When an organ or tissue from one member of a species is transplanted into a nonidentical member of the same species, an immune response ensues. This response is termed the alloimmune response , and it is primarily orchestrated by initial T-cell recognition of alloantigens (allorecognition, signal 1). However, full activation of T cells requires in addition to signal 1 two other sequential signals: signal 2 delivered by ligation of costimulatory receptors on T cells by ligands on antigen-expressing cells (APCs); and signal 3 delivered by multiple cytokines that regulate cell proliferation, differentiation, and survival/death. The first part of this chapter reviews the process of T-cell allorecognition with particular emphasis on recent advances in our understanding of T-cell costimulatory pathways and novel cytokines in regulating different phases of T-cell responses in transplant rejection. Activation of alloreactive T cells subsequently leads to the initiation of the effector mechanisms of the immune system, resulting in allograft destruction. Recently, B cells and alloantibodies against human leukocyte antigen (HLA) and non-HLA targets have been increasingly recognized as critical in the pathogenesis of acute and chronic allograft dysfunction. Furthermore, although T cells alone are necessary and sufficient for the rejection of allografts, evidence is accumulating on the role of humoral and cellular components of the innate immune system in allograft rejection and prevention of tolerance. The second part of this chapter focuses on the various effector mechanisms of allograft rejection. The ultimate goal in clinical transplantation is to induce a state of donor-specific immunological tolerance, where recipients can accept organs without the need of exogenous immunosuppression. The final part of this chapter reviews the most promising approaches to inducing transplantation tolerance. In particular, we highlight the biological basis and putative mechanisms of tolerance and discuss some of the major stumbling blocks to the induction and maintenance of tolerance in clinical transplantation.
The Alloimmune Response
A
Transplantation Antigens
Major Histocompatibility Complex
The major histocompatibility complex (MHC) antigens are the strongest and most polymorphic transplantation antigens, and they can stimulate the primary immune response without priming. The recipient T-cell receptor’s recognition of donor MHC/peptide complexes is the central event in the initiation of an alloimmune response, with the allogeneic donor HLA antigens being the main immunological targets of different effector arms on the immune system. In some cases, antibodies against donor HLA antigens may have been previously generated because of pregnancies, blood transfusions, or previous transplantation. These preformed antibodies may cause hyperacute or accelerated acute graft rejection at the time of transplantation, though immunological testing has reduced the frequency of this type of rejection. Later after transplantation, the appearance of de novo donor-specific antibodies has been linked to chronic allograft dysfunction.
T lymphocytes recognize foreign (nonself) antigens in the context of self cell-surface molecules encoded in the MHC. In humans, this genetic region is located in a 3.5 million base-pair region on the short arm of chromosome 6. This locus is further subdivided into three clusters based on the structure and function of the proteins encoded by the genes. Human MHC molecules are called HLAs, and the three regions have been designated HLA class I, class II, and class III ( Fig. 35.1 ).

HLA class I molecules (classic HLA-A, -B, and -C, and other nonclassic molecules) are composed of a 44-kD heavy chain and a 12-kD light chain ( Fig. 35.2 ). The amino terminus portion of the heavy chain that extends into the extracellular space is composed of three domains: alpha1, alpha2, and alpha3. The alpha1 and alpha2 domains interact to form the sides of a cleft (or groove). The cleft is the site where foreign proteins bind to MHC molecules for presentation to T cells. The highly variable amino acid residues located in the groove determine the specificity of peptide binding and T-cell antigen recognition. The light chain, β 2 -microglobulin, stabilizes the heavy chain such that displacement of β 2 -microglobulin from the class I molecule causes a loss of heavy chain native structure. Class I molecules are expressed on essentially all nucleated cells. The function of intact class I molecules is to present antigenic peptides as protein fragments (peptides) in the context of self to T lymphocytes. HLA class I molecules are highly polymorphic. This polymorphism aids in maximizing the potential for peptide binding by the species. Peptides bind within the HLA class I groove (see Fig. 35.2 ) based on the sequence of amino acids in the peptide-binding region. HLA class I molecules tend to bind peptides of 9 to 11 amino acids in length. These peptides fit tightly into the groove and do not extend out of the ends of the molecule. MHC class I molecules generally present endogenous proteins ( Fig. 35.3 ). These proteins, such as viruses and normal self proteins, are degraded in the cytoplasm in proteasomes. Short peptide sequences are then moved to the endoplasmic reticulum through specific transporters associated with antigen presentation (TAP transporters). In the endoplasmic reticulum, these peptides associate with class I heavy chain and β 2 -microglobulin, and the mature complex is transported to the cell surface, where it can be recognized by T lymphocytes. Peptides sit in the middle of the groove and run the length of the cleft. The rules for peptide binding are restricted to certain “motifs” based on the polymorphisms described earlier but are lax enough to permit the binding of many different peptides to a single HLA type (allele). Antigens associated with class I molecules are recognized by cytotoxic CD8 + T lymphocytes.


