimulated by exercise or ischemia, has been demonstrated to produce a decrease in plasma potassium when stimulated chemically in the rat. This decrease does not appear, however, to be mediated by the Na,K-ATPase, and may instead be secondary to diminished efflux of potassium from the intracellular compartment.

Alpha-Adrenergic Effects
The fact that opposing alpha- and beta-adrenergic influences have in the past been reported on smooth muscle tone, glucoregulatory hormones, presynaptic membrane receptors, and changes in intracellular second messengers suggests the role of alpha-adrenergic agonists in potassium homeostasis. As noted previously, the early rise in extracellular potassium emphasized by D’Silva in 1934 was later attributed to alpha-mediated hepatic potassium release by the mixed alpha- and beta-agonist epinephrine. This initial rise in potassium could be prevented by alpha blockade. In addition, phenylephrine, a pure alpha-agonist, was observed to cause a sustained increase in potassium in dogs.
When phenylephrine was infused into normal human subjects who were challenged with an intravenous potassium-load, the overall rise in plasma potassium was augmented by about 50%, despite no change in insulin, renin, aldosterone or urinary potassium. In separate studies, addition of the alpha-antagonist phentolamine blocked the phenylephrine effect on potassium disposal. Neither alpha stimulation nor blockade appeared to affect the concentration of potassium in the absence of potassium-loading.
Other evidence suggests that the alpha effect might directly contribute to potassium homeostasis in certain circumstances. Alpha-receptor stimulation during vigorous exercise contributes to the acute rise in potassium that is maximal at peak exercise, and limits the dramatic fall due to potassium re-uptake during recovery. Furthermore, during potassium depletion in rats, the sodium–potassium pump of skeletal muscle is suppressed by an increase in alpha-adrenergic activity mediated by nerves, an action that would mitigate the expected fall in plasma potassium concentration. It is therefore speculated that enhanced alpha-agonist activity might act to preserve potassium similarly during a variety of acute illnesses, such as myocardial infarction or delirium tremens, where catecholamine stimulation of both beta- and alpha-receptors may coexist. Unopposed stimulation of alpha receptors may contribute to the impairment of potassium disposal caused by beta-receptor blockade.
Dopamine
The infusion of dopamine is known to augment glomerular filtration rate, renal plasma flow, osmolar clearance, sodium excretion, and potassium excretion in normal humans and animals. Levodopa, the metabolic precursor of dopamine, has also been reported to enhance renal potassium excretion, and an increase in endogenous dopamine produced by protein feeding has been associated with augmented kaliuresis. On the other hand, the dopamine antagonist metoclopramide also increases potassium excretion, an action ascribed to its blockade of tonic dopaminergic inhibition of aldosterone release. Whether the dopaminergic system plays a role in extrarenal potassium homeostasis remains uncertain. Both dopamine and dobutamine lower plasma potassium when infused into anesthetized dogs, but only by a few tenths of a mEq per liter. While some studies demonstrate enhanced extrarenal potassium uptake into cells following administration of metoclopramide, others demonstrate no such effect of either metoclopramide or the dopamine agonist bromocriptine. The discrepancy between these studies may well be attributed, at least in part, to the fact that metoclopramide is a nonspecific antagonist of dopamine. The determination of the role, if any, of the dopaminergic system in extrarenal potassium homeostasis must await studies employing specific dopamine antagonists.
Clinical Implications
The clinical significance of epinephrine-induced hypokalemia is underscored by reports of hypokalemia occurring during acute illnesses, such as myocardial infarction, as well as during medical treatment with beta-agonists. Struthers et al. discovered that when epinephrine infusion produced circulating epinephrine levels similar to those found after myocardial infarction, the serum potassium fell from 4.06 to 3.22 mEq/liter in normal volunteers. This ability of small doses of epinephrine to diminish potassium levels was confirmed by Brown et al. In this context, the frequency of hypokalemia during acute myocardial infarction has been observed to be 8 to 30%. Concomitant therapy with diuretics may further increase the frequency of hypokalemia during myocardial infarction, as it appears to do in normal subjects who are experimentally infused with epinephrine.
Because several studies have suggested that hypokalemia is an independent risk factor for cardiac arrhythmias in patients with acute myocardial infarction, these observations may have clinical relevance. A higher incidence of atrial fibrillation and ventricular arrhythmias are reported in hypokalemic compared with normokalemic patients during infarction. In one report, ventricular tachycardia or fibrillation increased three-fold (to 35%), and hospital mortality doubled in hypokalemic patients with myocardial infarction.
It must be appreciated, however, that the hypokalemia reported during earlier studies of epinephrine infusion occurred when high concentrations of plasma epinephrine were sustained by infusion. Whether elevations of plasma epinephrine during acute myocardial infarction are similarly sustained, rather than transient, is uncertain. The contribution of circulating epinephrine to the hypokalemia that may occur during this form of acute illness therefore remains hypothetical. Nonetheless, pharmacologic data do suggest that such acutely ill patients may be protected from the hypokalemia that is sometimes associated with myocardial infarction by the use of beta blockers. Whether the beneficial effect of beta blockade reported in this setting is due to diminution in hypokalemia-related arrhythmias is not known. That hypokalemia is not more prevalent in the setting of acute myocardial infarction may, in part, be a consequence of a simultaneous elevation in plasma norepinephrine, suggesting alpha-receptor stimulation that might antagonize the effect of epinephrine on potassium homeostasis.
Transient lowering of potassium levels has also been reported in other acute medical conditions in which catecholamines might stimulate beta-adrenergic receptors capable of increasing cellular uptake of potassium. Pharmacologic therapy employing beta2-agonists such as terbutaline, ritodrine, and salbutamol to prevent premature labor may produce a substantial degree of hypokalemia. Administration of salbutamol and albuterol in the treatment of bronchospasm may also produce unwanted hypokalemia. In this context, it is important to appreciate that administration of inhaled beta2-agonists to healthy volunteers in doses similar to those used by asthmatic patients during an acute attack has produced decrements of plasma potassium by as much as 0.9 mEq/liter. These observations raise the disturbing possibility that such usage of these agents may be the explanation for the high incidence of sudden death in adolescent asthmatics. Hypokalemia in this setting might be further exaggerated by the concomitant use of theophylline or other methylxanthines. Theophylline not only increases the level of circulatory catecholamines, but also enhances catechol stimulation of adenylate cyclase by blocking inhibitory adenosine receptors. Hypokalemia associated with elevated levels of epinephrine has also been reported in patients admitted for trauma, and in normal subjects who received endotoxin. Sepsis induced in rats, moreover, increases the activity of Na,K-ATPase.
