Hypernatremia can occur with normal, increased or decreased total body sodium content. In healthy individuals and in normal conditions, the plasma concentration of sodium ranges between 136 and 143 mEq/l of plasma, despite large individual variations in the intake of salt and water. The concentration is maintained at constant levels because of the homeostatic mechanism in the body. Claude Bernard was the first to appreciate that higher animals: “have really two environments: a milieu exterieur in which the organism is situated, and a milieu interieur in which the tissue elements live.” The latter is the extracellular fluid (ECF) that bathes the cells of the body. 1,2,3 Maintenance of this consistency of plasma sodium and solute activity is the function of the thirst–neurohypophyseal–renal axis. 4,5 Thirst and urinary concentration are the main defenses against hyperosmolality, and hence hypernatremia. Hypernatremia is a relatively common problem, with prevalence in hospitalized patients of 0.5 to 2%. It is defined as plasma Na + concentration ([Na + ]) greater than 145 mEq/l. It can be produced by the administration of hypertonic sodium solutions or in almost all cases, by the loss of free water. Since [Na + ] is an effective osmole, the increase in the plasma osmolality (P osm ) induced by hypernatremia creates an osmotic gradient that results in water movement out of the cells into the ECF. It is this cellular dehydration, particularly in the brain, that is primarily responsible for the neurologic symptoms associated with hypernatremia. A similar syndrome can be produced when the plasma osmolality is elevated by hyperglycemia. However, when hyperosmolality is due to the accumulation of cell-permeable solute, such as urea or ethanol, there is no water shift in the steady-state because osmotic equilibrium is reached by solute entering the cell.
Introduction
Water is the most abundant component of all cells, and the ability to absorb and release water is considered a fundamental process of life. Plasma membranes serve as selective barriers that control the solute composition of the cell and regulate the entry of ions, small uncharged solutes, and even water. Epithelial tissues have apical and basolateral plasma membranes that constitute serial barriers that regulate the transepithelial movement of solutes and water, thereby contributing to the homeostasis of multicellular organisms. Identification and characterization of the molecular entities responsible for the function of biologic membranes have been long-standing goals of physiologists; however, the molecular identity of water transporters remained unknown until less than two decades ago.
Because water can slowly diffuse through lipid bilayers, all biologic membranes exhibit some degree of water permeability. Nevertheless, observations made in multiple laboratories indicated that specialized membrane water-transport molecules must exist in tissues with distinctively high water permeability (see review ). For example, the water permeability of red cell membranes is higher than that of many other cell types or artificial lipid bilayers, and the activation energy of this process is equivalent to the diffusion of water in solution, E a <5 kcal/mol. In addition, reversible inhibition by HgCl 2 and a subset of organomercurials suggested that the water transporter is a membrane protein (see review ). Further evidence that a membrane protein is involved in water transport was provided by the observation that some epithelial tissues exhibit changes in water permeability on a timescale that is not compatible with changes in lipid composition.
Kidneys are the major determinant of body water and electrolyte composition. Thus, comprehending the mechanisms of water transport is essential to understanding mammalian kidney physiology and water balance. Because of its importance to human health, water permeability has been particularly well-characterized in the mammalian kidney (see review ). Approximately 180 L/day of glomerular filtrate is generated in an average adult human, the majority of this is reabsorbed by the highly water-permeable proximal tubules and descending thin limbs of Henle’s loop. The ascending thin limbs and thick limbs are relatively impermeable to water, and empty into renal distal tubules and ultimately into the collecting ducts. The collecting ducts are extremely important clinically in water-balance disorders, because they are the chief site of regulation of water reabsorption. Basal epithelial water permeability in collecting duct principal cells is low, but the water permeability can become exceedingly high when stimulated with vasopressin (also known as ADH, antidiuretic hormone). In this regard, the toad urinary bladder behaves like the collecting duct, and it has served as an important model of vasopressin-regulated water permeability. Stimulation of this epithelium with vasopressin produces an increase in water permeability in the apical membrane, which coincides with the redistribution of intracellular particles to the cell surface. These particles were believed to contain water channels.
Discovery of Aquaporin-1 (AQP1)
The molecular identity of membrane water channels long proved elusive. Attempts to purify water channel proteins from native tissues or to isolate water channel cDNAs by expression cloning, were unproductive (see review ). This may be explained by the physical characteristics of water, a simple molecule not amenable to chemical modification such as introduction of chemical cross-linking groups or labels. In addition, HgCl 2 was known to inhibit membrane water channels, a property potentially useful in the identification of the water channel proteins. However, because the agent reacts with free sulfhydryls in other proteins, its inhibitory effect on water channels is not specific. This circumstance led to the mistaken identification of the band 3 anion exchanger as a molecular water channel. In addition, the diffusional permeability of all biologic membranes results in high background permeability, and frustrated efforts to clone cDNAs for water channels by functional expression.
The recognized characteristics of membrane water channels led to chance identification of the first known water channel. In the process of isolating the 32 kDa bilayer-spanning polypeptide component of the red cell Rh blood group antigen, a 28 kDa polypeptide was partially co-purified. Initial studies demonstrated that the 28 kDa polypeptide comprised hydrophobic amino acids and exhibited an unusual detergent solubility, which facilitated purification and biochemical characterization. The 28 kDa polypeptide was found to exist as an oligomeric protein with physical dimensions of a tetramer; a unique N-terminal amino acid sequence was identified which permitted cDNA cloning. Also of note, radiation inactivation studies of water permeability by renal vesicles yielded a target size of 30 kDa. Because the 28 kDa polypeptide was found to be abundant in red cells, renal proximal tubules, and descending thin limbs, it was suggested that this protein might be the sought-after water channel. Although this protein was first known as “CHIP28” (channel-like integral protein of 28 kDa), the need for a functionally relevant name was recognized. The name “aquaporin” was coined. After recognition of related proteins with similar functions, this name was formally proposed for the emerging family of water channels now known as the aquaporins. Thus, CHIP28 was designated aquaporin-1 (symbol AQP1). The Human Genome Nomenclature Committee has embraced this nomenclature for all related proteins, and presently a total of 13 such related proteins have been identified in mammals.
The measurement of the movement of water across cell membranes poses a unique experimental challenge. Unlike ion conductances, which may be measured electrophysiologically, or solute transport, which may be measured with radioactive substrates, transmembrane water movement in cells relies on determination of changes in cell volume in response to an osmotic driving force. The Xenopus laevis oocyte expression system was used to search for water channel RNAs, because these cells are known to exhibit remarkably low membrane water permeability. Oocytes injected with cRNA encoding AQP1 exhibit remarkably high osmotic water permeability (P f ~200×10 −17 cm/s), causing the cells to swell rapidly and eventually rupture in hyposmotic buffer. In contrast, control oocytes not injected with AQP1 cRNA exhibited less than one tenth of this permeability.
Oocyte studies demonstrated that AQP1 behaves like the water channels in native cell membranes. Osmotically-induced swelling of oocytes expressing AQP1 occurs with a low activation energy, and is reversibly inhibited by HgCl 2 . Moreover, AQP1 oocytes fail to demonstrate any measurable increase in membrane current. Although these early studies demonstrated only swelling of oocytes, it was predicted that the direction of water flow through AQP1 is determined by the orientation of the osmotic gradient. Thus, AQP1 oocytes swell in hyposmolar buffers but shrink in hyperosmolar buffers.
To confirm that the interpretation of the oocyte studies was correct, highly purified AQP1 protein from human red cells was reconstituted with pure phospholipids into proteoliposomes, which were compared with simple vesicles (liposomes) by rapid transfer to hyperosmolar buffer. These studies permitted determination of the unit water permeability, which had an astonishingly high value (P f ~3×10 9 water molecules subunit −111 sec −111 ). Moreover, the water permeability is reversibly inhibited by HgCl 2 , and exhibits low activation energy. Several of these studies have been confirmed by using red cell membranes partially depleted of other proteins, and attempts to demonstrate permeation by other small solutes or even protons showed that AQP1 is water selective. Together, these studies indicated that AQP1 is both necessary and sufficient to explain the well-recognized membrane water permeability of the red cell, and suggested that AQP1 or similar proteins could be the long-sought-after epithelial water channels of the nephron and collecting ducts.
Structure and Function of Aquaporins
General Structure of AQP1
The availability of pure AQP1 protein in milligram quantities and the simple functional assay in oocytes led to rapid advances in the understanding of the molecular structure of AQP1. Hydropathy analysis of the deduced amino acid sequence of AQP1 predicted that the protein resides primarily within the lipid bilayer, a feature in agreement with initial studies of red cell AQP1. As previously described for the homolog major intrinsic protein from lens (MIP, now referred to as AQP0), the polypeptide contains an internal repeat ( Figure 41.1 ), with the N- and C-terminal halves being sequence-related, and each containing the signature motif Asn-Pro-Ala (NPA), suggesting ancestral gene duplication. When evaluated by hydropathy analysis, six bilayer-spanning domains, five connecting loops (A–E), and intracellular N-and C-termini are predicted. Attenuated total reflection-FTIR (Fourier Transform InfraRed spectroscopy) of highly-purified red cell AQP1 reconstituted into membrane crystals demonstrated a lack of beta structure in AQP1, indicating the existence of tilted alpha helices.

The two homologous domains equivalent to the N-and C-termini halves of the protein each consist of three transmembrane helices that are thought to be oppositely orientated in the lipid bilayer. A system was adapted for analyzing the structure of AQP1 after minimally perturbing the molecule by adding peptide epitopes at various sites. It was important that the epitope did not destroy function, and could be localized to intracellular or extracellular sites with antibodies and by selective proteolysis of intact membranes or inside-out membrane vesicles. These studies demonstrated that loop C resides at the extracellular surface of the oocytes and the intracellular location of loop D as well as the N-and C-termini. Moreover, the obverse symmetry of the N-and C-terminal halves of the molecule was confirmed ( Figure 41.1 ).