The second major region of the MHC is called the class II locus (see Fig. 35.1 ). HLA class II molecules—HLA-DP, DQ, and DR—are composed of polymorphic α and β chains of about 35 and 31 kD, respectively. These two chains associate to form a peptide-binding region, but unlike class I molecules, determinants of the peptide-binding region are contributed by both chains ( Fig. 35.2B ). MHC class II molecules bind longer peptides—typically 12 to 28 amino acids. In contrast to class I, MHC class II molecules have binding grooves that are open at the ends, permitting peptides of greater lengths to extend beyond the groove (see Fig. 35.3 ). Class II molecules tend to bind antigens that are derived from the exogenous pathway (see Fig. 35.3 ). MHC class II α and β chains associate in the endoplasmic reticulum with an invariant chain, a portion of which binds in the antigen-binding groove. This invariant chain appears to both protect the groove from peptide binding and permit proper folding of the class II complex. A nonomeric form exits the endoplasmic reticulum and moves through the Golgi apparatus and into an endosomal compartment. Within the endosome, the invariant chain is degraded, leaving a shorter class II–associated invariant chain peptide (CLIP) fragment in the groove. Peptides from the extracellular space are taken up into endosomes by endocytosis and targeted to the “compartment for peptide loading.” Within this specialized intracellular compartment, exogenous peptides displace CLIP, and the mature heterotrimeric complex (α, β, and peptide) is transported to the cell surface, where it can be recognized by T lymphocytes. Class II molecules are only expressed on specialized cells such as professional APCs and endothelial cells, and antigens bound to class II MHC molecules are usually recognized by CD4 + helper T cells. In addition to the classic class II antigens, the MHC class II region encodes proteins that make up the proteasome (low-molecular-weight proteins LMP-2 and LMP-7) and the TAP transporters (TAP-1 and TAP-2) (see Fig. 35.1 ). Polymorphisms associated with these structures probably also contribute to the specificity and diversity of peptide binding and antigen presentation. The class III region encodes a variety of proteins of immunological relevance (see Fig. 35.1 ). These include the tumor necrosis factor (TNF) and the complement proteins factor B and C4.