The clinical significance of the adrenergic nervous system in potassium balance is also evident in the well-established effect of beta-antagonists to elevate extracellular potassium concentration. An example is the acute effect of propranolol to exaggerate the hyperkalemia of vigorous exercise. Some, but not all, investigators have reported a 10–15% increase in ambient plasma potassium during chronic beta blockade with nonselective agents. In addition, one study suggests that the serum potassium may rise above 6 mEq/liter in patients on nonselective beta-blockers at the time of open heart surgery. Transient hyperkalemia has been reported after cyclosporine administration in patients on beta-blocking agents, but not when these are omitted. Patients receiving succinylcholine anesthesia may also be at risk for the development of hyperkalemia. Patients with end-stage renal failure on dialysis, moreover, regularly exhibit an increase in predialysis potassium of about 1 mEq/L when treated with propranolol. Despite the clinical relevance of these studies describing an increase in extracellular potassium concentration in a variety of clinical conditions in patients receiving beta-blockers, it is important to realize that beta-blockers are unlikely to produce any significant increment in potassium concentration in subjects in whom the other mechanisms of potassium homeostasis are intact.
Since some studies do substantiate the anticipated absence of effect on potassium of cardioselective beta1-antagonists, selected groups of patients at risk for hyperkalemia might be protected from significant elevations in potassium due to beta blockade by the use of such selective agents. Such patients include those with diabetes mellitus, hypoaldosteronism, and renal failure.
Thyroid
Thyroid hormones enhance the activity of Na,K-ATPase in muscle cells, accelerating the extrarenal disposal of potassium. A portion of this effect can be ascribed to upregulation of beta-adrenergic receptors and an increase in beta-adrenergic responsiveness, but there appears to be a direct effect on cellular Na,K-ATPase as well. Hypokalemic paralysis occasionally complicates thyrotoxicosis; in such cases urinary excretion of potassium is always low, reinforcing the hypothesis that the low serum potassium reflects a shift of potassium from the extracellular to the intracellular compartment rather than urinary losses of potassium. The hypokalemia and paralysis respond to the administration of beta-adrenergic-blocking agents. This syndrome has been reported many times in Orientals, but is extremely rare in Caucasians and Blacks. It is not, moreover, associated with the inherited mutations in the calcium and sodium ion channels reported to cause familial hypokalemic periodic paralysis, but may in some cases be associated with a mutation in a channel mediating potassium efflux. Hyperkalemia has been reported in hypothyroid dogs after exercise, but not in humans with hypothyroidism.
Acid–Base
Although acid–base balance affects potassium excretion by the kidney, shifts of potassium in and out of body cells are the initial way in which acid–base disturbances alter serum potassium. These movements are influenced by a variety of factors, including the changes in extracellular and intracellular pH, the degree of cellular buffering, the nature of the anion accompanying H + , and the associated changes in hormonal and neural regulators of potassium uptake, including insulin and catecholamines.
The concentration of potassium in plasma tends to vary in the same direction as that of free hydrogen ions, so that acidosis promotes hyperkalemia and alkalosis promotes hypokalemia. Since approximately 40% of the acid buffering capacity of the body is provided by intracellular proteins, largely in muscle cells, and since potassium is the chief intracellular cation of muscle, the release of intracellular potassium is to be expected as a consequence of the intracellular buffering of hydrogen ions. Conversely, with repair of acidosis or during alkalosis, potassium will tend to move into cells. This exchange of K + for H + is not necessarily equimolar, and the routes across the plasma membrane that are responsible for such transfers have not been completely delineated. A simple reciprocal exchange of K + and H + via a single plasma membrane transporter has not been described in muscle cells.
Hydrogen Ion
The importance of pH in affecting internal potassium distribution during acidosis was demonstrated in early studies. Fenn and Cobb reported in 1934 that potassium exited from skeletal muscle in vitro when the bath pH was lowered, and moved into the tissue when blood at physiologic pH was substituted for acidic medium. They invoked the Donnan equilibrium to account for similarity in the intracellular to extracellular ratios of hydrogen and potassium ions, so that a decrease in that ratio produced by acidosis would be associated with a decrease in the ratio for potassium – that is, an increase in extracellular K + . In vivo as well, an approximately linear relationship appears to exist between the potassium gradient and the pH gradient across muscle cell membranes, as predicted by the Donnan effect. Nevertheless, the shifts in potassium observed in experimental situations are not always fully explained, either by the Donnan effect or by changes in membrane potential.
Since the ratio Ki/Ke for skeletal muscle is approximately 30, the maintenance of a similar hydrogen ion ratio between intracellular and extracellular fluid would require an intracellular pH of approximately 5.92. However, intracellular pH is actually in the range of 6.9–7.0, so that the intracellular to extracellular proton ratio is only approximately 3 rather than 30. The major reason for this is the operation of the amiloride-sensitive Na + H + antiporter, which transports H + out of cells by a secondary active mechanism that ultimately derives its energy from ATP via the Na,K-ATPase of plasma membranes.
Moreover, the direction of the movement of potassium during acute acid–base disorders is not uniform among various tissues. During acute respiratory acidosis, potassium moves out of the cells in muscle and liver, but into cardiac muscle. Yet, in both skeletal and cardiac muscle, the ratio of intracellular to extracellular hydrogen ion concentration falls. As will be seen subsequently, powerful evidence has been adduced under both in vitro and in vivo conditions suggesting that, in some circumstances, the ionic ratios of hydrogen and potassium can be dissociated and transmembrane fluxes of potassium change in the face of a stable pH gradient.
