SOLUTE AND WATER TRANSPORT ACROSS A DIALYSIS MEMBRANE
The net clearance of any specific solute, or of water, from a patient’s blood at the end of a dialysis treatment is the sum total of a complex interplay between diffusive transport, convective transport, and adsorption, all of which are in turn determined by solute properties, membrane characteristics, solute concentration gradients, transmembrane pressure (TMP) gradients, blood and dialysate flow rates, ultrafiltration rate, patient’s hematocrit (Hct) and protein concentrations, and temperature. These processes are described in this section.
Solute Classification by Molecular Weight
A small molecular weight (SMW) solute is one whose molecular weight is less than 500 Da. Examples include most nitrogen-based solutes such as creatinine and urea, lactic acid, cholesterol, most vitamins (A, B1, B2, B3, B5, B6, B7, B9, C, D2, D3, E, K1, K2, K3), and all electrolytes (TABLE 1.2).
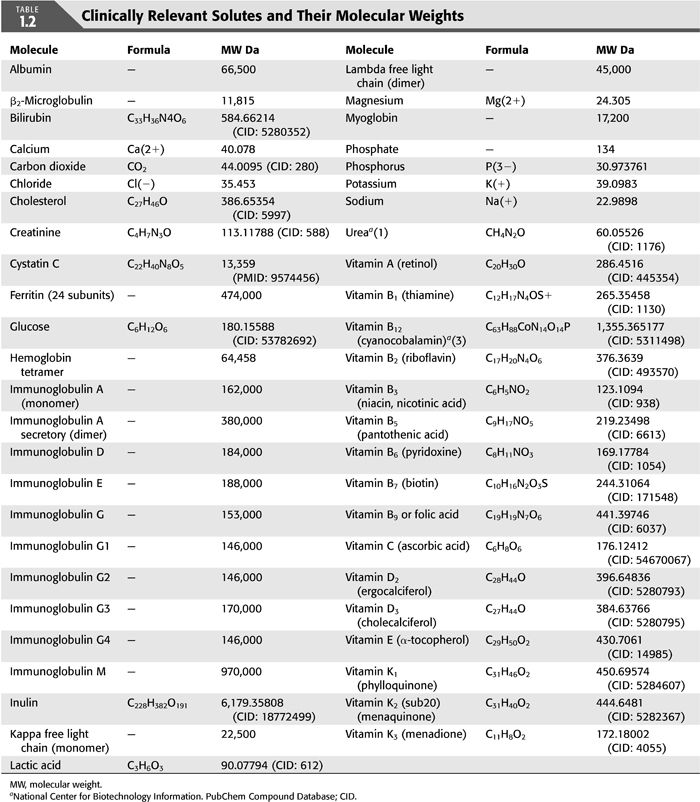
A middle molecular weight (MMW) solute is a solute with a molecular weight between 500 Da and 15 kDa. Examples include β2-microglobulin (β2-MG), cystatin C, vitamin B12, inulin, hemoglobin (Hb) and myoglobin, and bilirubin (TABLE 1.2).
A large molecular weight (LMW) solute has a molecular weight greater than 15 kDa. Examples include albumin, immunoglobulins, kappa and lambda free light chains, and ferritin (TABLE 1.2).
Diffusion
Diffusion is a mass transfer process that describes the transport of matter from one part of a system to another as a result of random molecular motion triggered by thermally induced agitation; in HD, such transport occurs between the blood and the dialysate across the semipermeable dialysis membrane from a region of high concentration to a region of low concentration. This is best represented by Fick’s first law of diffusion:

where JDiffusion (dn/dt) is flux in mol/s, D is diffusion constant in cm2/s, A is membrane surface area in cm2, ΔC is the concentration gradient in moles/cm3, and ΔX is thickness of the barrier to diffusion in cm. Since flux remains positive while the concentration gradient is dissipating, a minus sign is added to D.