As just outlined, MHC molecules are highly polymorphic, because each MHC locus can express any one of hundreds of different molecules encoded by various alleles . The set of different alleles expressed on one chromosome is called a haplotype . Each parental chromosome 6 provides a haplotype to the offspring. Haplotypes are usually inherited intact from each parent, although crossover between the A and B locus occurs in about 2% of offspring, resulting in a recombination and a new haplotype. A genotype is the sum of two haplotypes. A child is by definition a one-haplotype match to each parent unless recombination has occurred. In addition, the MHC molecules are codominantly expressed—that is, an individual expresses alleles from both chromosomes at each locus. Therefore the MHC genotype of an individual consists of 12 different MHC molecules (two alleles from each of six loci). In clinical transplantation, the most polymorphic MHC genes are HLA-A, -B, and -DR and therefore historically identified as the most important transplant targets. The antigenic determinants of their six alleles are the focus of attempts at HLA matching to improve graft survival. Given the remarkable degree of polymorphism exhibited by MHC genes, there are 88 recognized antigens encoded by more than 1000 distinct alleles, and the number of new alleles is still increasing. The HLA antigens were initially identified using antisera obtained from multiparous women and named in the sequence they were discovered (for example, A1, A2, etc.). However, introducing DNA technologies for HLA testing resulted in the identification of growing numbers of alleles that could be identified by their unique nucleotide sequences within the antigen designations. Thus the serological nomenclature was modified to associate alleles with antigens, and four-digit designations were developed in which the antigen designation makes up the first two digits and the sequential allele designation makes up the third and fourth digits. For example, the first allele for HLA-A1 is HLA-A∗0101, which includes the locus (A), an asterisk to indicate the typing was performed by DNA methods, the serological antigen (01), and the allele number (01). As an example, HLA-B has more than 3600 different alleles. Although HLA gene polymorphism plays a critical role in protective immunity by enabling the binding and presentation of a wide variety of microbial peptides to T cells, it creates a practical barrier to successful transplantation. In fact, it has recently been found that differences between MHC molecules by as little as one or two amino acids (called micropolymorphism ) in the antigen-binding cleft can change the size and diversity of the peptide repertoire presented by each HLA molecule. It is not feasible to select a completely HLA-matched donor for every potential recipient because of the enormous polymorphism of the HLA system. Although allele differences between the donor and the recipient of bone marrow transplants (even micropolymorphism) lead to graft-versus-host disease, HLA mismatches affect primarily the long-term graft survival of kidney transplantation with the best outcomes in six HLA-matched kidneys compared with zero matched kidneys.
The alloimmune response is strong and does not require priming. At least part of the basis for the greater magnitude of the allogeneic response is the relatively high frequency of T-cell precursors that are capable of responding to a foreign MHC antigen. For example, the frequency of specific T cells to conventional antigens is approximately 1 in 10 4 to 10 5 , whereas the frequency responding during allogeneic stimulation can be as high as 1 in 10 1 to 10 2 . The concept of positive and negative selection in the thymus (see mechanisms of self tolerance) also helps explain the strength of the alloimmune response. During development, T cells with receptors of too high an affinity are deleted (negative selection), whereas those with too low an affinity are not selected. The result of this selection is that T-cell receptors (TCRs) of intermediate affinity exit the thymus and enter the periphery. Within an individual, clonal deletion occurs early in development. Potentially, autoreactive clones (with too high an affinity for self) are deleted; failure of deletion of some clones may lead to autoimmunity. In the case of transplantation across an allelic difference, however, the recipient’s T cells do not contact allo-MHC molecules during development in the thymus and thus escape the deletion (negative selection) imposed by interaction with self-MHC. Thus the result is the large number of donor MHC/peptide complexes on the graft to which a potential recipient has not been tolerized during ontogeny. Moreover, the relatively low affinity of any given TCR for its ligand suggest that each T cell could potentially recognize more than one MHC/peptide complex. The high density of alloantigens on the surface of an allograft additionally contributes to the strong T-cell response. In addition, because recipient T cells recognize intact allogeneic MHC molecules directly (see following), they are stimulated maximally by the high density of MHC on the surfaces of transplanted cells.
Minor Transplantation Antigens
Studies involving MHC identical grafts in mice indicate that minor histocompatibility antigens can also mediate rejection. Recipient T cells can be directly primed to minor histocompatibility antigens. A minor histocompatibility antigen is molecularly defined as a donor-derived peptide presented on a donor cell by an MHC molecule shared by a donor and a recipient. Note that the donor and the recipient express the same MHC molecules. Donor dendritic cells (DCs) directly prime CD8 + T cells to become effector cells without the need for further antigen processing by recipient APCs. As one illustration, CD8 + T cells from female C57BL/6 mice specific for male-derived H-Y Uty peptide + MHC class I mediate rejection of syngeneic C57/BL6 male skin. In humans, it has been recognized for several years that minor histocompatibility antigens can be immunogenic from observations based on organ graft rejections and bone marrow graft-versus-host reactions in cases of genetically matched HLA antigens. A few cases of donor-directed, HLA class I–restricted, cytotoxic T-cell responses have been demonstrated in such cases. Two general families of such antigens have been identified :
- 1.
H-Y antigens are proteins encoded on the Y chromosome. Females of the species may mount an immune response against these proteins.