Inhibition of the sodium–potassium pump of plasma membranes by an acid pH contributes importantly to the relationship between acidosis and plasma potassium. In every case in which it has been measured, an increase in plasma potassium associated with systemic acidosis is accompanied by a fall in intracellular potassium. The optimal pH for mammalian Na,K-ATPase is 7.5–7.6, whereas intracellular pH of muscle is 6.9–7.2. The effect of pH on the activity of the sodium–potassium pump is exerted from the intracellular, rather than the extracellular, side of the membrane. In bladder epithelial cells, for example, a reduction of intracellular pH by 0.3 units, from pH 7.2 to 6.9, reduced pump activity to about 70% of that at pH 7.2. On the other hand, alkalinizing the cell interior to pH 7.5 led to a 35% increase in sodium-pump activity.
In addition to a direct effect of pH on the sodium–potassium transport enzyme, an indirect mechanism might involve the linked operation of the Na-H antiporter and the Na,K-ATPase. An attractive mechanism to explain the shift of potassium out of cells that occurs in acidosis involves the linked operation of these two transporters. Both of these transporters are present in the plasma membranes of most cells, including skeletal muscle. Acidification of the extracellular fluid would be expected to slow the rate at which hydrogen ions leave the cell and sodium ions enter, via the Na-H antiporter. The resultant decrease in intracellular sodium concentration would slow the Na-K pump, reduce active uptake of potassium by cells, and increase the concentration of potassium in extracellular fluid. A prediction of this hypothesis is that amiloride, which blocks the Na-H antiporter, would prevent the hyperkalemia produced by acidosis. It is not yet known whether this is the case, but amiloride does block the extracellular acidosis produced by KCl infusions.
Finally, intracellular acidosis appears to open ATP-sensitive potassium channels in skeletal muscle by reducing the degree of channel inhibition by ATP, an action that might accelerate efflux of K + from muscle cells during anoxia or extreme acidosis.
Mineral Acids
Acute mineral acidosis, produced by infusing HCl or NH 4 Cl, elevated plasma potassium by an average of 0.7 mEq/liter per 0.1 unit decrease in blood pH, in 14 different studies summarized by Adrogue and Madias. The results of these studies were extremely variable, however, ranging from 0.24 to 1.67 mEq/liter per 0.1 pH unit.
One reason for the variability in these reports is that the effect of mineral acid infusions on plasma potassium depends on the duration of the acidosis. Immediately following an acute acid infusion there is a marked fall in extracellular pH and bicarbonate, followed by a gradual rise over the next 2 hours or so, as tissue buffering and respiratory compensation moderate the initial changes in blood pH. Plasma potassium begins to rise early in the course of the infusion and increases progressively as buffering takes place, so that its concentration does not change in parallel with the change in pH. Late in the course of an acute acid-load, when cell buffers have been exhausted and intracellular pH drops sharply, plasma potassium rises more steeply.
It might be expected that the hyperkalemic response to an acid-load would be influenced by the state of muscle potassium. Experimental potassium depletion induced by DOCA (mean plasma K + 1.9 mEq/liter) did indeed attenuate the increase in plasma potassium produced by metabolic acidosis, but did not prevent it, and the percentage rise in plasma potassium from baseline induced by NH 4 Cl acid-loading was not significantly different from that of controls.
Clinical observations on the endogenous mineral acidosis of uremia, due to retention of sulfate and phosphate, suggest that the change in potassium that is observed when acidosis is corrected is of the magnitude expected in experimental mineral acidosis.
Organic Acids
That changes in acidity are not the sole determinant of the kalemic response to acidosis is indicated by the disparate effects which mineral acidosis and nonmineral acidosis have on potassium. In humans and animals, infusion of organic acids such as acetic, lactic or beta-hydroxybutyric acid produce far smaller elevations of potassium than hydrochloric acid. The prevailing hypothesis is that the anions of organic acids, either by readily penetrating the intracellular compartment, by entering cells as intact molecules or by being formed endogenously within cells, minimize the necessity for potassium cations to leave cells in exchange for hydrogen ions. The addition of HCl to rat diaphragms in vitro results in a loss of potassium from the muscle, whereas when extracellular pH is lowered by addition of acetic acid, beta-hydroxybutyric acid or lactic acid, no shift of potassium out of the cell is observed.
For similar degrees of clinical severity, less elevation of serum potassium occurs with endogenous organic acidosis encountered in ill patients than with mineral acidosis, although the correlation of pH and serum potassium in clinical states of organic acidosis is further complicated by prior total body potassium depletion, oliguric renal failure, hypercatabolism, and other factors. Ketone acids, for example, stimulate the secretion of insulin by the normal pancreas, while suppressing the secretion of glucagon. Infusions of hydrochloric acid, on the other hand, do not stimulate insulin secretion, but enhance plasma glucagon, which in turn tends to elevate the serum potassium. The sympathoadrenal system, which is strongly activated in most clinical states accompanied by organic acidosis (e.g., diabetic or alcoholic ketoacidosis, and the lactic acidosis of circulatory shock), plays an important role in minimizing hyperkalemia, because of the hypokalemic action of beta-adrenergic stimulation. Moderately severe potassium depletion (as occurs in diabetic ketoacidosis) itself attenuates the increase in plasma potassium induced by metabolic acidosis.
Untreated severe diabetic ketoacidosis is characterized by marked deficits (200–300 mEq) in total body potassium, owing primarily to coincident potassium losses from the gastrointestinal tract or kidneys. Despite these losses, hypokalemia is uncommon, present in only 4% of episodes in one series, probably because of concomitant acidosis. Serum potassium is usually normal or elevated at presentation, but falls during successful treatment. Hypokalemia during recovery from diabetic ketoacidosis is most prominent in patients who initially received sodium bicarbonate.
Since insulin plays a significant role in cellular shifts of potassium, lack of insulin is presumably important in promoting hyperkalemia during diabetic ketoacidosis, as it is in patients with hyperosmolar nonketotic coma, who may have severe hyperkalemia without acidosis.