Loops B and E
Loops B and E encompass the two NPA motifs, and are the most conserved regions in the major intrinsic protein family. The loops exhibit significant hydrophobicity, suggesting association with the lipid bilayer. Subsequent studies implicated loops B and E as a structural component of the aqueous pathway. Experiments expressing AQP1 in Xenopus oocytes led to the observation that Cys 189 in loop E is the site of mercurial inhibition. Site-directed mutagenesis experiments in oocytes revealed that substitution of Cys 189 with a serine residue eliminates HgCl 2 sensitivity and increases osmotic water permeability, while substitution with larger amino acids residues prevents facilitated water transport. These results suggest that water transport and selectivity in AQP1 is somehow dependent on the steric properties of Cys 189 . In accordance with the internal repeat theory, Ala 73 located in loop B, the equivalent to Cys 189 in loop E, was investigated. By creating double mutants expressing Cys 189 as a serine and Ala 73 as a cysteine, the HgCl 2 sensitivity and osmotic water permeability were restored. These results suggested that loops B and E were arranged in a symmetrical fashion, and underlined that these loops were functionally essential for water permeability. This line of inquiry lead to the “hourglass” model, in which these domains overlap midway between the leaflets of the bilayer, creating a constitutively open, narrow aqueous pathway ( Figure 41.2 ).

Tetrameric Organization
Early biophysical characterization of AQP1 suggested that the protein formed multisubunit oligomers. Rotary and unidirectionally shadowed freeze-fracture electron microscopic analyses of AQP1 protein from human red cell membranes reconstituted in proteoliposomes provided detailed molecular insights. AQP1 had an oligomeric structure, consisting of four subunits surrounding a central depression. These tetrameric structures were also seen in highly water-permeable nephron segments expressing native AQP1. Although the oligomerization of AQP1 is still not understood in detail, all studies indicate that the protein is a tetramer composed of functionally-independent aqueous pores.
Structural Analyses and Molecular Dynamics of Aquaporins
By reconstituting the highly-purified red cell AQP1 into membranes under controlled conditions, membrane crystals were produced with AQP1 in highly uniform lattices. These membranes appeared as flat sheets or as large, resealed vesicles in which the AQP1 protein was found to fully retain water permeability. Thus, the opportunity to define the structure of AQP1 in a biologically-active state became possible. Electron microscopic studies by multiple groups permitted the elucidation of the protein at increasing levels of resolution. By performing high-resolution electron microscopic evaluation of negatively-stained membranes at a series of tilts, a three-dimensional view was obtained. Image projections revealed the presence of multiple bilayer-spanning domains, and atomic force microscopy further defined the orientation and extramembranous dimensions of AQP1. Electron crystallographic analysis of cryopreserved specimens has been undertaken at tilts of up to 60°. These analyses revealed the three-dimensional structure of AQP1 at increasing resolutions, down to 3.8 Å. X-ray crystallographic analysis has added further information about the structure of AQP1. Using the above method, the structure of AQP1 has been determined down to a resolution of 2.2 Å. In contrast to the previous studies, the high resolution enables observation of water molecules in transit through the channel. Based on combined efforts, a detailed picture of AQP1s tertiary structure could be formed.
As earlier studies indicated, the AQP1 monomers form a tetrameric cluster. The model shows an extension of the tetramer from the extracellular plane, while the intracellular surfaces form a shallow depression. The N-termini from one monomer is closely situated near the C-termini of the neighboring monomer. The gap formed by the four monomers narrows from 8.5 Å down to 3.5 Å. The residues surrounding the gap are hydrophobic, suggesting an interaction with a hydrophobic molecule. So, despite the monomeric formation of a central cavity, the tetrameric structure does not support the transport of water. This is in agreement with earlier observations, suggesting that each monomer is capable of facilitating water transport.
The dimensions of each monomer are 40 Å across and 60 Å long, as reported by Sui et al. ( Figure 41.3 ). The monomer is composed of six bilayer-spanning alpha helices surrounding a central density. The C loop located on the extracellular surface connects the N-and C-termini halves of the protein. Part of the central density represents the B and E loops, which appear as two short α-helix structures not permeating the membrane ( Figure 41.3 ). This organization is strikingly similar to the proposed hourglass arrangement of the B and E loops. The NPA containing loops are located on opposite sides of the membrane, juxtapositional to each other, interacting through their NPA motifs. The central density is composed of an extracellular and intracellular vestibule, separated by a narrow pore. The extracellular vestibule is approximately 15 Å at its widest. Following the extracellular vestibule towards the lipid bilayer, a decrease in diameter occurs and the pore becomes exceedingly small. The constriction site (also referred to as the aromatic/arginine constriction) is located 20 Å from the beginning of the extracellular vestibule, and is composed of several highly-conserved residues in conventional aquaporins. The constriction site is approximately 2.8 Å wide, about the diameter of a water molecule. After the constriction site the pore continues for 20 Å, a region termed the “selectivity filter”. The selectivity filter is slightly wider and part of the region is formed by the helical loops B and E. This locates the asparagine residues in the NPA motifs within the selectivity filter. Additionally, Cys 189 in loop E protrudes into the pore, confirming earlier studies, suggesting that HgCl 2 sensitivity was due to steric hindrance of water movement through the pore. Following the pore towards the intracellular vestibule, the diameter increases again, reaching 15 Å ( Figure 41.3 ).

Using molecular dynamic simulations, de Groot and Grubmüller obtained time-resolved, atomic resolution models of water transport through AQP1. From the extracellular vestibule, water molecules enter the constriction site, composed of side chains from the aromatic Phe 56 and His 180 , and the charged Arg 195 . This conformation situates the water molecule, allowing hydrogen bonding with the polar residues, thereby reducing hydrogen bonding between water molecules. In addition, electrostatic repulsion by Arg 195 suggests that the constriction site is the main site for exclusion of protons (hydronium ions) and other ions. This is further supported by mutagenic analysis of the residues in the constriction site, showing permeation of urea, glycerol, and ammonia in AQP1 when altered. Moreover, replacing Arg 195 with a valine residue appears to facilitate proton transport. Passing onward through the hydrophobic selectivity filter, exposed polar moieties mainly consisting of backbone carbonyls, lead the water molecules towards the NPA motifs. The water molecule transiently reorientates to bond with the two asparagines residues of the NPA motifs, and is led out of the selectivity filter towards the intracellular vestibule, again by hydrogen bonding with a few selected backbone carbonyls. Hence, the selectivity filter encompassing the highly-conserved NPA motifs appears to serve mainly as a filter for size, while the ar/R constriction is a major checkpoint for solute amd ion permeability. The Escherichia coli aquaglyceroporin (GlpF) selectively facilitates glycerol transport over that of water. Evaluation of differences between AQP1 and GlpF using molecular dynamic simulations revealed a larger selectivity filter in GlpF. Moreover, the preference for glycerol could be explained by what de Groot and Grubmüller describe as a glycerol-mediated “induced fit” gating motion (i.e., glycerol transport serves as the prime mechanism for water exclusion).
Structural characterizations of crystallized human AQP2 in two-dimensional protein–lipid arrays by atomic force microscopy and electron crystallography have revealed the structure of AQP2 at a resolution of 4.5 Å in the membrane plane. As with AQP1, AQP2 is found in a tetrameric assembly in the lipid bilayer. The AQP2 monomer shows structural features similar to those earlier reported for AQP1, while structural variation is found around the tetramer’s axis.
The structure of AQP0 has also been determined down to a resolution of 2.2 Å. The water conductance of AQP0 is much lower than that reported for AQP1, possibly due to highly-conserved tyrosine residues in AQP0 imposing further constriction on the channel. The constriction site is smaller than that in AQP1, and the selectivity filter is also narrower. Molecular dynamics studies suggest that water movement is facilitated by AQP0 due to thermal motions of certain side chains, although this builds up a free energy barrier, possibly contributing to the lower permeability of AQP0. The extensive analyses of the structure of various AQP isoforms have provided detailed insight into the molecular basis for transmembrane water transport. Future studies aimed at defining the distinct structure of other members, including aquaglyceroporins such as AQP7 and AQP9 that appear to be involved in metabolism rather than water transport may provide further insights. Several studies have also been aimed at identifying novel aquaporin blockers. Although some progress has been reported so far, only a few compounds, including related tetraammonium compounds, have shown selective effects on AQPs. Copper and nickel also significantly block aquaporins. Over the past five years the overall structural concepts of aquaporins have been confirmed.