For a specific dialysis membrane, D and ΔX are constant at any preset temperature, and thus, the above equation can simplified as follows:

where Ko is the overall mass transfer coefficient in cm/min. If rearranged, Ko = (JDiffusion / A) / −ΔC = flux per unit area / driving force of the concentration gradient; furthermore, this can be rewritten as (JDiffusion / A) = −ΔC/(1 / Ko) = −ΔC / Ro, which can be read as mass transfer per unit area = driving force / resistance to transport. For any specific solute to diffuse from the blood to the dialysate, the total resistance Ro would be the sum of the resistance to move from the bloodstream to the blood surface of the membrane RB, the resistance to move across the dialysis membrane RM, and the resistance to move from the dialysis side of the membrane to the dialysate RD: Ro = RB +RM + RD (3). RB can be minimized by the maximization of the blood-flow rate QB and shear rate, RD can be attenuated by increasing the dialysate flow rate QD, and RM can be optimized by an even packing density of the membrane fibers and spacer compounds during production.
In intermittent HD, diffusion is the major process for small solute clearance; is governed by concentration gradients; and depends mainly on membrane surface area and thickness, blood-flow rate, dialysate flow rate, and temperature (4). QB is usually slower than QD and as such has the greatest impact on diffusive solute clearance; at a QB of 400 mL/min, any increase in the QD above 600 mL/min will only offer a mediocre increase in diffusive solute clearance (5).
Convection
Convection is a mass transfer process that describes the bulk transport of molecules within fluids where transport is dependent upon factors such as changes in hydrostatic or osmotic pressure and membrane porosity; in HD, this is represented by a solute following the bulk transport of water (solvent drag phenomenon) during ultrafiltration triggered by a positive TMP. Said bulk transport follows Poiseuille’s law for hydrodynamic flow, which describes laminar flow of a Newtonian fluid through a pipe (e.g., hollow fiber); Newtonian fluids, such as water, have a constant viscosity in time and at different rates of shear stress.

where Q is the volume flow rate under laminar flow conditions, ΔP is the pressure drop across a pipe, r is the radius of the pipe, η is the viscosity coefficient (a constant that is dependent on temperature but independent of the speed of flow), and L is the length of the pipe.
However, blood does not obey Poiseuille’s law, owing to its being a non-Newtonian viscoelastic fluid with cells, which can deform, and proteins, both of which make its viscosity variable at different Hct and protein concentrations. Viscosity would be at its highest when the blood-flow rate and the shear rate applied are at their lowest (4). For blood flowing in a laminar fashion across a hollow-fiber dialysis membrane under preset condition that makes its viscosity constant, convection is reflected by the Hagen-Poiseuille equation:

where ΔP is the pressure gradient across the dialysis membrane, η is the blood viscosity, L is the length of the dialyzer membrane, QB is the blood-flow rate, N is the number of hollow fibers in a dialyzer, and r is the radius of the hollow fiber.
Thus, convective clearance across a dialysis membrane is better achieved with a higher pressure gradient ΔP along the length of the dialysis membrane, a higher blood viscosity η which is dependent on Hct, a higher blood-flow rate QB exceeding 300 mL/min, a smaller diameter of individual hollow fibers.
Convective clearance can be represented by the following equation:

where SC is the apparent solute sieving coefficient and QF is the ultrafiltration rate (6).
In HD, convective solute transport is driven by TMP and ultrafiltration (QF) and accentuates diffusive transport; convective transport gains greater clinical significance as the solute’s molecular weight increases (7) and is the cornerstone of therapies such as hemodiafiltration (HDF) (8).