Similar to uncontrolled diabetes, several factors in lactic acidosis complicate the effect of acidemia per se on serum potassium. These include volume-depletion, prerenal azotemia, hypercatabolism, concomitant diabetes, catecholamine effects, and external potassium imbalance. Although severe hyperkalemia is unusual in lactic acidosis, Perez et al. have pointed out the absence of detailed studies on potassium in this varied disorder. In experimental lactic acid infusion in animals, and in uncomplicated post-seizure lactic acidosis in humans, potassium does not increase with the appearance of acidosis. However, in other forms of lactic acidosis, such as those earlier encountered due to phenformin therapy in diabetes, and in other forms not associated with tissue hypoxia, mild elevation of potassium may occur. Renal insufficiency may be a contributing factor in such patients. Most patients with alcoholic ketoacidosis have a normal extracellular potassium concentration, although there is significant variability. The effects of rarer forms of organic acidosis on internal potassium homeostasis have been reviewed elsewhere in detail.
Respiratory Acidosis
The effect of acute respiratory acidosis in elevating plasma potassium is, in general, smaller in magnitude than that of metabolic acidosis, though some effect can usually be detected. A rise varying from 0.06 to 0.3 mEq K + /liter per 0.1 pH unit was detected in 22 studies of mild to moderate respiratory acidosis. More severe experimental acidosis, provoked by inhaled concentrations of CO 2 as high as 30%, induce more pronounced hyperkalemia. In anephric dogs, the initial effect on potassium is small, but after 2 hours respiratory acidosis may increase to the range observed in metabolic acidosis. On the other hand, in in vitro experiments, when the extracellular medium bathing isolated rat diaphragms was acidified by raising the ambient CO 2 from 2 to 10%, no shift in potassium was observed, consistent with the ability of carbonic acid to permeate cells, as do other organic acids. Chronic respiratory acidosis produced in dogs was not attended by hyperkalemia.
The increase in serum potassium induced by severe respiratory acidosis in intact rats could be roughly approximated from the decrease in (H + ) i /(H + ) o , and was attributed to the importance of the Donnan effect in producing these changes. In addition, it is very likely that sympathoadrenal stimulation plays a major role in modulating serum potassium during respiratory acidosis in vivo , since it is well-established that acute hypercapnia results in an intense sympathetic discharge and an increase in the plasma concentration of epinephrine. Glycogenolysis induced by epinephrine probably contributes to the initial release of glucose and potassium from the liver with hypercapnia, noted by Fenn and Asano, and the hypokalemic effect of beta-adrenergic stimulation is likely to blunt the movement of potassium out of skeletal muscle that would otherwise accompany acidosis. Probably for this reason, mild respiratory acidosis induced acutely in anesthetized patients was not found to elevate the plasma potassium, although beta-adrenergic blockade produced marked hyperkalemia in hypercapneic animals.
Bicarbonate
It has been suggested that the serum bicarbonate concentration, even at constant or “isohydric” pH, alters extrarenal potassium distribution and might account in part for the weak correlation between pH and serum potassium in many studies. Under conditions of constant blood pH, infusion of sodium bicarbonate appears to lower serum potassium, and during acidosis in rats, potassium may correlate better with serum bicarbonate than with blood pH. A similar correlation can be found in patients with hyperkalemia of diverse etiologies, as well as in experimental acute ammonium chloride acidosis. This has been taken to indicate that bicarbonate therapy, in addition to beneficially raising pH, corrects hyperkalemia directly, perhaps via intracellular transfer with the potassium cation.
Another explanation of the same data is that it is the quantity of acid buffered by cells, rather than the arterial pH, that governs the release of intracellular potassium. This would account for the fact that extracellular bicarbonate, rather than arterial pH, sometimes seems to exert an independent controlling influence on extracellular potassium. Effective buffering of an acid-load by intracellular and extracellular buffers, so as to produce a low serum bicarbonate and a normal arterial pH, involves the liberation of substantial amounts of potassium cations from intracellular proteins. It can be inferred from the foregoing that alkali treatment of hyperkalemia should be most effective when the plasma bicarbonate is low and acidosis marked, and less so when plasma bicarbonate and pH are normal; this is indeed the case. This result is also inherent in the finding that the changes in plasma potassium produced by metabolic alkalosis are smaller than those seen in metabolic acidosis.
Alkalosis
Metabolic and respiratory alkalosis are commonly associated with hypokalemia. In both, renal losses are important in initiating and perpetuating hypokalemia, but extrarenal adjustments are also involved. Acute alkalosis induced by infusions of sodium bicarbonate usually leads to a decrement in plasma potassium concentrations. The Δ(K)p/ΔpH slope is usually smaller than that commonly observed in acute mineral acidosis, although the reported range is wide, from −0.09 to −0.42 mEq/liter per 0.1 pH unit. The initial change is not attributable to kaliuresis. Acute respiratory alkalosis produces a comparable decrease in plasma potassium, although during voluntary overbreathing this fall may be counteracted by an increase in circulating norephinephrine which, as noted earlier, tends to increase plasma potassium. It should be pointed out that most studies of extrarenal effects of metabolic alkalosis have involved the infusion of hypertonic solutions of sodium bicarbonate. Since hypertonicity itself promotes a rise in plasma potassium, this would tend to counteract any hypokalemic effect of alkalosis per se .
An intuitively appealing explanation for the extrarenal effect of alkalosis is replacement of H + associated with cellular buffers by potassium, in the reverse of the sequence discussed earlier by which acidosis releases intracellular potassium ions. Enhanced exchange of intracellular H + for extracellular Na + via the amiloride-sensitive Na + H + antiporter would accelerate cellular potassium accumulation by stimulating the Na,K-ATPase pump. In addition, intracellular alkalosis probably stimulates the sodium–potassium pump directly, as discussed earlier.
The combined effects of respiratory alkalosis on renal and extrarenal potassium handling commonly produce mild hypokalemia (around 3.0 mEq/liter) resistant to potassium replacement in hypocapneic patients who are artificially ventilated. Extreme hypocapneic alkalosis and hypokalemia, as seen in recently intubated patients who are overventilated, may produce serious cardiac arrhythmias.