- 2.
T cells recognize peptidic antigens corresponding to polymorphisms among autosomal proteins expressed by individuals of the species. Examples include mitochondrial proteins and enzymes. In the presence of both major and minor incompatibilities, it is clear that the alloimmune response targeted against the MHC molecules predominates.
Several studies reported a detrimental role for minor H antigen-specific T cells in graft survival. However, some data indicate that minor H antigens might also be clinically involved in preventing rejection of solid organ grafts, possibly by induction of regulatory T cells.
ABO Blood Group Antigens
The A and B groups are glycosylated differentially, whereas group O lacks the enzymes necessary for glycosylation. The antigens are readily recognized by natural antibodies, termed hemagglutinins because they cause red cell agglutination. They are relevant to transplantation because they are expressed on other cell types, including the endothelium. Thus they cause hyperacute rejection of vascular allografts because of preformed natural antibodies. Allograft rejection as a result of red blood cell type mismatching can be readily prevented by routine blood typing before transplantation. A2 antigen has significantly lower expression on endothelium, so that transplantation of A2 kidneys to B recipients in the absence of high titers against A2 is feasible. The rhesus (Rh) factor and other red cell antigens are of little concern because they are not expressed on endothelial cells.
Monocyte and Endothelial Cell Antigens
Occasionally, allografts undergo hyperacute rejection, despite appropriate ABO matching. Some of these rejection episodes have been attributed to additional non-ABO antigens expressed on endothelial cells (such as MICA) and monocytes, but these antigen systems remain poorly understood. Pretransplant tissue typing does not currently evaluate the endothelial/monocyte antigens because of the apparent rarity of such antibodies and the lack of accurate reagents for typing.
B
Cellular Events Leading to Allograft Rejection
In the context of allograft rejection, T cells play a central role in orchestrating the immune response because they recognize alloantigens through three distinct pathways (see later). Once activated, they secrete cytokines and chemokines to activate and attract various effector cells, such as CD8 + T cells and macrophages into the allograft. They are also able to interact with B cells that will secrete highly specific alloreactive antibodies. These cells in turn mediate the effector mechanisms of allograft destruction (see following). Box 35.1 summarizes the steps leading to allograft rejection. Furthermore, although T cells alone are necessary and sufficient for the rejection of allografts, evidence is accumulating about the role of the innate immune system in allograft rejection and prevention of tolerance.
- 1.
Recognition of the alloantigen (direct, semi-direct, and indirect pathways)
- 2.
T-cell and B-cell activation, differentiation, and expansion
- 3.
Effector functions
- 4.
Resolution of the response with residual memory
Allorecognition Pathways
The first step in an alloimmune response is the recognition of alloantigens by T cells (priming of alloreactive T cells). In the setting of a transplant, there is the potential for three different cellular mechanisms of allorecognition; these have been called the direct, semi-direct, and indirect pathways of allorecognition ( Fig. 35.4 ).

Direct refers to cell recognition of a whole, intact foreign MHC molecule on the surface of donor cells. Although the specific peptide (typically derived from endogenous proteins, including MHC antigens) bound in the groove of the MHC molecule may be important in this recognition process, it does not restrict this response. The graft, which includes donor bone marrow–derived APCs, usually expresses several class I and class II MHC molecules that differ from the recipient’s MHC molecules, and which can directly stimulate recipients T cells. In sum, donor APCs prime CD4 + and CD8 + T cells through the direct pathway. However, as these donor APCs are destroyed during the priming process, direct T-cell priming is likely to be time limited. Thus direct allorecognition may account for early acute cellular rejection. Consistent with this idea, direct alloreactivity was not detectable in the peripheral blood of a cohort of renal allograft recipients with chronic allograft dysfunction several years after transplantation. In contrast, indirect refers to T-cell recognition of nonself MHC-derived peptides (allopeptides) in the context of self MHC molecules expressed on recipient APCs. In this case, similar to the physiological pathway of antigen recognition, the peptide sequence determines the response. Indirect presentation could occur through a number of mechanisms: soluble donor MHC molecules are shed from the graft and drain through the bloodstream/lymphatics to the recipient secondary lymphoid organs, where they would be processed/presented by recipient APCs to recipient T cells. Alternatively, donor graft cells that migrate to recipient secondary lymphoid organs could be endocytosed by recipient APCs. Third, recipient monocyte/macrophages entering the donor graft could endocytose donor antigens and present the peptides to recipient T cells. Other allopeptides may be derived from minor histocompatibility antigens or tissue-specific antigens. Because recipient monocytes migrating through the allograft can constantly endocytose donor antigen, priming through the indirect pathway could occur for as long as the graft is present in the host. Thus, although indirect alloreactive T cells may participate in acute rejection, they may play a predominant role in chronic rejection. Consistent with this concept, several groups have now provided data correlating the indirect alloreactive T cells with the presence of chronic allograft dysfunction. Interestingly, recent emerging data demonstrate that not only CD4 + T cells, as traditionally thought, but also CD8 + T cells can be primed through the indirect pathway of allorecognition and contribute to graft destruction.