Adsorption
Adsorption refers to solute removal via solute deposition, predominantly low molecular weight proteins (LMWPs), and adhesion to the dialysis membrane. An initial phase of competitive adsorption of high molecular weight proteins (HMWPs) to the inner layer of the dialysis membrane occurs until saturation is reached; this process results in a surface biofilm consistent of HMWP such as albumin, globulins, and fibrinogen. A second dynamic and slower phase of adsorption involves LMWP, such as β2-MG, cytokines, and anaphylatoxins, and involves the inner layers of the dialysis membrane and is completely dependent on membrane porosity and charge-dependent selective permeability (9). Synthetic membranes have a higher adsorption clearance than cellulosic membranes owing in part to their higher hydrophobicity and porosity; examples include polysulfone, polyacrylonitrile (PAN), and polymethylmethacrylate (PMMA) (10).
Clearance
The clearance of any specific solute is the sum total of its diffusive clearance, convective clearance, and adsorptive clearance. Clearance K = solute mass removal rate per unit time / plasma solute concentration. Based on prior discussion, solute clearance can be increased in the majority of instances by maximizing QB and QF.
Membrane Efficiency
Membrane efficiency refers to SMW solute permeability, most commonly, urea. It is the capacity of any one specific membrane to clear any one specific solute from the blood when other HD variables are preset, namely, blood-flow rate (QB) and dialysate flow rate (QD); urea has been traditionally the solute of choice to report efficiency in the dialysis membrane industry.
Efficiency is determined by a membrane’s surface area and its solute permeability and is quantitatively measured by the membrane permeability-area coefficient KoA, where Ko is the mass transfer coefficient and A is the membrane’s surface area (11). As mentioned earlier, most dialysis membrane manufacturers report the values for KoA measurements for urea when all other dialysis conditions are preset in vitro, namely, the QB, the QD, the ultrafiltration rate QF, dialysate temperature, and blood percent Hct. The KoA of a membrane indicates its urea clearance when QB and QD are infinite.
In vivo membrane efficiency may fall short of the in vitro performance due to blood-to-dialysate flow mismatch; for example, this may be caused by a higher blood viscosity on the luminal side of the membrane and thus affecting the blood-flow distribution, or to an uneven fiber density in a heterogeneously packed hollow-fiber membrane, thus affecting the dialysate flow distribution and resulting in a decreased dialysate flow velocity in the more densely packed areas, usually in the center, and a higher flow velocity in the less densely packed areas (4). Synthetic membrane fibers are usually distributed in an undulated or rippled pattern and thus result in a lesser blood-to-dialysate flow mismatch than the straight and unevenly packed fibers seen in cellulose membranes (12). It is also worth noting that the performance of many membranes is reported by the manufacturer based on an Hct of 32%, and higher in vivo values may also affect dialyzer efficiency (13,14). When in vitro experiments use a fluid other than blood, the manufacturer’s reported efficiency or KoA tends to overestimate the in vivo values; for instance, the Hemodialysis (HEMO) Study Group reported an in vivo KoA at 80 ± 7% (SD) of the in vitro values (p <0.001) in the 1,208 patients with arteriovenous accesses at first use and at a QD of 500 mL/min, and this further declined to 74.8 ± 6.6% when other variables were accounted for, namely, effects of QD and reuse number (11).
Synthetic and asymmetric membranes also tend to be more efficient due to a lesser resistance to water and solute transport conferred by their lower membrane thickness when compared to homogeneous and symmetric membranes (15).
Membrane Flux
Membrane flux refers to MMW solute permeability. This measure of permeability by molecular size cutoff is routinely reported in reference to a specific MMW or high molecular weight (HMW) solute, most commonly β2-MG and less commonly vitamin B12 or inulin.
Current dialysis membranes in order of permeability are high-cutoff membranes or super high-flux membranes > high-flux membranes > low-flux membranes.