Aldosterone
In addition to its action on the kidney to increase potassium excretion, aldosterone enhances potassium secretion into intestinal fluids and saliva. Aldosterone-induced colonic potassium secretion appears to occur through increased expression of luminal BK channels. In chronic renal insufficiency, moreover, the colonic secretion of potassium is greatly enhanced. In that sense, its effect on serum potassium can be said to have an extrarenal component. Aldosterone also has an independent action to accelerate renal acid excretion, and the consequent alkalosis itself has a secondary effect on cellular uptake of potassium. Apart from these mechanisms, there is no convincing evidence for a direct action of aldosterone to increase potassium uptake by muscle cells. For example, incubation of isolated rat diaphragms with aldosterone results in a loss, rather than a gain, of intracellular potassium.
An extrarenal action of aldosterone has been suggested in the past, because of several lines of evidence. First, in experimental animals, hyperkalemia after adrenalectomy seemed not to be entirely accounted for by a positive balance of potassium. Furthermore, the fall in plasma potassium accompanying the administration of mineralocorticoids was not necessarily associated with an increase in potassium excretion. Interpretation of such data is complicated, because of the effect of adrenal medullary hormones on extrarenal potassium uptake, and the renal action of mineralocorticoids to retain sodium and excrete acid, resulting in expansion of extracellular fluid volume and alkalosis, both of which might secondarily lower serum potassium.
Long-term treatment of dogs by Young and Jackson with high doses of aldosterone altered the relationship between plasma potassium and total body exchangeable potassium, so that at any level of exchangeable potassium, plasma potassium was lower than in untreated animals. In these experiments, however, mean plasma bicarbonate was significantly higher in aldosterone-treated dogs than in controls, leaving open the possibility that aldosterone-induced alkalosis influenced potassium distribution.
Bia et al. gave intravenous potassium loads to adrenalectomized and nephrectomized rats, and found that the acute administration of aldosterone prior to infusing potassium blunted the hyperkalemia. The differences they observed, however, were small, and could have been completely accounted for by a modest increase in the potassium concentration of gastrointestinal secretions (e.g., an increase of 20 mEq/liter in 1 ml of intestinal fluid) caused by the known action of aldosterone to increase intestinal potassium secretion.
The role of mineralocorticoids in the disposition of an oral potassium-load was studied in anephric humans by Sugarman and Brown. Anephric patients were given 0.5 mEq/kg of KCl after 72 hours of either mineralocorticoid treatment (10 mg DOCA daily) or spironolactone (300 mg daily). The rise in serum potassium was delayed by DOCA, but the difference from control experiments with spironolactone was most marked at 1 hour, much less pronounced at 2 and 3 hours, and not present at 24 hours, suggesting that the mineralocorticoid had delayed absorption of potassium from the gastrointestinal tract. Chronic administration of high doses of mineralocorticoids is said to diminish serum potassium slightly in anuric patients, perhaps because of an increase in stool potassium.
Finally, Alexander and Levinsky reported that chronic potassium-loading improved extrarenal disposal of an acute potassium-load given after a night’s fast, an effect abolished by adrenalectomy and restored by chronic (but not acute) high-dose mineralocorticoid replacement. They postulated that chronic hyperaldosteronism acts directly by enhancing cellular uptake of an acute potassium-load. Another interpretation was offered by Spital and Sterns. These investigators showed that, when dietary potassium is withdrawn from rats previously fed a high-potassium diet, high rates of potassium excretion persist and “overshoot,” causing these animals to become progressively more depleted of potassium than controls. This “paradoxical potassium depletion” is responsible, at least in part, for extrarenal potassium adaptation, by creating a sink of potassium-hungry cells that avidly take up potassium, and thereby blunt the increment in plasma potassium after an acute potassium-load. Hyperaldosteronism magnifies urinary potassium losses during fasting, and thus promotes potassium depletion, which in turn facilitates the uptake into muscle of an acute potassium-load.
In summary, aldosterone and other mineralocorticoid hormones do accelerate the disposal of potassium, but probably not by a direct effect on muscle. The changes observed can be accounted for by the known actions of aldosterone on the renal and gastrointestinal transport of sodium and potassium, and the separate action of aldosterone to produce systemic alkalosis by enhancing acid excretion.
Renal Failure
In states of mild-to-moderate chronic renal failure, the ability to excrete potassium is well maintained by an adaptive increase in the rate of fractional potassium excretion to levels near the maximal for subjects with normal renal function. As renal function declines further, however, so does the ability to excrete potassium in a timely manner. With advanced renal failure, potassium is retained longer and dependence on extrarenal disposal becomes more critical.
Whether extrarenal potassium disposal is enhanced, normal or impaired in the setting of uremia remains controversial. Studies in which an oral potassium load was given found that patients with chronic renal failure excreted only one quarter to one-half the amount of potassium excreted by subjects with normal renal function over a comparable period of time. Nonetheless, an exaggerated rise in serum potassium did not always occur. Reports of impaired extrarenal disposal of an oral potassium-load in patients with chronic renal failure might be criticized, either because the patients had higher basal serum potassium concentrations or might have been more acidotic than the control subjects. Another study that concluded that extrarenal potassium disposal was impaired in patients with chronic renal failure involved chronic rather than acute potassium-loading. A more general criticism of studies administering oral potassium that might account for some of the discrepant conclusions, however, is the inherent difficulty of estimating the rate of gastrointestinal absorption of potassium in the setting of renal failure. With intravenous potassium-loading, however, most, but not all animal studies appear to show a discernible defect in extrarenal potassium disposal in the setting of renal failure. With rare exceptions, however, these studies examined extrarenal potassium homeostasis immediately after acute nephrectomy, rather than in the uremic state.
If extrarenal potassium disposal is impaired in uremia, it cannot be a consequence of increased cellular potassium content or high total body potassium, since both are normal or low in this state. Nor does there appear to be peripheral resistance to insulin-mediated potassium uptake, despite the known resistance to the action of insulin on glucose metabolism in renal failure. The chronic hyperinsulinemia of end-stage renal failure may afford some protection against hyperkalemia. Renin production, which might be expected to be suppressed in patients with end-stage renal disease, is normal or elevated in many patients. Furthermore, both hyperkalemia and salt restriction appear to stimulate aldosterone production adequately in the setting of chronic renal failure (in patients who do not have hyporeninemic hypoaldosteronism), and a lowering of the plasma potassium suppresses aldosterone secretion normally.