Endothelial cells of donor origin are located at the interface between the recipient’s blood and the allograft and have been implicated in graft rejection ( Fig. 35.5 ). Graft endothelial cells express MHC class I and II molecules and have been found recently to promote direct allorecognition by serving as APCs and as targets for T-cell–mediated cytotoxicity. In addition, endothelial cells may promote indirect allorecognition by a crosstalk mechanism, which involves the recruitment and transformation of recipient monocytes by endothelial cells into highly efficient antigen-presenting dendritic cells.

More recently, a third pathway of alloantigen presentation has been discovered in which recipient APCs acquire intact allogeneic HLA-peptides complexes from donor cells and present them to directly reactive recipient T cells. This presentation is called a semi-direct or cross-dressing pathway to differentiate it from the true direct presentation performed by donor APCs. Marino et al. described a murine model of recipient APC cross-dressing, in which the HLA molecules derived from donor exosomes and donor immune cells were acquired by recipients APCs (see Fig. 35.4 ). The relative contribution of this pathway to allograft rejection is still not clear.
Finally, the question of where T cells meet the transplant antigens has been the target of much study. The site of alloantigen recognition had been believed to be in the allograft itself, but data seem to indicate that peripheral lymphoid organs are required for allograft rejection. Whether unprimed T cells encounter antigens first outside the allograft in peripheral lymphoid tissue or they migrate to secondary lymphoid organs after they encounter the alloantigens in the graft for further maturation and differentiation remains controversial. Interestingly, primed/effector/memory cells appear to mediate graft rejection independent of peripheral lymphoid organs, suggesting that they are activated by alloantigens in the graft itself.
T-Cell Activation
Allograft rejection is a T-cell–dependent process; animals that lack T cells do not reject an allograft. In particular, CD4 + helper T cells appear to be essential orchestrators of the alloimmune response leading to allograft rejection. T lymphocytes initiate the immune response, which ultimately results in graft rejection. In addition, they can function as regulators and effectors in the immune response (see following). As just discussed, allorecognition is the essential initial step for initiation of the cascade of events that results in rejection of the graft (see Box 35.1 ). The essential cell-cell interactions between T cells and APCs (donor or self) may involve five classes of receptors: the antigen-specific TCR, the CD4 or CD8 coreceptor, costimulatory molecules, accessory or adhesion molecules, and lymphokine receptors. Members of each class of receptors may present suitable targets for therapeutic and experimental manipulation and are thus discussed next.
T-cell receptor–CD3 complex
T-cell recognition of alloantigens on APCs is the central event that initiates allograft rejection. The interaction between T lymphocytes and APCs involves multiple T-cell surface molecules and their counterreceptors expressed by APCs. Antigen specificity is determined by the TCR, which recognizes processed antigens in the form of short peptides that are bound to an MHC molecule. Clonally restricted TCRs are made up of two chains. The major TCR is an α, β heterodimer, and a less commonly expressed TCR consists of γ and δ chains. The TCR consists of constant and variable portions involved in binding to HLA and recognition of the specific alloantigenic targets. Although the TCR allows T cells to recognize antigen-MHC complexes, the cell-surface expression of TCR molecules and the initiation of intracellular signaling depend on a complex of additional peptides known as the CD3 complex. After a given TCR is engaged by alloantigen, the T cell is activated and a signal (signal 1) is transduced through the TCR-CD3 complex. As will be discussed following, full activation of T cells requires two synergistic signals (see Costimulatory Molecules).