Membrane flux has also been reported as a membrane’s capacity at ultrafiltration, or water flux, the major determinant of convective solute clearance across the membrane. The measure of the ultrafiltration capacity of a membrane, and thus its designation as low or high flux, is based on the ultrafiltration coefficient (KUF):

where QF is the ultrafiltration rate in mL/min, and PB − PD refers to the TMP gradient between the blood and the dialysis compartments. KUF is measured in vitro by the ultrafiltration of bovine blood at different TMPs (16). KUF is naturally dependent on membrane permeability to water, and thus, pore size is the major determinant of membrane flux; a membrane with larger pore sizes has the advantage of higher rates of water flux and a higher ultrafiltration capacity as well as a better removal of HMW solutes than membranes with smaller pore size.
Both parameters used to report membrane flux, MMW solute clearance and ultrafiltration capacity (KUF), correlate well as membrane performance measures (17).
Internal Filtration and Backfiltration
Internal filtration/backfiltration (IF/BF) is the phenomenon that describes the automatic and uncontrolled convective transport of solute with water from the dialysate compartment to the blood compartment when the driving force of filtration is dissipated under countercurrent flow conditions; this occurs when the oncotic pressure balances the hydrostatic pressure due to the high ultrafiltration rate characteristic of high-flux dialyzers (18). During a typical HD treatment with a high-flux membrane, the ongoing balance between hydrostatic and oncotic pressures along the membrane results in an ongoing process of IF/BF even when the ultrafiltration rate is nil; it is estimated that 6 to 8 L are internally filtered and backfiltered during a 4-hour treatment when no ultrafiltration is prescribed.
Theoretically, this IF/BF may result in the transport of bacterial byproducts or inflammatory mediators from a contaminated dialysate to the patient’s blood and result in bioincompatibility of the membrane; thus, it is recommended that ultrapure dialysate be used with high-flux HD to avoid potential proinflammatory effects (19).
This IF/BF phenomenon contributes to an automatic, uncontrolled form of convective solute transport, a convection-enhanced or IF-enhanced HD with its own internal hemodiafiltration (iHDF) component and without a replacement solution. IF/BF promotes MMW and LMW solute clearance, such as β2-MG, and LMWP clearance, in a process that is dependent on the IF flow rate QIF, which can be augmented by increasing QB (20–22). Several membrane developers have used this same phenomenon in the development of a newer generation of iHDF membranes that enhances the clearance of aforementioned solutes. A quantifying mathematical model has also been developed (23).
Dialysis Membrane Biocompatibility
Biocompatibility refers to the magnitude of the reaction of the human body upon exposure of blood to the dialysis membrane, triggering a cascade of events and generating a proinflammatory status, via activation of cellular and humoral immunity, and/or a thrombogenic effect. Thus, a hypothetically perfect biocompatible membrane would not trigger any human response (immune or thrombogenic or otherwise) and a bioincompatible membrane would mobilize all components of the immune and coagulation systems among others. For example, membranes made of the naturally abundant, unmodified cellulose polysaccharide (a D-glucose linear homopolymer (C6H10O5)n) (24) are among the least biocompatible membranes and, while at one point of time were the most widely used dialyzer membrane worldwide, have since been discontinued in 2006 and largely replaced by more biocompatible synthetic membranes, largely made of polysulfone (25).
CLASSIFICATION OF DIALYSIS MEMBRANES
There is no one classification of dialysis membranes by geometric design, chemical composition, efficiency, flux, or biocompatibility. With the continued development of newer membranes, there is a considerable overlap when considering all these criteria (TABLE 1.1).
Classification of Dialysis Membranes by Geometry, Design, and Structure
Coil Dialyzers have the highest resistance for solute mass transport from the blood to the dialysate (16) and are mostly of historical interest at this time.
Parallel-Plate Dialyzers have a lesser resistance for solute mass transport from the blood to the dialysate (16).
Hollow-Fiber Dialyzers have the lowest resistance for solute mass transport from the blood to the dialysate (16) and are the most widely used all over the world. Cross-sectionally, hollow-fiber dialyzers may be symmetric or asymmetric. Symmetric fibers are homogenous in cross-section with similar pore sizes across all layers. Asymmetric fibers are designed with a thin inner layer on the blood side of the membrane, which is the major determinant for solute permeability, and a thick outer supporting layer (the stroma) with larger pores toward the dialysate side of the membrane. The internal distribution of the hollow fibers plays an important role in its performance, and as mentioned earlier, asymmetric design is associated with less blood-to-dialysate flow mismatch (12).