Catecholamines circulate at increased levels in most, but not all, patients with renal failure. While it is likely that much, if not all, of this increase is a consequence of decreased renal excretion, the pressor and pulse responses to norepinephrine infusion have been reported to be impaired in renal failure. Both intravenous and inhaled albuterol appear, however, to be effective and rapid therapeutic modalities to treat hyperkalemia in patients with advanced renal failure. If beta-adrenergic-mediated extrarenal potassium homeostasis is blunted in renal failure when compared with normal subjects, it may well be in those whose endogenous plasma epinephrine levels are chronically elevated or who are more acidemic. Contrarily, it should be appreciated that the administration of drugs that possess beta2-antagonist properties can produce significant hyperkalemia in patients with renal failure on dialysis.
Impaired extrarenal disposal of potassium in uremia might conceivably be caused by high circulating levels of parathyroid hormone. Infusion of parathyroid hormone appears to impair extrarenal disposition of a potassium-load in nephrectomized rats, an action ascribed to the enhancement of potassium efflux from cells produced by increasing intracellular calcium. Potassium tolerance in partially nephrectomized rats with chronic renal failure is improved by parathyroidectomy, and by administration of the calcium channel-blocker verapamil.
Metabolic acidosis can impair cellular potassium uptake (see “Acid–Base”), and alkalinization has been demonstrated to reverse this effect in anuric patients. Part of this effect of acidosis may be to diminish the activity of Na,K-ATPase, which has a pH optimum in the range of 7.5–7.6 in mammalian tissue.
That the activity of the Na-K pump is impaired in the erythrocytes of some uremic subjects has been well-established. The impairment is correlated with an increase in intracellular sodium of red blood cells. Also, the diminished pump activity is reversed when the red blood cells of uremic patients are incubated in normal plasma or when the patients are dialyzed, and the impairment is reproduced when normal erythrocytes are incubated in uremic plasma. These studies suggest the presence of a circulating inhibitor of the Na-K pump in some uremics. Other investigators have reported a decrease in the number of pump sites, estimated by ouabain-binding, rather than in the activity of the pump (defined as the ion turnover rate per pump site) in the red blood cells of certain uremic patients. Experimental uremia in rats decreases the Na,K-ATPase activity of skeletal muscle, an effect reproduced by incubating normal muscle cells with uremic serum. The excessive rise in plasma potassium exhibited by patients with chronic renal failure undergoing exercise is consistent, moreover, with an impairment in skeletal muscle Na,K-ATPase in humans with this condition, although a higher baseline potassium concentration or a more acidotic state can be confounding variables in such studies.
Magnesium
In a variety of clinical states, potassium-depletion accompanies magnesium-depletion. Among patients with hypokalemia, the coincidence of magnesium deficiency may range from less than 10% to over 40%. Clinical conditions with a high incidence of combined deficiencies in potassium and magnesium, such as diuretic administration, alcoholism, and diabetic ketoacidosis, generally involve a defect in renal conservation of potassium. Along with interest in the relationship between extrarenal magnesium and potassium balances has come information on the relationship of their movements in and out of cells.
Because potassium and magnesium are the principal intracellular cations, it is not surprising that an important physiologic relationship might exist between them. For each, reduced intracellular concentrations may exist in certain states of depletion, out of proportion to the reduction in serum levels. Furthermore, a high correlation is found between magnesium and potassium concentrations in extrarenal tissues, such as skeletal muscle. A deficiency of magnesium evokes potassium-depletion in animals and humans. In rats, however, serum potassium remains normal, whereas in human subjects, hypokalemia is often observed.
A specific effect of magnesium-depletion on potassium homeostasis can be deduced from experimental magnesium deficiency states in which magnesium deficiency is associated with renal potassium-wasting. One proposed mechanism is of importance to their internal homeostasis, insofar as it would involve a direct effect of magnesium depletion on the ability of both renal and extrarenal cells to preserve their potassium. It is known that experimental dietary magnesium depletion in rats results in loss of cellular potassium in cardiac as well as skeletal muscle. Furthermore, during magnesium depletion in both animals and humans, the intracellular deficiency of potassium cannot be restored by provision of potassium alone; correction of the magnesium deficiency is required. Clinical combined depletion may play a role in cardiac arrhythmias seen in patients with alcoholism or on diuretics, underscoring the importance of magnesium replacement in the correction of refractory hypokalemia and cellular potassium-depletion.
Two extrarenal mechanisms have been suggested: reduced Na,K-ATPase activity; and increased cell membrane permeability to potassium. Cellular potassium-depletion due to diminished active potassium uptake mediated by Na,K-ATPase might occur because this cation pump requires cellular magnesium. Animal studies have shown that magnesium-depletion is associated with a reduced concentration of Na K pump units in rat skeletal muscle. Reduced ouabain-binding, indicative of a decreased number of Na K pumps, has also been found in humans treated with diuretics who developed low muscle potassium concentrations associated with hypomagnesemia. Additional data suggest that magnesium-depletion may also exert its effect on intracellular potassium through an impairment of the activity of the sodium-potassium pump per se , rather than on the number of pump sites per cell.
The second proposed mechanism of potassium loss would involve effects of magnesium on membrane potassium channels. In mammalian heart cells and in a cultured insulin-secreting cell line investigated by the patch-clamp technique, physiologic concentrations of intracellular magnesium block outward current by inhibiting the opening of ATP-sensitive potassium channels. Magnesium may therefore play an important role in the low conductance of the outward potassium current through these channels under normal conditions. Such a direct effect of magnesium on potassium channels might result in cellular potassium-depletion during magnesium deficiency.