Rabbit antithymocyte globulin (rATG; thymoglobulin or ATG), one of the most common induction therapy agents, targets multiple T-cell surface molecules, including CD3. rATG is typically prepared by immunizing pathogen-free rabbits, with a cell suspension of human thymic tissue (thymocytes). After immunization, the serum is harvested from rabbits, and immunoglobulins against thymocytes are isolated and subjected to a number of purification processes. Samples from more than 26,000 immunized rabbits are pooled to achieve a high level of batch-to-batch consistency. The resulting antibodies are polyclonal and target multiple different surface molecules present on T cells. The mechanism of action of ATGs is lymphocyte depletion, predominantly by complement-dependent cells lysis.
CD4 and CD8 T-cell receptor coreceptors
The two major subsets of T cells, cytotoxic CD8 + T cells and helper CD4 + T cells, recognize processed antigens on MHC class I and II molecules, respectively. CD4 and CD8 molecules enhance the interaction between the TCR and APCs through the MHC. By binding class II MHC molecules, the CD4 molecule facilitates TCR-CD3 complex-mediated signal transduction and assists the actions of class II MHC–restricted T cells. Similarly, the CD8 molecule binds to class I MHC molecules and stabilizes the interaction of the class I MHC–restricted T cell with a target cell–mediating signal transduction. Thus CD4/CD8 + TCR–CD3 complex proteins function together in initiating the signals for T-cell activation.
Adhesion molecules
Immune cells gain access to the site of inflammation in the graft from nearby lymph nodes and the bloodstream. Associated with transplantation, alloantigens enter local lymph nodes. APCs, such as dendritic cells and tissue macrophages, take up foreign HLA (indirect allorecognition) or exosomes containing foreign HLA (semi-direct allorecognition), or foreign HLA on donor APCs is recognized directly (direct allorecognition). Antigen-specific T cells are activated, differentiate, divide, and enter the bloodstream.
Immune cells move from the bloodstream into the site of inflammation by a three-step process. First, they roll along the vessel wall through interactions between selectins on the endothelium and receptors on the immune cells. Second, they adhere to vessel endothelium. Third, chemoattractant cytokines (chemokines) are released ( Fig. 35.6 ). Adhesion molecules and chemokines are important regulators of rejection and appear to be targets for immunotherapy. Adhesion molecules (integrins) are well known for their ability to facilitate adhesion between cells and between cells and the extracellular matrix. Integrins on T cells include lymphocyte function-associated antigen 1 (LFA-1), which interacts with intercellular adhesion molecules (ICAM) 1 and 2; CD2, which interacts with CD58 (leukocyte function-associated antigen 3 [LFA-3]); and very-late-appearing antigen (VLA-4) (CDw49d, CD29), which interacts with vascular cell adhesion molecule (VCAM-1, CD106). These receptors are of two large structural families. The integrins, including LFA-1 and VLA-4, are made up of α, β heterodimers, whereas members of the immunoglobulin superfamily, including CD2, LFA-3, VCAM-1, and the ICAMs, are made up of disulfide-linked “receptor” domains. The inhibition of adhesion cell function has been found to be immunosuppressive. Previous studies in murine and primate models reported increased graft survival with anti–ICAM-1 monoclonal antibodies; however, in a randomized multicenter trial, short-term use of the anti–ICAM-1 monoclonal antibody enlimomab for induction therapy after renal transplantation did not reduce the rate of acute rejection or delayed graft function. No agents targeting trafficking of T cells are being used in clinical transplantation.