Classification of Dialysis Membranes by Chemical Composition/Material
Dialysis membranes may be made of unmodified or regenerated cellulose, synthetically modified cellulose (SMC), or synthetic polymers (TABLES 1.1 and 1.3). Polymer-based synthetic membranes became commercially available in the 1970s and have since dominated the dialysis membrane industry; they tend to have higher performance, are more biocompatible, and their availability in high flux made it possible for patients to have a shorter HD time without jeopardizing dialysis adequacy (26). It must be noted though, that a dissociation between solute and water flux for membranes of any specific composition makes any simplified model of flux/composition classification almost impossible.
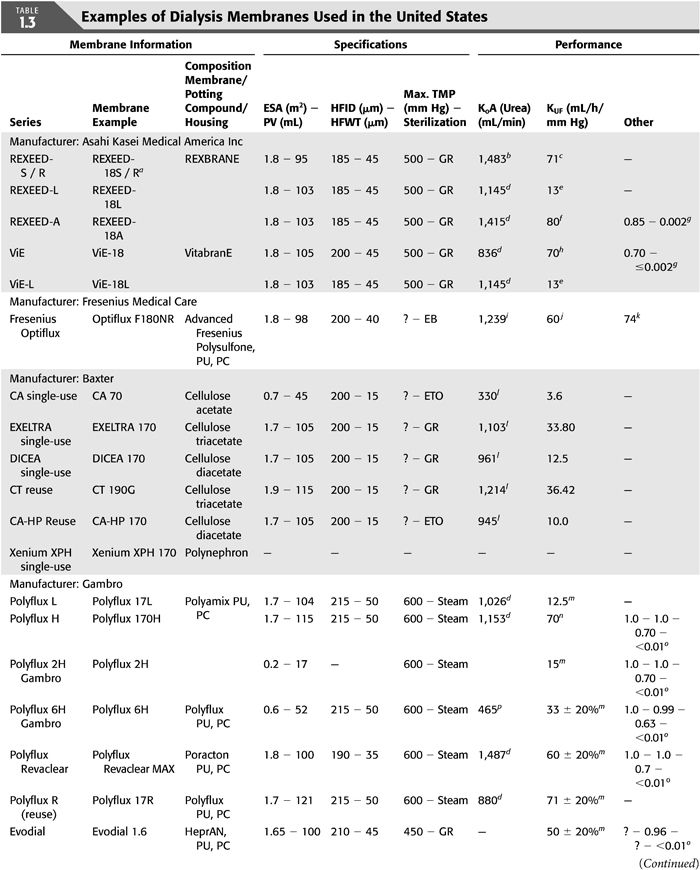
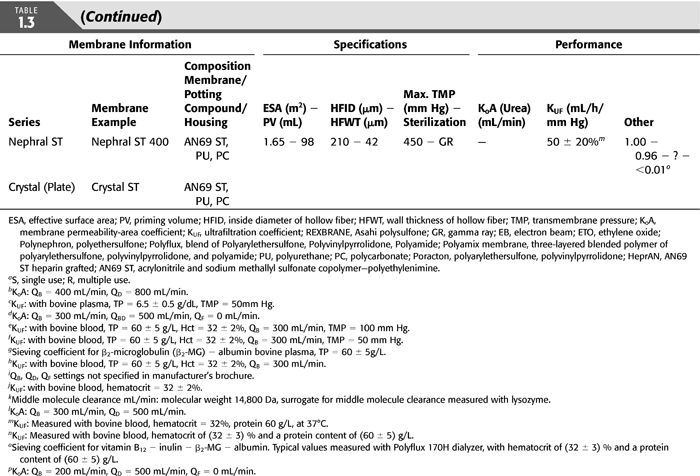
Unmodified or Standard Cellulose
The first described dialysis membrane was made of collodion, a polymer of cellobiose, an unmodified cellulose-trinitrate derivative (27); cellulose comprises 90% of cotton and 50% of wood and is as such the most abundant of all naturally occurring organic compounds. It is this low-flux membrane that Adolf Fick and Thomas Graham, among other scientists, used to lay out the principles of HD in the 19th century and early 20th century to selectively separate SMW solutes from blood through the process of diffusion (28). In 1914, a team of scientists led by Abel (29) developed and tested the first efficient in vivo dialysis system in a living animal at Johns Hopkins University School of Medicine, and in 1924, the first human HD was performed in a uremic patient by George Haas in 1924 at the University of Giessen in Germany (30,31).