Distribution of AQP1 in Kidney and Other Tissues
Well before recognition of its function, the red cell AQP1 protein was known to be expressed at high levels in the proximal convoluted tubules and descending thin limbs of kidney. This was confirmed with polyclonal rabbit antiserum, and was defined in rat and human kidney with affinity-purified immunoglobulin specific for the N- and C-terminal domains of AQP1. In all studies, AQP1 was demonstrated to be constitutively present in the apical plasma membranes (i.e., the brush borders), and in basolateral membranes of S2 and S3 segment proximal tubules ( Figure 41.4 ). Quantitative immunoblotting indicated that AQP1 makes up 0.9% of total membrane protein from rat renal cortex and 4% of brush border proteins. Enzyme-Linked Immunosorbent Assay (ELISA) measurements of microdissected tubules revealed that proximal tubules contain approximately 20 million copies of AQP1 per cell. Additionally, AQP1 is found in the plasma membrane of glomerular mesangial cells in humans, although not in rat. Other immunohistochemical and immunogold electron microscopic studies have demonstrated AQP1 in multiple capillary endothelia throughout the body, including the renal vasa recta. AQP1 is also abundant in peribronchiolar capillary endothelium, where expression is induced by glucocorticoids, apparently acting through the classic glucocorticoid response elements in the AQP1 gene. In addition, AQP1 has been defined in multiple water-permeable epithelia including choroid plexus, peritoneal mesothelial cells, fetal membranes, at multiple locations in eye, including ciliary epithelium, lens epithelium, and corneal endothelium, and in hepatobiliary epithelium, pancreatic interlobular ducts, heart and skeletal muscle, gall bladder, salivary glands, inner ear, and several other organs including the nervous system (see review ). AQP1 has also been localized in tumor cells and their vasculature. Developmental expression of AQP1 is complex: transient expression occurs in some tissues before birth; expression in other tissues is subsequent to birth; constitutive life-long expression is found in other tissues.

AQP1 Deficiency
Humans have been identified who totally lack the AQP1 protein. The human AQP1 gene was localized to chromosome 7p14 and the Co blood group antigens were previously linked to 7p, suggesting a molecular relationship. It was determined that the Co blood group antigen results from an Ala/Val polymorphism at the extracellular surface of red cell AQP1 ( Figure 41.1 ). Although the International Blood Group Referencing Laboratory in Bristol, England, has detailed phenotyping information on millions of donors worldwide, only six individuals had been shown to lack Co. Most of these Co-null individuals are women who developed anti-Co during pregnancy. Three of these Co-null individuals were found to have mutations in the AQP1 gene. Although the exceedingly rare blood group phenotype makes them impossible to match for blood transfusion, it was surprising that none of them exhibited any other obvious severe clinical phenotype. The extreme rarity of the Co-null state may reflect an important developmental role, resulting in reduced fetal survival; however, the frequency of the heterozygous state is unknown. Detailed studies of the urinary concentrating ability of Co-null individuals revealed a marked inability of the individuals to concentrate urine to more than 400 mOsm/l, even in the presence of dehydration or dDAVP treatment, revealing a significant urinary concentrating defect. Moreover, Co-null individuals display reduced pulmonary vascular permeability.
The development of AQP1 gene knockout mice has provided further insights into the role of AQP1 in renal water homeostasis. AQP1-null (AQP1 −/− ) mice appeared moderately polyuric under basal conditions. However, AQP1 −/− animals exhibited an extreme degree of polyuria and polydipsia when undergoing water deprivation, including rapid hyperosmolar extracellular fluid volume depletion. Additionally, AQP1 −/− mice failed to respond appropriately to vasopressin, suggesting that renal water conservation and urinary concentration is highly dependent on AQP1 protein. Detailed classic in vivo and in vitro physiological evaluation of proximal tubules from the AQP1 −/− mice established that transepithelial water permeability was reduced by 80%, and led to an approximate 50% decrease in proximal tubule fluid reabsorption. The apparent differences in transepithelial water permeability and proximal tubule fluid reabsorption are likely dependent on the generation of a hypotonic filtrate in proximal tubules of AQP1 −/− mice. Despite these observations, distal fluid delivery in AQP1 −/− remained unchanged, due to a compensatory reduction in single nephron glomerular filtration. When blunting the TGF response, and thereby the compensatory reduction in glomerular filtration observed in AQP1 −/− mice, ambient urine osmolalities and urinary flow rates appeared no different from normal AQP1 −/− mice, probably due to distal tubular compensatory mechanisms. The impaired proximal fluid reabsorption observed in AQP1 −/− mice, albeit with normal distal fluid delivery, suggests that the polyuric phenotype largely depends on a concentrating defect impairing collecting duct water reabsorption
Studies using isolated perfused thin descending limbs have revealed that the osmotic water permeability of the type II thin descending limbs (outer medullary thin descending limbs from long loop nephron) is decreased by 90% relative to wild-type values in kidneys from AQP1 knockout mice. Additionally, earlier observations using freeze-fracture electron microscopic techniques showed a remarkably high density of intramembrane particles in the thin descending limb of rat, which has been attributed to the tetrameric assembled AQP1 subunits. In the thin descending limb of AQP1 −/− mice, the abundance and size of these intramembrane particles was markedly reduced. Moreover, the distribution of AQP1 in the vasa recta suggests a role in microvascular exchange, hence affecting urinary concentration. In the presence of a NaCl gradient, osmotic water permeability was almost eliminated in the descending vasa recta of AQP1 −/− mice, leading to a predicted reduction in medullary interstitial osmolality and likely an impairment of countercurrent multiplication. Additional studies using adenoviral gene delivery restored AQP1 protein expression in the proximal tubule epithelia and renal microvessels of AQP1 −/− knockout mice, albeit not in the descending thin limbs. The adenovirus-treated mice showed slight restoration of the concentrating defect during water deprivation, probably due to reinsertion of AQP1 water channels in the vasa recta, although urinary concentrating ability was still highly insufficient in comparison to wild-type mice. In conclusion, the severe concentrating defect seen in the AQP1 −/− mice primarily results from impaired water absorption in the thin descending limb, underlining the necessity for a constitutively high water permeability in this segment for a functional countercurrent multiplication system.
Additional Functions of AQP1
AQP1 is generally believed to be a constitutively active, water-selective pore. Nonetheless, some observations contradict this. A small degree of permeation by glycerol has been seen in oocytes which may represent opening of an unidentified leak pathway, and the biologic significance remains unclear. Forskolin was reported to induce a cation current in AQP1-expressing oocytes, but multiple other scientific groups have failed to reproduce this effect. Although small changes in water permeability by oocytes expressing a bovine homolog of AQP1 have also been ascribed to vasopressin and atrial natriuretic peptide, the significance is uncertain. Likewise, secretin-induced membrane trafficking has been noted in isolated cholangiocytes ; however, this awaits confirmation by immunoelectron microscopy. Permeation of AQP1 by CO 2 has also been evaluated. Rates of pH change are about 40% higher in oocytes expressing AQP1 than in control oocytes. Although the background permeation of lipid bilayers by CO 2 , as well as oxygen, ammonia, nitric oxide, and other gases, may be high, the potential physiological relevance of AQP1 permeation by gases warrants more study (see review ). Additionally, AQP1 appears to play a role in cell migration. AQP1 −/− mice implanted with tumor cells show reduced tumor vascularity and growth, thus leading to improved survival in comparison to wild-type mice. Moreover, in vitro analysis of endothelial cells isolated from AQP1 −/− mice showed marked impairment in cell migration. In primary cultures of proximal tubule cells from AQP1 −/− mice, in vitro cell migration was impaired compared to AQP1 +/+ mice. Furthermore, in an ischemia–reperfusion model of acute renal tubular injury, AQP1 −/− mice showed more severe pathological changes, including more prominent tubule degeneration. Thus, although the evidence that AQP1 functions as a water channel is incontrovertible, the possibility of yet undiscovered transport functions cannot be excluded.
Aquaporins in Kidney
The first functional definition of one member of a protein family often prompts a search for related proteins. This has certainly been the case for the aquaporins, and the homology cloning approach has been undertaken by multiple laboratories whose combined efforts have expanded the aquaporin family membership list. Homology cloning has most frequently been undertaken by using polymerase chain amplification with degenerate oligonucleotide primers. Thirteen mammalian aquaporins are now known, and they form at least two subgroups: water-selective channels (orthodox aquaporins) and channels permeated by water, glycerol, and other small molecules (aquaglyceroporins). Given the large potential for confusion, the Human Genome Organization has established an Aquaporin Nomenclature System, accessible by internet ( http://www.gene.ucl.ac.uk/nomenclature ). Of the known aquaporins, eight are expressed in mammalian kidney ( Table 41.1 ). Soon after AQP1 was discovered to be a water channel, AQP2 was identified in renal collecting duct, where it is regulated by vasopressin and is involved in multiple clinical disorders ( Table 41.2 ). AQP3 was identified in kidney and other tissues, and was found to be permeated by glycerol and water. AQP4, a HgCl 2 -insensitive water channel is most abundantly expressed in brain, and is present in kidney collecting duct in the basolateral plasma membrane of principal and IMCD cells and in other tissues, but it is not inhibited by mercury. AQP6 was identified at the cDNA level and found to be localized in intracellular vesicles in the collecting duct intercalated cells in the kidney. AQP7 is permeated by water and glycerol. First cloned from testis, AQP7 is present in segment 3 proximal tubule brush border membranes, where it facilitates glycerol and water transport. AQP8 is a HgCl 2 -sensitive water channel found in intracellular domains of proximal tubule and collecting duct cells ; however, its function remains unclear. AQP11 is found in the cytoplasm of renal proximal tubule cells. The exact function is not established, although deletion of the AQP11 gene produces a severe phenotype with renal vacuolization and cyst formation.