Costimulatory molecules
T cells require two signals for full activation ( Fig. 35.7 ). One signal is provided by the interaction of the TCR with the MHC-peptide complex; the second “costimulatory” signal depends on one or more additional receptor-ligand interactions between T cells and APCs. The two-signal model has gained enormous support, defining several costimulatory pathways, and is now widely accepted. Traditionally these molecules were broadly grouped into two families based on their molecular structures: the immunoglobulin (Ig) superfamily and the tumor necrosis factor receptor (TNFR) family. The CD28-B7 (from the Ig superfamily) and CD154-CD40 pathways (from the TNFR family) have been studied the longest and described as the critical costimulatory pathways for T-cell activation. Blockade of these pathways has been reported to regulate both autoimmune and alloimmune responses in experimental models and in human disease. However, studies have indicated that inhibition of these pathways is insufficient to reproducibly induce long-lasting immunological tolerance in some experimental autoimmunity and transplantation models, indicating a role for other costimulatory pathways. In fact, the T-cell immunoglobulin mucin (TIM) family of molecules have been described as having costimulatory properties (see following).

Emerging data suggest that the costimulatory pathways exhibit some redundancy, hierarchy, and unique functions where various costimulatory molecules affect different T-cell populations and act at different times during the course of the immune response. In addition, a growing number of negative T-cell costimulatory or coinhibitory pathways have been found to play an important role in the regulation of T-cell responses. Thus, in the next section, we first review the role of the most widely studied CD28-B7 and CD154-CD40 pathways in transplantation. Thereafter, we review some novel emerging data from studies about the role of costimulatory molecules in transplantation.
The CD28/CTLA4–B7 pathway
Many T-cell molecules may serve as receptors for costimulatory signals; the CD28 molecule is the best characterized of these molecules ( Fig. 35.8 ). According to gene expression studies, CD28 costimulation can lead to significant augmentation of expressions of genes induced by TCR signaling alone. These findings are consistent with a model of costimulation in which CD28 signaling lowers TCR thresholds for activation of cells. CD28 has two known ligands, B7-1 (CD80) and B7-2 (CD86), both of which are expressed primarily on activated professional bone marrow–derived APCs. T cells also express cytotoxic T-lymphocyte-associated protein 4 (CTLA-4), a molecule that is highly homologous to CD28 that also binds CD80 and CD86. However, unlike CD28, CTLA-4 transmits an inhibitory signal that serves to terminate the immune response. Although CD28 is expressed by both resting and activated T cells, CTLA-4 is expressed only on activated T cells. Because CTLA-4 binds B7 molecules with a higher affinity than does CD28, its inhibitory interaction eventually predominates, leading to the termination of the immune response. The importance of CTLA-4 as a negative regulatory T-cell costimulatory molecule in the physiological termination of T-cell responses is highlighted by the observation that CTLA-4 gene knockout mice develop massive lymphoproliferation and early death. One point that needs to be emphasized is the critical role of costimulation in T-cell responses. In the absence of costimulatory signals, sometimes the T cell simply ignores the peptide–MHC complex presented to it. At other times, the T cell undergoes apoptotic death or is rendered anergic for up to several weeks; that is, the T cell is unable to respond to antigens, even when they are presented by APCs that express a costimulatory molecule. Precisely what determines the outcome of the stimulation of T-cell antigen receptors in the absence of costimulation (ignorance, apoptosis, or anergy) is not known. This process is considered one important factor in induction of peripheral tolerance (see following). Thus manipulation of the CD28/B7 pathway has been envisioned as a potential strategy for achieving therapeutically useful immunosuppression or tolerance. T-cell costimulatory blockade in the form of CTLA-4Ig, which blocks CD28 interaction with B7, has been extensively studied as a means of immunosuppression. To improve the biological potency of CTLA4-Ig, CTLA4-Ig was mutated by substituting amino acids at two positions. The resulting protein LEA29Y (belatacept) had a significantly higher affinity for both CD86 and CD80.
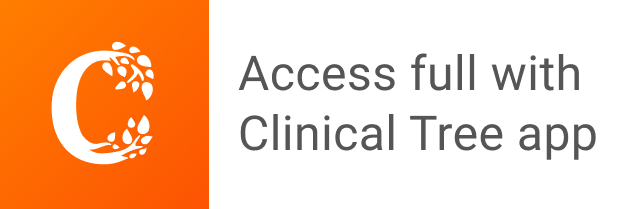