Unmodified cellulose membranes are mechanically stable, have symmetric fibers, and are homogenous in cross-section with similar pore sizes across all layers; they are also hydrophilic enabling lower membrane thickness (6 to 15 μm dry thickness) (15,25), have small pores, and as such a good efficiency/performance on LMW or small solute diffusive clearance. However, they have a low flux with a KUF of <10 mL/h/mm Hg and perform poorly on middle-molecule clearance (molecular cutoff clearance of 5,000 Da; e.g., β2-MG); this, along with their bioincompatibility, driven by a high degree of alternative complement pathway activation as a result of complement protein interaction with the free cellulose hydroxyl moiety (25), eventually led to their replacement and the development of more biocompatible membranes with better clinical performance measures and clinical outcomes.
Regenerated Cellulose
Regenerated cellulose is obtained from cellulose that has been liquefied, purified, and extruded and changed by physical, rather than chemical, treatment such as cellophane, rayon, and viscose; their chemical structure remains identical to cellulose. One prototype dialysis membrane manufactured from regenerated cellulose is Cuprophan (CU, manufactured by Membrana); other examples include cellophane and cuprammonium rayon. In 1947, Cuprophan flat sheets were established as wrapping foil, and by 1965, the first flat sheet HD membranes were developed, but it was only in 1966 that Cuprophan was used in the world’s first industrially manufactured artificial kidney. Regenerated cellulose membranes replaced collodion because they offered better performance at the time and were more stable mechanically; they also had the same advantage of a low membrane thickness owing to their strong hydrophilic characteristics (15).
Modified Cellulose
Substituted Cellulose
This membrane is made by the chemical treatment of cellulose, for example, with acetic acid, where acetate replaces the free hydroxyl groups on the surface of the cellulose molecule. The replacement of the hydroxyl group decreases the availability of free nucleophilic moieties and is thought to reduce complement protein binding and activation and hence enhance biocompatibility (25).
A prototype of substituted cellulose membrane is cellulose triacetate (molecular formula C40H54O27; molecular weight: 966.84056 g/mol) (32) (e.g., CT190G, Baxter Healthcare Corp, Deerfield, IL) where there is a total replacement of all hydroxyl groups with acetate; other examples include cellulose acetate, cellulose diacetate, and cellulose hydrate. When replaced with acetate, there is an increase in membrane porosity and hence water and solute permeability; furthermore, the substituted cellulose triacetate membrane is highly hydrophobic and the absence of swelling decreases the membrane thickness (15,16). The cellulose triacetate dialysis membrane is the most biocompatible of all cellulosic membranes has a similar biocompatibility to that of the polysulfone membrane (16).
Semisynthetic Cellulose
This modification aims at replacing the free surface hydroxyl group with a bulky moiety that sterically hinders blood–membrane interactions. One prototype of modified semisynthetic membranes is Hemophan (manufactured by Membrana), in which a synthetic material, diethylaminoethyl (DEAE), is mixed with liquefied cellulose during its regeneration for membrane formation. This membrane retains the high-efficiency characteristics of the unmodified cellulose membrane and is available in low flux and high flux and has an affinity to bind anions, such as heparin and phosphate, and hence a decrease in thrombogenicity and phosphate clearance when compared to nonmodified cellulose membranes (33) but no change in phosphorus kinetics when compared to synthetic membranes (34).