AQP | Localization (renal) | Subcellular Distribution | Regulation | Localization (extrarenal) |
---|---|---|---|---|
AQP1 | S2, S3 segments of proximal tubules | Apical and basolateral plasma membranes | Glucocorticoids (peribronchiolar capillary endothelium) | Multiple tissues, including capillary endothelia, choroids plexus, ciliary and lens epithelium, etc. |
AQP2 | Collecting duct principal cells | Intracellular vesicles, apical plasma membrane | Vasopressin stimulates short-term exocytosis long-term biosynthesis | Epididymis |
AQP3 | Collecting duct principal cells | Basolateral plasma membrane | Vasopressin stimulates long-term biosynthesis | Multiple tissues, including airway basal epithelia, conjunctiva, colon |
AQP4 | Collecting duct principal cells | Basolateral plasma membrane | Dopamine, protein kinase C | Multiple tissues, including central nervous system astroglia, ependyma, airway surface epithelia |
AQP6 | Collecting duct intercalated | Intracellular vesicles | Rapidly gated | Unknown |
AQP7 | S3 proximal tubules | Apical plasma membrane | Insulin (adipose tissue) | Multiple tissues, including adipose tissue, testis, and heart |
AQP8 | Proximal tubule, collecting duct cells | Intracellular domains | Unknown | Multiple tissues, including gastronintestinal tract, testis, and airways |
AQP11 | Proximal tubule | Intracellular domains | Unknown | Multiple tissues, including liver, testes, and brain |
Congenital defects |
Central diabetes insipidus |
Mutation in gene encoding vasopressin (Brattleboro rat) |
Nephrogenic diabetes insipidus |
Mutations in gene encoding V 2 receptor (human, X-linked) |
Mutations in gene encoding aquaporin-2 (human, dominant and recessive) |
Partial concentration defects |
Targeted disruption of gene encoding aquaporin-1 (mouse) |
Targeted disruption of gene encoding aquaporin-4 (mouse) |
Acquired defects |
Lithium treatment |
Hypokalemia |
Hypercalcemia |
Postobstructive nephropathy, unilateral or bilateral |
Conditions with water retention |
Congestive heart failure |
Hepatic cirrhosis |
Nephritic syndrome |
Pregnancy |
Other conditions |
Syndrome of inappropriate vasopressin secretion |
Primary polydipsia |
Chronic renal failure |
Acute renal failure |
Low-protein diet |
Age-induced reduction in renal concentration |
Localization and Function of AQP2, AQP3, AQP4, AQP6, AQP7, AQP8, and AQP11 in Kidney
The amino acid sequences of the N-and C-termini of the different aquaporins are not closely related, and specific polyclonal antibodies can be raised in rabbits immunized with synthetic peptides conjugated to carrier proteins such as keyhole limpet hemocyanin. As with AQP1, these antibodies have permitted localization of the other aquaporins in kidney by immunocytochemistry, immunoelectron microscopy, and single tubule microdissection combined with ELISA ( Figure 41.5 ).

Three aquaporins (AQP2, AQP3, and AQP4) are expressed in the collecting duct principal cells and the connecting tubule segment, sites where vasopressin regulates epithelial water permeability. AQP2 is located in the apical plasma membrane and small intracellular vesicles of collecting duct principal cells ( Figure 41.6 ). ELISA measurements of microdissected rat collecting ducts have revealed that AQP2 is an extremely abundant protein in the collecting duct, with more than six million copies per cell throughout the collecting duct system. Additional studies using the single-tubule ELISA method and immunocytochemistry demonstrated co-localization of AQP2 and V2 vasopressin receptor expression on connecting tubule arcades, suggesting that regulated water transport may occur in the arcades of the cortical labyrinth in addition to the collecting duct. Additionally, AQP2 has been identified in the basolateral membranes of connecting tubules and inner medullary collecting ducts.

The apical plasma membrane is the rate-limiting barrier for transepithelial water transport across the collecting duct principal cell, and is the site where vasopressin regulates water permeability. Localization of AQP2 in the apical plasma membrane suggested that it is the target for vasopressin-regulated collecting duct water permeability ( Figure 41.6 ). This conclusion was firmly established by multiple studies. A direct correlation between AQP2 expression and collecting duct water permeability has been demonstrated in Brattleboro rats, which lack circulating vasopressin owing to a mutation in the gene encoding vasopressin precursor protein. Likewise, human patients with mutations in the AQP2 gene manifest severe vasopressin-resistant nephrogenic diabetes insipidus, demonstrating the requirement for AQP2 in collecting duct water transport. Lack of functional AQP2 expression in mice, by generation of AQP2 gene knockouts, produces a severe concentration defect, resulting in early postnatal death. Moreover, morphological changes including renal medullary atrophy and dilation of the collecting ducts is observed in these mice. Generation of AQP2 −/− knockouts selectively in the collecting ducts, but not in the connecting tubule segments, rescues mice from the lethal phenotype; however, body weight, urinary production, and the response to water deprivation is still severely impaired. Additionally, in a tamoxifen-inducible mouse model of AQP2 gene deletion, adult mice present with severe polyuria, a marked urinary concentration defect, and develop mild renal damage. Together these studies demonstrate the pivotal role of AQP2 in urinary concentration.
AQP3 is localized in a large variety of organs and highly-expressed in the kidney. AQP3 was originally cloned from rat kidney, and when expressed in Xenopus oocytes it facilitated glycerol transport (discussed below) and increased HgCl 2 -sensitive osmotic water permeability. Moreover, channel-gating is regulated by protons and copper. AQP4 was found to be expressed in several tissues with high abundance in the brain. In oocyte expression studies, the protein increased osmotic water permeability in a HgCl 2 -insensitive manner.
AQP3 and AQP4 are also present in the connecting tubule and collecting duct principal cells; however, the sites where they are expressed do not overlap with the expression of AQP2 in most portions of the collecting duct. AQP3 and AQP4 are restricted to the basolateral plasma membranes of collecting duct principal cells, where they are presumed to permit water entry into the interstitium. Although both are basolateral water channels, they are distributed differently along the collecting duct system, with the greatest abundance of AQP3 in cortical and outer medullary collecting ducts, and the greatest abundance of AQP4 in inner medullary collecting duct. AQP4 is also found in basolateral membranes of proximal tubule S3 segments, although only in mice. Using freeze-fracture electron microscopy, orthogonal arrays of intramembrane particles (OAP) were demonstrated in the basolateral membranes of the proximal tubule S3 segment in AQP4 +/+ , but not in AQP4 −/− , mice. AQP4 is expressed in two splice isoforms, the M1 and M23 splice variants. The M23-AQP4 appears to be the OAP-forming water channel when expressed in LLC-PK1 cells. When organized in OAPs, a significantly higher single-channel water permeability coefficient is observed in comparison to the non-AOP forming M1-AQP4. Shuttling of AQP3 or AQP4 is not expected to occur, as the predominant fraction is found in the plasma membrane (short- and long-term regulation of these aquaporins will be discussed later). Sodium restriction or aldosterone infusion in normal and Brattleboro rats greatly increases the abundance of AQP3, while AQP3 abundance is markedly reduced during aldosterone deficiency, suggesting a direct effect of aldosterone on collecting duct AQP3 expression. AQP4 appears to be regulated by PKC and dopamine, where stimulation by these factors decreases water permeability in AQP4 transfected cells.
Deletion of the AQP3 gene induces polyuria and polydipsia and a marked reduction in osmotic water permeability in the basolateral plasma membrane of the cortical collecting duct. Moreover, urinary osmolality is reduced in AQP3 −/− mice and they fail to respond appropriately to dDAVP, thus presenting with a urinary concentrating defect. Targeted disruption of AQP4 in mice results in a 75% reduction in the osmotic water permeability of the inner medullary collecting duct. However, phenotypically the AQP4 −/− mice appeared grossly normal, presenting with a very mild urinary concentrating defect. In double AQP3 −/− /AQP4 −/− -knockout mice, concentrating ability was only slightly more impaired than in the AQP3 −/− mice. It should be noted that the localization of AQP2 in basolateral membranes in both the connecting tubule and inner medullary collecting duct raises the possibility that the observed effect is partly compensated by this mechanism.
AQP6 vesicles reside in subapical vesicles within intercalated cells of the collecting duct, where it is co-expressed with the V-type H + -ATPase. AQP6 appears functionally distinct from other known aquaporins. Oocyte expression studies have revealed low water permeability of AQP6 during basal conditions, while in the presence of HgCl 2 a rapid increase in water permeability and ion conductance is observed. Additionally, reductions in pH (below 5.5) quickly and reversibly increase anion conductance and water permeability in AQP6 expressing oocytes. Subsequent studies have shown that the channel is permeable to halides, with the highest permeability to NO 3 − , while the ionic selectivity becomes less specific after the addition of HgCl 2 . Moreover, when Asn 60 , a residue unique in mammalian AQP6, is converted to glycine, a highly-conserved residue in other mammalian aquaporins, anionic permeability is abolished and osmotic water permeability is increased during basal conditions.
AQP7 is a member of the aquaglyceroporin family, and was originally cloned from rat testis. Additionally, AQP7 is expressed in several organs, including adipose tissue and kidney. In the kidney, AQP7 localizes to the brush border membrane of the proximal tubule S3 segment. Expression of AQP7 in Xenopus oocytes showed that AQP7 facilitated the transport of glycerol and urea in addition to water. The development of AQP7 knockout mice has aided understanding of the physiological role of AQP7. Glycerol excretion is markedly increased in AQP7 −/− mice, suggesting a role for AQP7 in facilitating proximal tubular glycerol transport, and possibly renal gluconeogenesis (discussed below). In terms of water transport, AQP7 −/− mice experience only a slight reduction in proximal tubule membrane water permeability, and AQP7/AQP1 double knockout mice showed only a slightly more severe urinary concentrating defect than AQP1 −/− mice during water deprivation. Additionally, evaluation of the channels’ permeability to urea in AQP7 −/− mice revealed no change in urinary urea excretion or urea accumulation in the papilla, indicating a less prominent physiological role in urea transport. Expression of AQP7 in Xenopus oocytes increases the permeability to arsenite, thereby providing a possible route for arsenite uptake in mammalian cells ; the physiological role of these observations awaits further investigation.