Drugs
Medications are the primary etiologic factor in as many as one-third of cases of clinically significant hyperkalemia, and are contributing factors in more than 60% of hyperkalemic episodes in hospitalized adults. Potassium supplements, angiotensin-converting enzyme inhibitors, angiotensin-II receptor-antagonists, potassium-sparing diuretics, trimethoprim, pentamidine, heparin, and prostaglandin-suppressing drugs that induce hyporeninemic hypoaldosteronism account for most of these cases; relative to those, hyperkalemia due to drugs that alter internal potassium distribution would appear to be uncommon. As is true for drug-induced hyperkalemia in general, the risk of significant hyperkalemia is substantially increased in patients with diabetes mellitus, renal insufficiency, hypoaldosteronism, and in the elderly. In most other cases, the rise in potassium is mild.
Impaired Extrarenal Disposal
Hyperkalemia due to beta-adrenergic-blockers, the most common class of medications that elevate potassium by extrarenal mechanisms, was discussed in an earlier section. A small, transient increase in serum potassium usually occurs in patients given depolarizing muscle relaxants, such as succinylcholine. Exaggerated increments, however, may occur in patients with central nervous system diseases, spinal cord injury, increased intracranial pressure, and a variety of other ailments. In such pathological states, the acetylcholine receptors may be increased beyond the junctional area. Because potassium efflux from muscle end plates occurs during normal depolarization, massive efflux of the cation from such sensitized muscle can account for the hyperkalemia noted in patients with neurologic motor deficits, tetanus or muscle damage. Succinylcholine-induced hyperkalemia may also be seen with neuroleptic malignant syndrome and skeletal muscle metastasis of a rhabdomyosarcoma. Why burn patients and those with intracranial lesions not involving upper motor neurons are also at risk is less apparent. In patients at risk, succinylcholine should be used with caution and in the smallest dose possible, and with pretreatment by nondepolarizing muscle relaxants.
Muscle cell breakdown may also result in hyperkalemia in patients who develop myositis due to the HMG-CoA reductase inhibitor, lovastatin. Such reactions are more likely when lovastatin is administered in combination with gemfibrozil, cyclosporine, and nicotinic acid. HMG-CoA reductase inhibitors are commonly used in patients already predisposed to hyperkalemia due to diabetes mellitus or renal insufficiency.
Arginine HCl, in doses used for the correction of metabolic alkalosis, may increase serum potassium. When this cationic amino acid is taken up by cells, potassium is displaced into the extracellular fluid. Hyperkalemia is more likely in patients with renal failure who are unable to excrete this endogenous potassium-load, and in those with liver failure who are unable to metabolize the administered arginine normally. The magnitude of potassium rise in normal subjects is under 1 mEq/liter ; it is not closely correlated with pH, and may occur in patients before correction of the alkalosis is accomplished. Lysine HCl may also increase serum potassium levels. The antifibrinolytic agent epsilon aminocaproic acid (Amicar) has been reported to cause hyperkalemia as well, although the mechanism underlying this effect is unclear.
Trivial increments in potassium may also occur at therapeutic or mildly toxic levels of cardiac glycosides. Blockade of Na,K-ATPase-mediated potassium uptake with normal doses of digitalis has minimal effect on the serum potassium, since glycoside binding to skeletal muscle, the major body reservoir of potassium, is limited. At toxic concentrations of digitalis, however, such as following massive overdose, marked hyperkalemia is well-described and indicates a poor prognosis. The toxic concentration of digitalis also prevents standard treatment of the hyperkalemia with calcium. Although no significant effect to retard potassium disposal in normal subjects with therapeutic digitalis levels has been published, it is likely that such an effect may exacerbate hyperkalemia in patients at risk. In patients with renal failure, for example, even modest digoxin toxicity resulting from therapeutic doses is reported to cause hyperkalemia. In fact, of drugs known to induce hyperkalemia, digoxin was the most frequently encountered among patients with hyperkalemic episodes, and nearly one quarter of these had toxic digoxin levels.
Nonsteroidal anti-inflammatory drugs produce hyperkalemia best attributed to their antikaliuretic effect, which results in positive potassium balance. No impairment of extrarenal potassium homeostasis has been directly demonstrated.
Lithium intoxication in animals is associated with a progressive elevation in serum potassium and electrocardiogram abnormalities characteristic of hyperkalemia. However, only at concentrations of plasma lithium (>10 mEq/liter) many times the therapeutic lithium levels (1 mEq/liter) attained in manic-depressive patients do these changes occur. Increments in serum potassium of under 1 mEq/liter have been reported during chronic lithium therapy, but frank hyperkalemia is rarely observed. Lithium may displace intracellular potassium from human red blood cells and from skeletal muscle. Amphotericin B (including the liposomal formulation) can cause severe hyperkalemia due to potassium released from the intracellular compartment when the dose is excessive, the rate of infusion is rapid or renal function is impaired.
Enhanced Extrarenal Disposal
Drug-induced lowering of serum potassium by exogenous insulin and beta-adrenergic-agonists was described in detail earlier. Increased circulating catecholamine levels, as well as enhancement of catechol stimulation of adenylate cyclase, also appear to mediate in part the effect of methylxanthine derivatives such as theophylline. Hypokalemia due to beta-adrenergic stimulation may occur with severe theophylline toxicity in humans. Propranolol is reported to block hypokalemia due to theophylline toxicity in the dog. Whether therapeutic levels of aminophylline are important in acutely lowering serum potassium in humans is unclear. Other methylxanthines, such as caffeine, might also be expected to decrease potassium concentrations, not only by stimulating release of catecholamines, but also by their blockade of high-affinity (A1) adenosine receptors, which normally act to inhibit adenylate cyclase.
Several clinical studies have reported minor decreases in serum potassium levels in patients treated with calcium-antagonists, sometimes associated with increased urinary potassium losses. Severe hypokalemia with heart block may complicate overdosage of verapamil. Enhanced extrarenal potassium disposal has been demonstrated with the calcium channel-blockers verapamil, nifedipine, and nitrendipine. In one study, pretreatment with either verapamil or nifedipine reduced by about 40% the increment in plasma potassium produced over 1 hour by the infusion of KCl in nephrectomized rats. The effect was not mediated by changes in pH, bicarbonate, insulin, aldosterone or the alpha- or beta-adrenergic systems. Diltiazem has been shown to reduce the interdialytic rate of increase in plasma potassium in patients with end-stage renal disease. Diminished calcium-mediated potassium efflux from cells may be responsible, since increased intracellular calcium enhances potassium permeability in vitro (the Gardos effect) in erythrocytes and other cells. While this action would lower plasma potassium, the net effect of calcium channel-blockers in clinical practice is complicated by the fact that these agents also inhibit secretion of aldosterone, which in turn would tend to produce potassium retention.