Other examples of semisynthetic cellulose membranes include benzyl-modified cellulose (SMC, Membrana GmbH, Wuppertal, Germany), polyethylene glycol-grafted cellulose (AM-BIO, Asahi Medical Co, Ltd, Tokyo, Japan), and vitamin E (Excebrane, Asahi Medical Co, Ltd, Tokyo Japan).
Noncellulose Synthetic
Synthetic membranes are manufactured with a noncellulosic polymers but are more often than not alloys or copolymers of more than one polymer; this latter point is extremely important for the accurate interpretation of the physical and chemical properties of any synthetic membrane, especially that membrane nomenclature may be often misleading as it refers to only to one of the polymers used.
The first synthetic dialysis membrane was developed in the early 1970s and was a PAN membrane, namely, AN69 (Gambro, Lakewood, CO; Hospal, Meyzieu, France), an alloy of the acrylonitrile and sodium methallyl sulfonate polymers; at the time, the rationale was to develop a membrane with a high ultrafiltration coefficient for high-flux HD and hemofiltration (35).
Currently, the majority of dialysis membranes in use are synthetic. Of the synthetic membranes used, 93% are polymers from the polyarysulfone family with 71% being polysulfone and 22% being polyethersulfone (36).
Prototypes of noncellulose synthetic dialysis membrane include polysulfone (molecular formula: C27H26O6S; molecular weight: 478.55674 g/mol) (32) (e.g., F80A, Fresenius, Lexington, MA) which is the most widely used membrane, polyarylethersulfone (PAES) or polyethersulfone [molecular formula: (C12H8O3S)n; molecular weight: (232.258)n g/mol], polyacrylonitrile (PAN) [molecular formula: (C3H3N)n; molecular weight: (53.06262)n g/mol] (32) (e.g., AN69), polycarbonate [molecular formula: (CO3Ï2)n; molecular weight: (60.0089)n g/mol] (32), polyamide (molecular formula: C18H35N3O3; molecular weight: 341.4888 g/mol) (32), PMMA membranes [molecular formula: (C5H8O2)n; molecular weight: (100.11582)n g/mol] (32), and poly(vinyl alcohol-co-ethylene) (EVOH) (linear molecular formula: (CH2CH2)x[CH2CH(OH)]y). Synthetic membranes are thicker than cellulose membranes (35 μm or more dry thickness) (25), their fibers may be symmetric (AN69, PMMA) or asymmetric (polysulfone, polyamide) with various structures (sponge- or fingerlike) of the supportive stromal layer (16
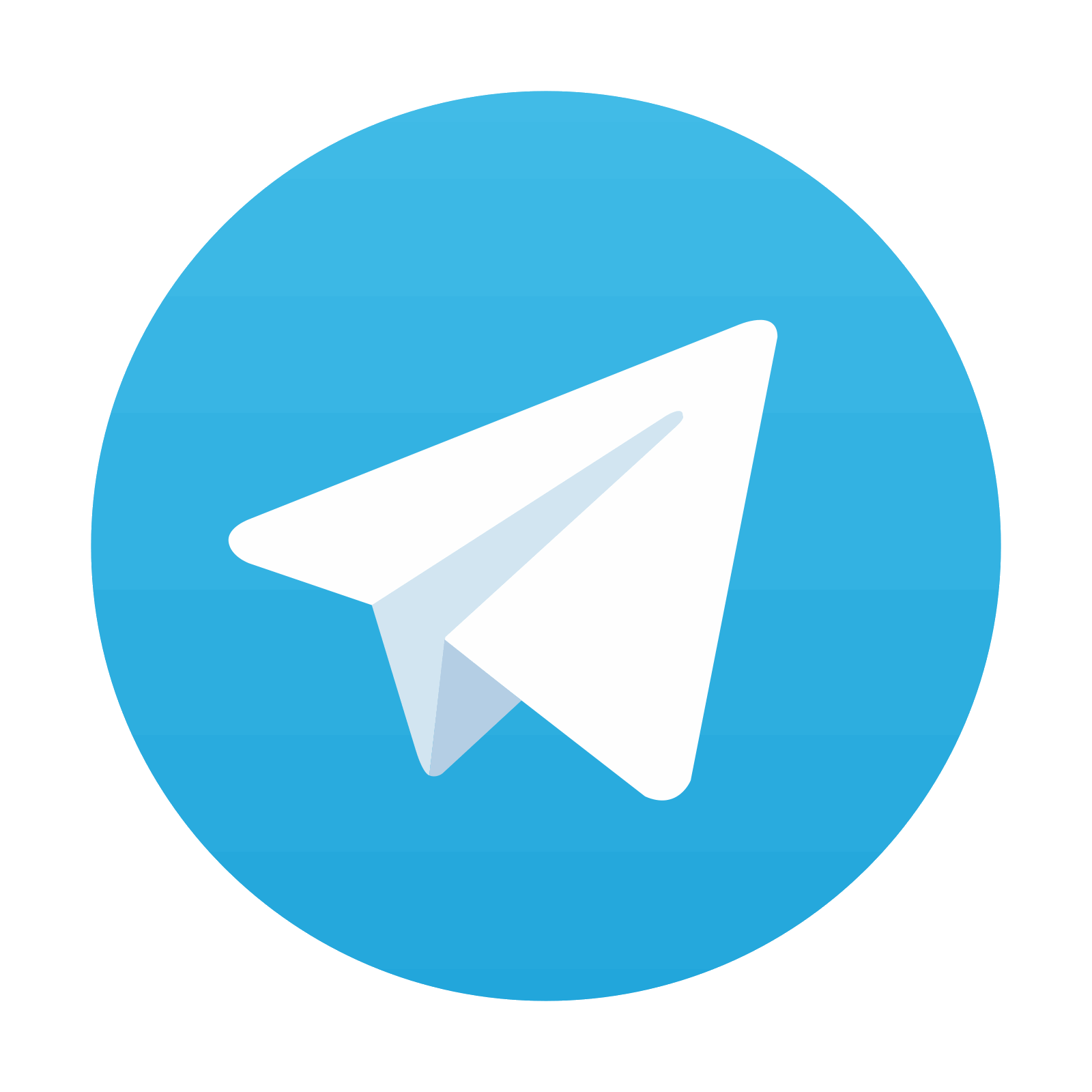
Stay updated, free articles. Join our Telegram channel
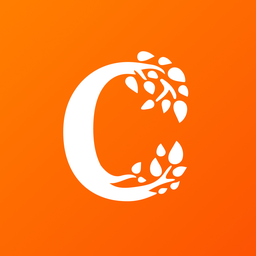
Full access? Get Clinical Tree
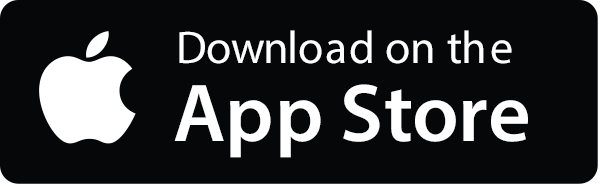
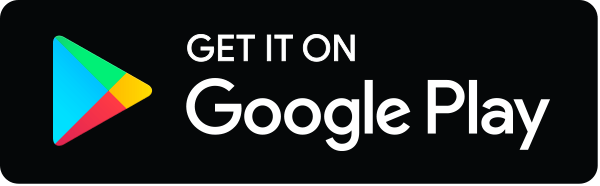