Cloning of the murine AQP8 water channel revealed its presence in many organs, including the kidney. In kidney, AQP8 localizes to intracellular domains of proximal tubule and collecting duct cells. Expression of AQP8 in Xenopus oocytes increased HgCl 2 -sensitive osmotic water permeability. Additionally, oocyte expression of AQP8 showed permeability to urea. Targeted deletion of the AQP8 gene produced a mild phenotype in AQP8 −/− mice, with no obvious changes in renal parameters. Subsequent studies have suggested that AQP8 facilitates NH 3 transport in Xenopus oocytes and in ammonia transport-deficient yeast. In AQP8 −/− mice, ammonia-loading mildly reduced hepatic ammonia accumulation and increased renal ammonia excretion, indicating that AQP8 does not play a significant physiological role in facilitated NH 3 transport.
Recently a new aquaporin was cloned from rat testis, namely AQP11. This AQP has only one NPA motif, and shares low similarity with the conventional aquaporins. AQP11 is most abundantly expressed in the testis, kidney, and liver. In the kidney, the AQP11 protein localizes to the cytoplasm of the renal proximal tubules. While lack of plasma membrane expression of the AQP11 protein in Xenopus oocytes greatly impaired measurements of transport, targeted deletion of the AQP11 gene in mice resulted in a severe phenotype with vacuolization and cyst formation in the proximal tubule. The AQP11 −/− mice died of renal failure and kidneys were polycystic. The cyst epithelia contained vacuoles, and the AQP11 −/− mice also presented with a proximal tubular endosomal acidification defect.
Aquaglyceroporins
AQP3, AQP7, and AQP9 constitute a subgroup of aquaporins with a broader permeation range that includes glycerol, hence the name “aquaglyceroporins.” AQP3, which transports water and glycerol, was cloned by three groups at the same time. The distribution at multiple sites, including the kidney collecting duct principal cells, airway epithelia, skin, urinary bladder, and secretory glands, suggests several functions. AQP3-null mice exhibit a nephrogenic diabetes insipidus phenotype; however, human mutants have not yet been reported. AQP7 is also permeated by water and glycerol, and is localized to the apical brush border of the proximal tubules, especially to the S3 segment. Recent studies have indicated that AQP7 may play a role in glycerol metabolism by showing adaptation to fasting by glycerol transport through AQP7 in adipose tissue. AQP7 −/− mice have a significantly lower plasma glycerol concentration than wild-type mice, and impaired adipocytic glycerol release in response to a β3 receptor stimulation. Moreover, AQP7 −/− mice experience severe hypoglycemia, but show normal hepatic gluconeogenesis during fasting, indicative of defective adipocytic glycerol transport in these mice. Glycerol excretion is also markedly increased in AQP7 −/− mice, suggesting a role for AQP7 in facilitating proximal tubular glycerol transport. AQP9, which is expressed in the liver but not in kidney, is permeated by a range of solutes including glycerol and urea. Recently, it has been demonstrated that expression of AQP9 in liver was induced up to 20-fold in rats fasted for 24–96 hours, and that AQP9 levels gradually declined after re-feeding. This indicates that the liver takes up glycerol for gluconeogenesis through AQP9 during starvation.
Vasopressin Regulation of Kidney Aquaporins
Of all the aquaporins, AQP2 has invoked the largest interest by nephrologists, because it is expressed in principal cells of the renal collecting duct, where it is the primary target for short-term regulation of collecting duct water permeability. AQP2 and AQP3 are also regulated either directly or indirectly by vasopressin through long-term effects that alter the abundance of these water-channel proteins in collecting duct cells.
Short- and Long-Term Regulation of Water Permeability in the Collecting Duct
Two modes of vasopressin-mediated regulation of water permeability have been identified in the renal collecting duct. Both involve regulation of the AQP2 water channel. Short-term regulation is the widely recognized process by which vasopressin rapidly increases water permeability of principal cells by binding to vasopressin V2-receptors at the basolateral membranes, a response measurable within 5 to 30 minutes after increasing peritubular vasopressin concentration. It is believed that vasopressin, acting through a cyclic adenosine monophosphate (cAMP) cascade, causes intracellular AQP2 vesicles to fuse with the apical plasma membrane, which increases the number of water channels in the apical plasma membrane ( Figures 41.6 and 41.7 ). Long-term regulation of collecting duct water permeability is seen when circulating vasopressin levels are increased for 24 hours or more, resulting in an increase in the maximal water permeability of the collecting duct epithelium. This response is a consequence of an increase in the abundance of AQP2 water channels per cell in the collecting duct, apparently due to increased transcription of the AQP2 gene ( Figure 41.7 ).

Short-Term Regulation of AQP2 by Vasopressin-Induced Trafficking
The final concentration of the urine depends on the medullary osmotic gradient built up by the loop of Henle, and the water permeability of the collecting ducts through the cortex and medulla (see review ). Collecting duct water permeability is regulated by vasopressin, and it has been suspected for many years on the basis of indirect biophysical evidence that the vasopressin-induced increase in water permeability depended on the appearance of specific water channels in the apical plasma membrane of the vasopressin-responsive cells. Molecular actions of vasopressin to increase epithelial water permeability were first demonstrated in the 1950s and early 1960s in the skin and urinary bladder of amphibia. Direct demonstrations that vasopressin rapidly increases water permeability of isolated collecting ducts were made during the next few years. These studies, undertaken with in vitro preparations, showed that vasopressin applied to the basolateral aspect of the collecting duct cells directly increased transepithelial osmotic water permeability within minutes. Kinetic studies in isolated perfused inner medullary collecting ducts revealed an increase in osmotic water permeability after only 40 seconds of incubation at 37°C, and half of the maximal water permeability was reached within 10 minutes. cAMP has been implicated in the regulatory process, because direct application of cAMP analogs to the collecting duct was found to mimic the short-term effects of vasopressin.
Multiple studies with affinity-purified polyclonal antibodies to AQP2 have unequivocally established that AQP2 is specifically involved in vasopressin-induced increases of renal collecting duct water permeability. Soon after isolation of the AQP2 cDNA and generation of specific antibodies, immunoperoxidase microscopy and immunoelectron microscopy clearly demonstrated that principal cells within renal collecting ducts contain abundant AQP2 in the apical plasma membranes and in subapical vesicles. These studies strongly supported the “shuttle hypothesis” originally proposed more than a decade earlier. This hypothesis proposed that water channels can shuttle between an intracellular reservoir in subapical vesicles and the apical plasma membrane, and that vasopressin alters water permeability by regulating the shuttling process. Shuttling of AQP2 was directly demonstrated in isolated perfused tubule studies. In these studies, water permeability of isolated perfused collecting ducts was measured before or after stimulation with vasopressin, and the tubules were fixed directly for immunoelectron microscopic examination ( Figure 41.6 ). Vasopressin stimulation resulted in a markedly decreased immunogold labeling of intracellular AQP2, accompanied by a five-fold increase in the appearance of AQP2 immunogold particles in the apical plasma membrane. This redistribution was associated with an increase in osmotic water permeability of similar magnitude. These findings were reproduced in vivo by injecting rats with vasopressin, which also caused redistribution of AQP2 to the apical plasma membrane of collecting duct principal cells. In contrast to the effect of vasopressin treatment, removal of vasopressin led to a reappearance of AQP2 in intracellular vesicles, and a decline in osmotic water permeability in the isolated perfused collecting duct system ( Figure 41.6 ). Moreover, the off-set response to vasopressin has been examined in vivo by acute treatment of rats with vasopressin-V 2 -receptor antagonist or acute water-loading (to reduce endogenous vasopressin levels ). These treatments (both reducing vasopressin action) resulted in a prominent internalization of AQP2 from the apical plasma membrane to intracellular subapical vesicles, further underscoring the role of AQP2 trafficking in the regulation of collecting duct water permeability and the reversibility of AQP2 trafficking to the plasma membrane.
The quantity of AQP2 in the apical plasma membrane is determined by the relative rates of exocytosis and endocytosis. Both processes occur continuously, and their relative rates are regulated to effect changes in water permeability. Kinetic evidence indicates that vasopressin regulates both endocytosis and exocytosis of apical water channels. These conclusions are supported by multiple earlier experiments with fluid-phase markers, which assessed rates of internalization of the apical plasma membrane. Intravenous injection of horseradish peroxidase was followed by uptake of the material into intracellular vesicles and multivesicular bodies by collecting duct principal cells. When compared with normal rats, the rate of uptake by vasopressin-deficient Brattleboro rats was much lower; however, this difference was lost after administration of vasopressin, presumably reflecting an effect of vasopressin in accelerating exocytosis, followed by a secondary increase in the rate of endocytosis to re-establish the steady-state. These observations were supported by studies with fluorescein-labeled dextrans, which confirmed that vasopressin enhances the rate of endocytosis of the apical plasma membrane under steady-state conditions. In apparent contrast, removal of vasopressin from isolated perfused rabbit cortical collecting ducts also was accompanied by increased endocytosis of horseradish peroxidase from the lumen, and it was suggested that water channels would also be internalized at an increased rate. Similar results were obtained in isolated rat inner medullary collecting duct, which showed that vasopressin washout was associated with increased appearance of albumin-gold or cationic ferritin in small apical vesicles and multivesicular bodies. Presumably this response reflects a direct effect of vasopressin to decrease the intrinsic rate of endocytosis and is reversed on vasopressin removal. Therefore, it appears that two factors affect the rate of endocytosis of the apical plasma membrane of collecting duct principal cells: (1) vasopressin directly decelerates endocytosis by unknown mechanisms; and (2) vasopressin indirectly accelerates the rate of endocytosis by stimulating exocytosis and increasing the total amount of apical plasma membrane. The latter effect is believed to be slower than the former, resulting in a biphasic decline in water permeability on vasopressin removal. Other studies have demonstrated that apical endocytosis is most rapid at about 3 to 5 minutes after vasopressin washout from isolated perfused inner medullary collecting ducts, corresponding to the period of the most rapid decrease in transepithelial water permeability. Thus, the results taken together strongly suggest that vasopressin directly regulates both endocytosis and exocytosis of AQP2. Presumably the net increase in water permeability seen in response to vasopressin is due both to direct stimulation of exocytosis and to direct inhibition of endocytosis.