The increased use of thiopental infusions to achieve therapeutic barbiturate coma has been associated with extreme degrees of hypokalemia secondary to enhanced cellular uptake of potassium. Serious rebound hyperkalemia has ensued, moreover, following the administration of potassium to correct the hypokalemia and discontinuation of the thiopental. The mechanism responsible for the hypokalemia is unknown, but could involve inhibition of voltage-dependent neuronal potassium currents. Overdosage of the antimalarial chloroquine has also been associated with hypokalemia, presumably from enhanced cellular uptake, but such cases are often confounded by concomitant administration of other medications. High-dose intravenous methotrexate has been associated with the rapid development of tetraparesis and marked hypokalemia in one patient that appeared to be caused by enhanced extrarenal disposal of potassium.
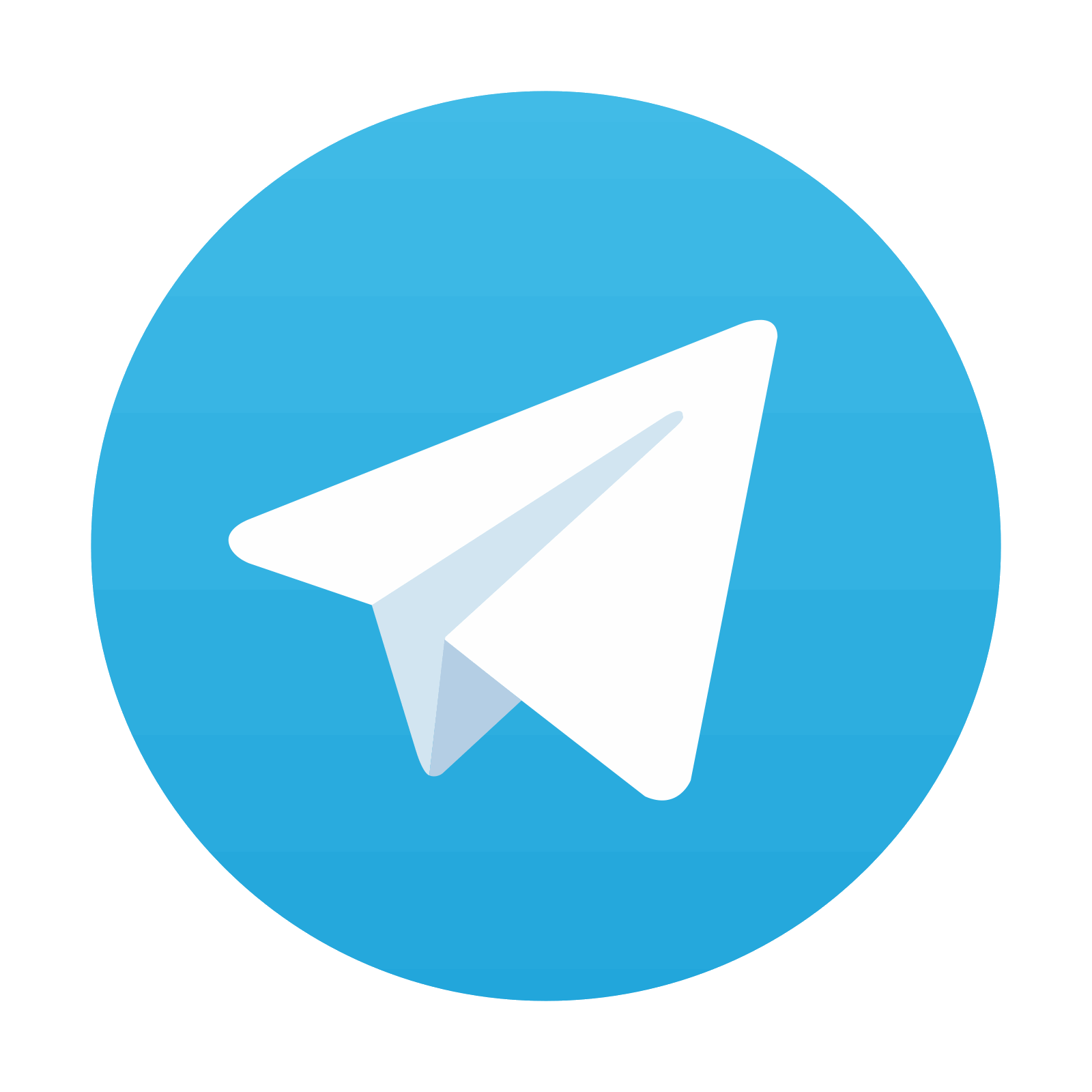
Stay updated, free articles. Join our Telegram channel
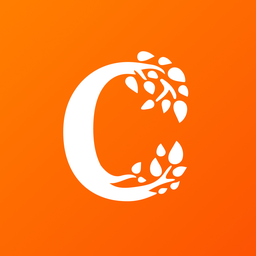
Full access? Get Clinical Tree
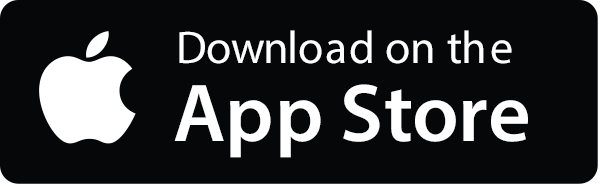
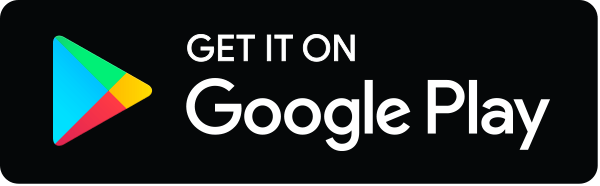