Several groups have now successfully reconstituted the AQP2 delivery system using cultured cells transfected with either wild-type AQP2 or AQP2 tagged with a marker protein or a fluorescent protein. Using such cultured cells stably transfected with AQP2, it has been shown that AQP2 trafficking occurs from vesicles to the apical plasma membrane, albeit in some cases to the basolateral plasma membrane, as well as retrieval and subsequent trafficking back to the surface upon repeated stimulation. This recycling of AQP2 also occurs in LLC-PK1 cells in the continued presence of cycloheximide, preventing de novo AQP2 synthesis. Despite remaining uncertainties, a working model for collecting duct water permeability permits molecular insight into this process ( Figure 41.7 ). The short-term effects of vasopressin-enhanced water permeability are explained by the redistribution of AQP2 to the apical plasma membrane in collecting duct principal cells. Acting through the V2 receptor, vasopressin stimulates adenylyl cyclase; the elevated levels of intracellular cAMP activate protein kinase A (PKA), causing a redistribution of AQP2 from intracellular vesicles to the apical plasma membrane by inducing exocytosis of AQP2 vesicles, and by inhibiting endocytosis of AQP2 from the apical membrane. The net increase of AQP2 in the apical plasma membrane increases the water permeability. In the pre-antidiuretic state, the apical membrane is the rate-limiting barrier for transepithelial water transport, because AQP3 and AQP4 are abundant in the basolateral plasma membrane. Thus, increasing the water permeability of the apical plasma membrane by insertion of AQP2 water channels results in an increase in transepithelial osmotic water permeability. Whereas the general model is now well-established, the molecular details explaining how an elevation in intracellular cAMP levels regulates trafficking of AQP2 to the apical plasma membrane and back remain uncertain. Serine at position 256, in the carboxyl-terminal tail of AQP2, is apparently a substrate for protein kinase A, and regulation of exocytosis and endocytosis of AQP2 is expected to involve phosphorylation and dephosphorylation of AQP2, and of ancillary proteins involved in the trafficking processes. This question will likely be a major focus of future research.
Signal Transduction Pathways Involved in Vasopressin Regulation of AQP2 Trafficking
The signal transduction pathways have been described thoroughly in previous reviews. cAMP levels in collecting duct principal cells are increased by binding of vasopressin to V 2 -receptors. The synthesis of cAMP by adenylate cyclase is stimulated by a V 2 -receptor coupled heterotrimeric GTP-binding protein, G s . G s interconverts between an inactive GDP-bound form and an active GTP-bound form, and the vasopressin-V 2 -receptor complex catalyzes the exchange of GTP for bound GDP on the ∀-subunit of G s . This causes release of the ∀-subunit, G s ∀-GTP, which subsequently binds to adenylate cyclase, thereby increasing cAMP production. Protein kinase A is a multimeric protein which is activated by cAMP, and consists in its inactive state of two catalytic subunits and two regulatory subunits. When cAMP binds to the regulatory subunits, these dissociate from the catalytic subunits, resulting in activation of the kinase activity of the catalytic subunits.
AQP2 contains a consensus site for PKA phosphorylation (RRQS) in the cytoplasmic COOH terminus at Ser 256 . Recent studies using 32 P labeling or using an antibody specific for phosphorylated AQP2 showed a very rapid phosphorylation of AQP2 (within 1 minute) in response to vasopressin-treatment of slices of the kidney papilla. This is compatible with the time-course of vasopressin-stimulated water permeability of kidney collecting ducts. As described above, PKA-induced phosphorylation of AQP2 apparently does not change the water conductance of AQP2 significantly. Importantly, it was recently demonstrated that vasopressin or forskolin treatment failed to induce translocation of AQP2 when Ser 256 was substituted by an alanine (S256A) in contrast to a significant regulated trafficking of wild-type AQP2 in LLC-PK1 cells. A parallel study by Sasaki and colleagues also demonstrated the lack of cAMP-mediated exocytosis of mutated (S256A) AQP2 transfected into LLC-PK1 cells. Thus, these studies indicate a specific role of PKA-induced phosphorylation of AQP2 for regulated trafficking. To explore this further, an antibody was designed that exclusively recognizes AQP2 which is phosphorylated in the PKA consensus site (Ser256). In normal rats phosphorylated AQP2 (Ser256) is present in intracellular vesicles and apical plasma membrane, indicating that it is constitutively phosphorylated even in low circulating vasopressin states. In contrast, phosphorylated AQP2 is mainly located in intracellular vesicles in Brattleboro rats, as shown by immunocytochemistry and immunoblotting using membrane fractionation. Importantly, dDAVP treatment of Brattleboro rats caused a marked redistribution of phosphorylated AQP2 (Ser256) to the apical plasma membrane, which is in agreement with an important role of PKA phosphorylation in this trafficking. Conversely, treatment with V 2 -receptor antagonist induced a marked decrease in the abundance of phosphorylated AQP2 (Ser256), likely due to reduced PKA activity and/or increased dephosphorylation of AQP2, e.g., by increased phosphatase activity. Moreover, the necessity for the Ser 256 phosphorylation in AQP2-regulated urine concentration is underlined by the recent identification of this site as being spontaneously mutated in congenital progressive hydronephrosis (cph). The spontaneous conversion of the serine phosphorylated site (Ser 256) to leucine in the cytoplasmic tail of the AQP2 protein in cph-mice prevents its phosphorylation at Ser 256 , and inhibits the subsequent accumulation of AQP2 on the apical membrane of the collecting duct principal cells. This causes polydipsia, polyuria, and a severe urinary concentration defect leading to hydronephrosis and obstructive nephropathy in these mice. Recently, three previously unknown phosphorylation sites in the C-terminus of AQP2 were identified (S261, S264, and S269) by using liquid chromatography coupled to mass spectrometry neutral loss scanning. During basal conditions, AQP2 phosphorylated on Ser 261 is approximately 24-fold more abundant than Ser 256 phosphorylated AQP2. During administration of dDAVP a decrease in Ser261 phosphorylation was observed, while AQP2 phosphorylated on Ser256, Ser264, and Ser269 increased. It is clear that phosphorylation of AQP2 is required for cell surface expression; however, it is still unclear how phosphorylation of AQP2 (S256, S261, S264, S269) induces apical trafficking of AQP2. One possibility is that phosphorylation of AQP2 directly influences an interaction between AQP2-containing vesicles and the cell cytoskeleton, microtubules or accessory cross-linking proteins. Indeed, S256 is important for a direct interaction of AQP2 with 70 kDa heat-shock proteins. Alternatively, phosphorylation could prevent endocytosis of AQP2, leading to accumulation at the cell surface.
Prostaglandin E 2 (PGE 2 ) has been known to inhibit vasopressin-induced water permeability by reducing cAMP levels. Zelenina et al. investigated the effect of PGE 2 on PKA phosphorylation of AQP2 in rat kidney papilla, and the results suggest that the action of prostaglandins are associated with retrieval of AQP2 from the plasma membrane, but this appears to be independent of AQP2 phosphorylation by PKA. In contrast, a recent study revealed the effect of selective E-prostanoid receptor agonists on the vasopressin-independent targeting of AQP2. In vitro , PGE2 and selective agonists for the E-prostanoid receptors EP2 or EP4 all increased trafficking and AQP2 phosphorylation (Ser264) in MDCK cells. In vivo , a V2R antagonist caused a severe urinary concentrating defect in rats, which was greatly alleviated by treatment with a selective agonist for the EP2 receptor. Further studies are still needed on the effects of PGE2 on urine concentration.
Phosphorylation of AQP2 by other kinases, e.g., protein kinase C or casein kinase II, may potentially participate in regulation of AQP2 trafficking. Angiotensin II-induced activation of protein kinase C could be, at least in part, involved in the AQP2 trafficking/expression. S256 is also a substrate for Golgi casein kinase 2, which is required for the Golgi transition of AQP2. Additionally, the phosphatase inhibitor okadaic acid induces increased AQP2 expression at the cell surface, even in the presence of the PKA inhibitor H89 and S256A AQP2 mutants (that lack S256 phosphorylation) which are able to accumulate at the cell surface after treatment with cholesterol-depleting agents. Phosphorylation of other cytoplasmic or vesicular regulatory proteins may also be involved. These issues remain to be investigated directly.
Involvement of the Cytoskeleton and Ca 2+ in AQP2 Trafficking
The cytoskeleton has been shown to be involved in AQP2 trafficking in the kidney collecting duct. In particular, the microtubular network has been implicated in this process, since chemical disruption of microtubules inhibits the increase in permeability both in the toad bladder and in the mammalian collecting duct. Thus, AQP2 vesicles may be transported along microtubules on their way to the apical plasma membrane. The microtubule-based motor protein dynein and the associated protein Arp1, which is part of the protein complex dynactin, were found by immunoblotting to be among the proteins associated with AQP2 vesicles from rat inner medulla. Immunoelectron microscopy further supported the presence of both AQP2 and dynein on the same vesicles. Moreover, both vanadate (a non-specific inhibitor of ATPases) and EHNA (a specific inhibitor of dynein) inhibit the antidiuretic response in toad bladder and kidney collecting duct. Thus, it is likely that dynein may drive the microtubule-dependent delivery of AQP2-containing vesicles to the apical plasma membrane.
Actin filaments are also involved in the hydrosmotic response. Recently evidence was provided that the myosin light chain kinase (MLCK) pathway, through calmodulin-mediated calcium activation of MLCK, leads to phosphorylation of myosin regulatory light chain and non-muscle myosin 2 motor activity. Studies in isolated perfused rat inner medullary collecting ducts showed the MLCK inhibitors ML-7 and ML-9 reduce the vasopressin-induced increase in water permeability, indicating that MLCK may be a downstream target for the vasopressin-induced Ca 2+ signal.
The intracellular Ca 2+ concentration has been shown to increase upon stimulation of isolated perfused rat inner medullary collecting ducts with vasopressin or dDAVP. These observations have been followed by a number of studies on the role of Ca 2+ in the vasopressin-induced increase in water permeability. Vasopressin or 8–4-chlorophenylthio-cAMP induced a marked increase in intracellular [Ca 2+ ] in isolated perfused IMCD, and BABTA blocked the rise in intracellular [Ca 2+ ] and co-contaminantly led to inhibition of the vasopressin-induced increase in tubular water permeability. Moreover, blocking calmodulin with W7 or trifluoperazine also inhibited the effect of vasopressin on tubular water permeability, and inhibited the vasopressin-induced AQP2 plasma membrane targeting in primary cultured IMCD cells. Removing Ca 2+ from both bath and lumen in isolated perfused tubules did not affect the vasopressin-induced Ca 2+ signal, indicating that Ca 2+ was released from an intracellular source. This was further supported by the observations that ryanodine inhibited the Ca 2+ signal in perfused IMCDs and inhibited AQP2 accumulation in the plasma membrane in primary cultured IMCD cells. In addition, RyR1 ryanodine receptor was localized to rat IMCD by immunohistochemistry. Ryanodine receptors are generally known to mediate a positive feedback and release Ca 2+ from intracellular stores in response to an initial rise in intracellular [Ca 2+ ]. Thus, it is likely that another mechanism is responsible for the initialization of the rise in intracellular [Ca 2+ ]. Further studies revealed that vasopressin or dDAVP elicited oscillations in intracellular [Ca 2+ ] in isolated perfused rat IMCD. The results on isolated perfused IMCDs indicate that an intracellular increase in [Ca 2+ ] is an obligate component of the vasopressin response leading to increased AQP2 expression in the apical plasma membrane. However, it should be mentioned that there is a discrepancy between the results from primary cultured IMCD cells and isolated perfused IMCD tubules with regard to the role of intracellular [Ca 2+ ] in vasopressin-induced AQP2 trafficking. Consistent with this, Lorenz et al. demonstrated that AQP2 shuttling is evoked neither by AVP-dependent increase of [Ca 2+ ] nor by AVP-independent increase of [Ca 2+ ] in primary cultured IMCD cells from rat kidney, although clamping of intracellular [Ca 2+ ] below resting levels inhibits AQP2 exocytosis. Further studies are required to clarify this discrepancy, which may be related to altered expression levels of vasopressin receptors and/or AQP2 or other elements in the AQP2 trafficking system.
Mechanism of AQP2 Trafficking by Targeting Receptors
The mechanism by which AQP2 vesicles are targeted to the apical plasma membrane and the mechanism by which cAMP controls docking and fusion of vesicles is a current area of active investigation. Considerable insight into this problem has been obtained from previous studies of regulated exocytosis of synaptic vesicles, which involve the actions of multiple proteins. Vesicle-targeting receptors (often referred to as “SNAREs”) are believed to induce specific interaction of vesicles with membrane sites. Vesicle-targeting receptors associated chiefly with translocating vesicles are known as “VAMPs” (vesicle associated membrane proteins, also referred to as “synaptobrevins”) and “synaptotagmins.” Two other families of membrane proteins are believed to serve as receptors in target membranes: the “syntaxins” and SNAP-25 homologs. Several of these SNAREs have been found in the renal collecting duct.
Syntaxins are 30 to 40 kDa integral membrane proteins. Syntaxins have a one bilayer-spanning domain near the C-terminus, so the majority of the protein resides in the cytoplasm. Syntaxins are widely distributed among mammalian tissues. It has been established that syntaxin-4 is expressed in the mammalian collecting duct by studies using polyclonal antibodies raised to conjugated peptides specific for individual syntaxins, and this has been confirmed by reverse transcription polymerase chain reaction. Immunolocalization studies have demonstrated that syntaxin-4 is predominantly located in the apical plasma membrane of collecting duct principal cells, where AQP2 is targeted in response to vasopressin.
VAMPs are believed to induce specific docking of vesicles by interacting directly with syntaxins in the target plasma membrane ( Figure 41.7 ). Although their primary amino acid sequences are not related to syntaxins, VAMPs also have a single bilayer-spanning domain near the C-terminus, and the majority of the protein resides in the cytoplasm. Three VAMP isoforms were initially identified : VAMP-1; VAMP-2; and VAMP-3 (also referred to as “cellubrevin”), and subsequently several additional homologs have been cloned. VAMP-2 and VAMP-3 have been localized in principal cells of renal collecting duct. Double-labeling immunoelectron microscopy revealed that AQP2 and VAMP-2 reside in the same intracellular vesicles in collecting duct principal cells. Furthermore, AQP2 vesicles isolated by differential centrifugation were found to contain VAMP-2.
At this time, several putative vesicle-targeting proteins are known to reside in collecting duct principal cells. VAMP-2 resides in AQP2 intracellular vesicles, and syntaxin-4 resides in the apical plasma membrane. In vitro binding assays have documented that VAMP-2 binds syntaxin-4 with high affinity, but VAMP-2 has not been shown to interact with syntaxin-3 or syntaxin-2. Thus, VAMP-2 and syntaxin-4 are likely participants in the targeting of AQP2 vesicles to the apical plasma membrane; however, a functional role for these targeting proteins remains to be formally demonstrated. A third SNARE protein, SNAP-23, has also been identified in principal cells of the collecting duct. Although considered a target-membrane SNARE (t-SNARE), SNAP-23 is present both in AQP2-containing vesicles and in the apical plasma membrane of principal cells. VAMP-2, syntaxin-4, and SNAP-23 may potentially form a complex with the N-ethylmaleimide-sensitive factor (NSF). Finally, synaptotagmin VIII, a calcium-insensitive homolog, was identified in collecting duct principal cells, and may potentially also play a role in vesicle targeting.
Recently, LC-MS/MS-based proteomic analysis of immunoisolated AQP2-containing intracellular vesicles from rat inner medullary collecting duct revealed that AQP2-containing vesicles are heterogeneous, and that intracellular AQP2 resides chiefly in endosomes, trans-Golgi network, and rough endoplasmic reticulum. Vasopressin-stimulated exocytosis of AQP2 vesicles involves several steps including: (1) translocation of vesicles from a diffuse distribution throughout the cell to the apical region of the cell; (2) translocation of AQP2 across the apical part of the cell composed of a dense filamentous actin network; (3) priming of vesicles for docking; (4) docking of vesicles; and (5) fusion of vesicles with the apical plasma membrane. Theoretically, each of these steps could be a target for regulation by vasopressin. If the SNARE proteins are involved in the vasopressin-induced trafficking of AQP2 to the apical plasma membrane, the regulatory mechanism might involve selective phosphorylation of one of the SNARE proteins or an ancillary protein that binds to them, possibly via protein kinase A or calmodulin-dependent kinase II. Although the SNARE proteins are recognized as potential targets for phosphorylation, there is presently no evidence for phosphorylation in the collecting duct. As noted above, however, AQP2 itself appears to be a target for PKA-mediated phosphorylation at a serine in position 256. The phosphorylation does not modify the single channel water permeability of AQP2, and instead the phosphorylation is believed to play a critical role in regulation of AQP2 trafficking to the plasma membrane. The establishment of a role for these SNARE proteins in regulated trafficking of AQP2 will most likely depend on the preparation of targeted knockouts for each of these genes in the collecting duct.
Long-Term Regulation of Aquaporin Expression
It has been known for more than 40 years that urinary concentrating ability is regulated through a long-term conditioning effect of sustained increases in vasopressin levels exerted over a period of days. The physiological and molecular basis for long-term conditioning of urinary concentrating ability is still being investigated. Water restriction causes a large, stable increase in water permeability of inner medullary collecting ducts dissected from rat kidneys and perfused in vitro . Thus, water permeability of the tubules from water-restricted rats became five-fold greater than that measured in well-hydrated rats by a mechanism independent of the short-term action of vasopressin. Other studies confirmed that water restriction enhances the vasopressin-independent water permeability of collecting ducts, and increases vasopressin-stimulated water permeability. This contrasts with the lack of an observed increase in urea permeability in water-restricted rats. These studies provided the firm conclusion that restriction of water intake for 24 hours or longer leads to a marked increase in the water-reabsorbing capacity of the renal collecting duct epithelium.
Further studies with anti-peptide antibodies have documented that the conditioned increase in water permeability is associated with increased expression of aquaporin proteins in collecting duct principal cells. Specifically, the increased water permeability that occurs in response to water restriction is accompanied by significant increases in the levels of AQP2 and AQP3 proteins in rat collecting ducts. Water restriction for 24 to 48 hours resulted in an approximately five-fold increase in AQP2 protein in the rat renal inner medulla when measured by immunoblotting and by immunostaining. This increase paralleled the increase in water permeability in response to water restriction.
A direct role for vasopressin in AQP2 expression has been established by multiple studies. Continuous infusion of arginine vasopressin into Brattleboro rats for 5 days resulted in a three-fold increase in AQP2 expression and a three-fold increase in water permeability of inner medullary collecting ducts. When the time-course of AQP2 expression in inner medullary collecting duct was studied, it was found that 5 days of vasopressin infusion were required to reach maximal AQP2 levels in Brattleboro rats.
Vasopressin is believed to be essential for evoking AQP2 expression, because Brattleboro rats totally lack circulating vasopressin fail to exhibit increased expression of AQP2 protein after long-term water restriction. Moreover, administration of a selective V2-receptor antagonist blocked the increase in AQP2 expression usually seen with thirsting in normal rats, whereas administration of the V2-selective agonist dDAVP (1-desamino-8-D-arginine vasopressin) to rats elicited a large increase in renal AQP2 mRNA and AQP2 protein abundance. The question of hyperosmolar induction of AQP2 during thirsting has been raised; however, five-day infusion of arginine vasopressin increased AQP2 comparably in renal cortex and medulla. This indicates that the vasopressin-induced expression of AQP2 is not critically dependent on increased osmolality or ionic strength in the tissue surrounding the collecting ducts. Thus, it has been clearly established that the major increase in AQP2 water channel expression elicited by restriction of water intake is due to vasopressin binding to the V2 receptor in collecting duct principal cells. However, studies of the mechanism of escape from the antidiuretic effects of vasopressin make it clear that AQP2 expression is regulated by other factors in addition to vasopressin (see later).
AQP3 protein expression also has been found to parallel AQP2 expression. A marked increase in AQP3 protein expression was observed in rats in response to restriction of water intake and after a five-day infusion of arginine vasopressin. These conditions did not lead to observed changes in AQP1 or AQP4 protein expression. Thus, it is concluded that long-term circulation of high vasopressin levels is associated with an adaptive increase in maximal water permeability in collecting ducts, apparently due a selective increase in the expression of both AQP2 and AQP3 in the principal cells of the collecting duct. However, immunoelectron microscopy has demonstrated that AQP3 is predominantly present in the basolateral plasma membranes with little labeling of intracellular vesicles. This suggests that AQP3 is not regulated by vesicular trafficking (in contrast to the findings with AQP2 ). Moreover, there are several examples where there is a decoupling of AQP2 and AQP3 expression, suggesting that other factors in addition to vasopressin may regulate one or other of the aquaporins. This is seen in conditions such as hepatic cirrhosis, vasopressin-escape, low-protein diet, and restriction of NaCl intake.
The adaptive changes in AQP2 and AQP3 expression are believed to be due to transcriptional regulation of these genes. Increased AQP2 mRNA was found in the inner medullae of water-restricted rats, and in response to infusion of dDAVP. All of these studies indicate that the long-term upregulation of AQP2 expression in the rat kidney is due to elevation of the levels of AQP2 mRNA. Vasopressin-induced increase in AQP2 mRNA expression could reflect either increased gene transcription or reduced transcript degradation. Examination of the 3′-untranslated region of the AQP2 mRNA has not revealed the presence of recognizable mRNA stabilization motifs, and it is presumed that vasopressin directly increases AQP2 gene transcription; however, no direct evidence supports this presumption. Transcriptional regulation would most likely occur through the vasopressin-induced increases in intracellular cyclic AMP that activates protein kinase A in collecting duct cells. Examination of the 5′-flanking region of the AQP2 gene revealed a putative cAMP-response element (CRE), which may play a role in the vasopressin-induced increase in AQP2 expression. Attempts to demonstrate expression of AQP2 promoter-luciferase reporter constructs have been undertaken in cultured renal epithelial cells and suggest that the putative CRE can drive increased expression of the AQP2 gene; however, the inability of the cell line to express AQP2 protein limits the interpretation.
As with AQP2, AQP3 mRNA levels were induced by infusion with the selective V2 agonist dDAVP. Examination of the 5′-flanking DNA of AQP3 failed to reveal a CRE; however, Sp1 and AP2 cis-regulatory elements are present, and these are known to be associated with cyclic AMP-mediated transcriptional regulation of other genes. A previous study revealed that AQP2 is ubiquitinated with one UbLys63-linked poly-Ub chain at K270 of AQP2 and lysosomal degradation was extensive for ubiquitinated AQP2. The degradation pathways, therefore, balance the abundance of proteins which play an important role in body water homeostasis.
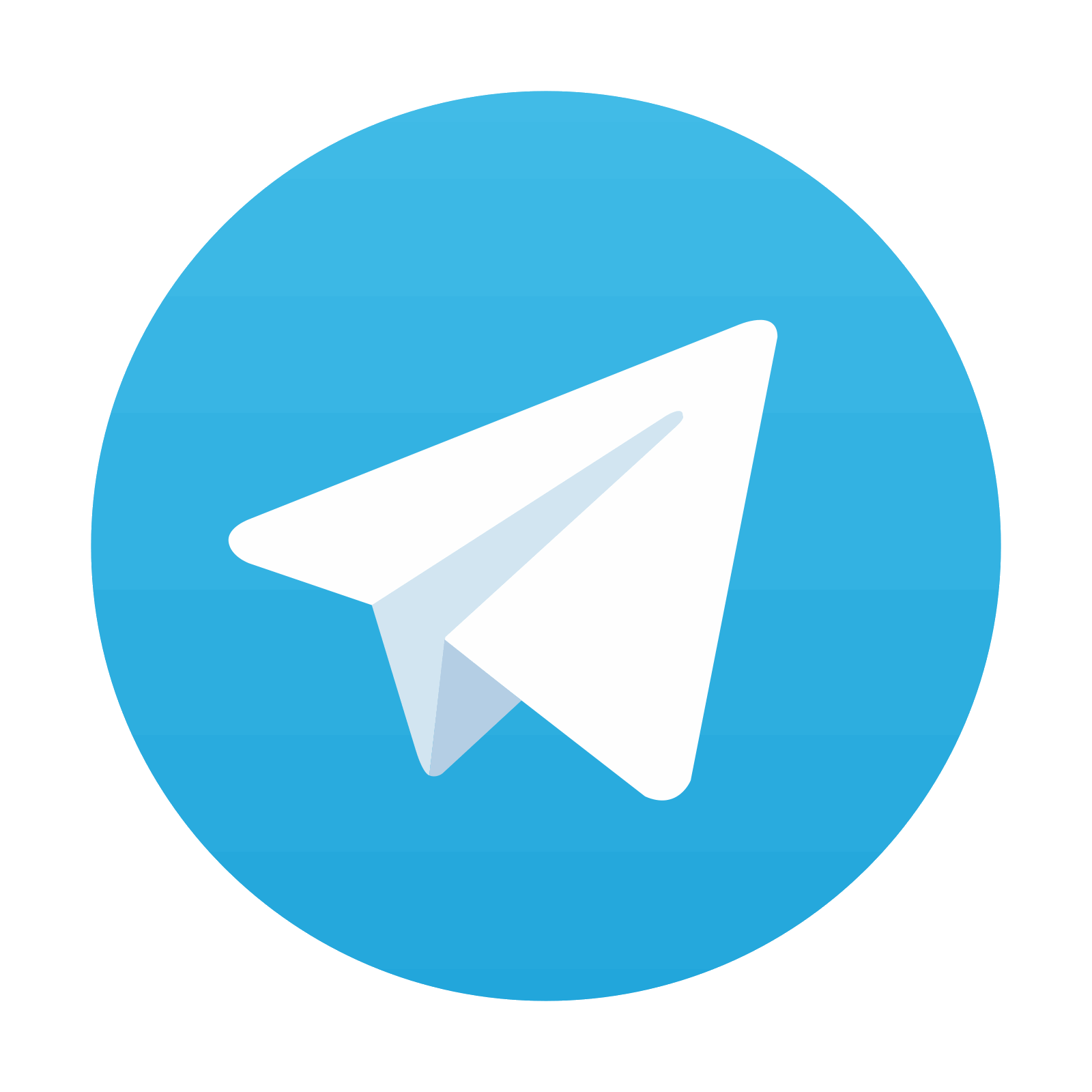
Stay updated, free articles. Join our Telegram channel
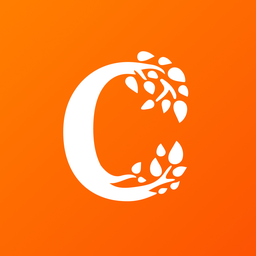
Full access? Get Clinical Tree
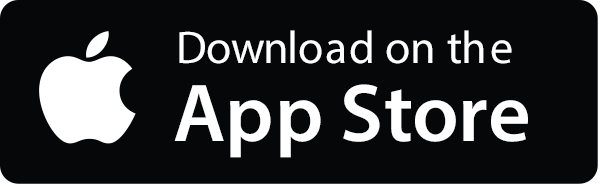
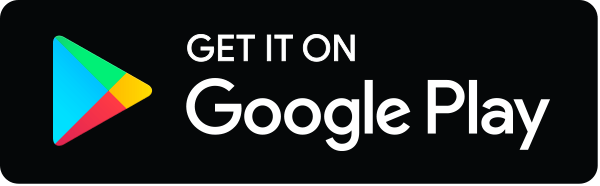