Anthony Atala, MD
Four medical firsts are described in the passage cited above: the use of anesthesia, surgery, cloning, and tissue engineering. Even three decades ago, the performance of two of these, tissue engineering and cloning, was not possible. The use of one body part for another or the exchange of parts from one person to another was mentioned in the medical literature even in antiquity and captured the imagination of many over time. The maintenance of organs in culture was a major area of inquiry in the early 1900s. Charles Lindbergh, the first pilot to successfully fly across the Atlantic in the 1920s, joined forces with Alexis Carrel, a Nobel Prize winner in the field of medicine, to investigate the potential of keeping organs alive ex vivo long-term (Carrel et al, 1935).
The field of urology was the earliest to gain from the advent of transplantation, with the kidney being the first entire organ to be replaced in a human, in 1955 (Guild et al, 1955). In the early 1960s, Joseph Murray, who later received the Nobel Prize for his work, performed a nonrelated kidney transplantation from a nongenetically identical patient into another. This transplant, which overcame the immunologic barrier, marked a new era in medical therapy and opened the door for use of transplantation as a means of therapy for different organ systems. However, lack of good immune suppression and the ability to monitor and control rejection, as well as a severe organ donor shortage, opened the door for other alternatives.
In the 1970s, a natural evolution occurred wherein researchers started to combine the field of devices and materials sciences with cell biology, in effect starting a new field called tissue engineering. As more scientists from different fields came together with the common goal of tissue replacement, the field of tissue engineering became more formally established. Tissue engineering was defined as “an interdisciplinary field which applies the principles of engineering and life sciences towards the development of biological substitutes that aim to maintain, restore or improve tissue function” (Atala et al, 2001). The first use of the term “tissue engineering” in the literature can be traced to a reference dealing with corneal tissue in 1985 (Wolter et al, 1985).
The field of stem cells also received a large boost with the discovery of mouse embryonic stem cells in the early 1980s (Martin, 1981). However, the field remained relatively dormant until the description of human embryonic stem cells in 1998 (Thomson et al, 1998). The description of these cells led to one of the most contested ongoing ethical debates in the field of medicine. Just a year later, in 1999, the world awoke to the startling media announcement of the creation of the first cloned mammal, a sheep named Dolly (Wilmut et al, 1997). Although cloning, or nuclear transfer, had been done in amphibians and other animal models for years, this accomplishment in a mammal showed once again that concepts believed to be scientifically prohibitive were indeed possible.
The fields of cell transplantation, tissue engineering, and nuclear transfer all had one unifying concept—the regeneration of living tissues and organs. Thus in 1999, William Haseltine, then the scientific founder and Chief Executive Officer of Human Genome Sciences, coined the term “regenerative medicine,” in effect bringing all these areas under one defining field (Haseltine, 1999). Soon, the first online journal for the new field of tissue engineering, Regenerative Medicine, was formed, along with The Regenerative Medicine Society.
Sources of Cells for Therapy
Stem Cells
The cells used for regenerative medicine can be autologous or heterologous, and from either native or stem cell sources. In general, there are three broad categories of stem cells obtained from living tissue that are used for cell therapies. Embryonic stem cells are obtained through the aspiration of the inner cell mass of a blastocyst or, more recently, a single cell from this mass. Fetal and neonatal amniotic fluid and placenta may contain multipotent cells that may be useful in cell therapy applications. Adult stem cells, on the other hand, are usually isolated from organ or bone marrow biopsies. Stem cells are defined as having three important properties: the ability to self-renew, the ability to differentiate into a number of different cell types, and the ability to easily form clonal populations (populations of cells derived from a single stem cell). Many techniques for generating stem cells have been studied over the past few decades. Some of these techniques have yielded promising results, but others require further research. The main techniques are discussed in detail below, and their advantages and limitations are summarized in Table 19–1.
Table 19–1 Summary of Alternate Methods for Generating Pluripotent Stem Cells
METHOD | ADVANTAGES | LIMITATIONS |
---|---|---|
Somatic cell nuclear transfer | Customized stem cells Has been shown to work in nonhuman primates | Requires oocytes Has not been shown to work in humans |
Single cell embryo biopsy | Patient-specific to embryo Does not destroy or create embryos Has been done in humans | Allogeneic cell types Is not known if single cells are totipotent Requires coculturing with a previously established human embryonic stem cell line |
Arrested embryos | Cells obtained from discarded embryos Has been done in humans | Allogeneic cell types Quality of cell lines might be questionable |
Altered nuclear transfer | Customized stem cells | Ethical issues surround embryos with no potential Modified genome Has not been done with human cells |
Reprogramming | Customized stem cells No embryos or oocytes needed Has been done with human cells | Retroviral transduction Oncogenes |
Embryonic Stem Cells
In 1981 pluripotent cells were found in the inner cell mass of the human embryo, and the term “human embryonic stem cell” was coined (Martin, 1981). These cells are able to differentiate into all cells of the human body, excluding placental cells (only cells from the morula are totipotent; that is, able to develop into all cells of the human body). These cells have great therapeutic potential, but their use is limited by both biological and ethical factors.
The political controversy surrounding stem cells began in 1998 with the creation of human embryonic stem (hES) cells derived from discarded embryos. hES were isolated from the inner cell mass of a blastocyst (an embryo 5 days postfertilization) using an immunosurgical technique. Given that some cells cannot be expanded ex vivo, ES cells could be an ideal resource for regenerative medicine because of their fundamental properties: the ability to self-renew indefinitely and the ability to differentiate into cells from all three embryonic germ layers. Skin and neurons have been formed, indicating ectodermal differentiation (Reubinoff et al, 2001; Schuldiner et al, 2001; Zhang et al, 2001). Blood, cardiac cells, cartilage, endothelial cells, and muscle have been formed, indicating mesodermal differentiation (Kaufman et al, 2001; Kehat et al, 2001; Levenberg et al, 2002). Pancreatic cells have been formed, indicating endodermal differentiation (Assady et al, 2001). In addition, as further evidence of their pluripotency, embryonic stem cells can form embryoid bodies, which are cell aggregations that contain all three embryonic germ layers while in culture, and can form teratomas in vivo (Itskovitz-Eldor et al, 2000). These cells have demonstrated longevity in culture and can maintain their undifferentiated state for at least 80 passages when grown using current published protocols (Reubinoff et al, 2000; Thomson et al, 1998).
Single Cell Embryo Biopsy
Because a major objection to hES cell research for some persons is that it results in the destruction of embryos, a method of isolating these cells without destroying the embryo would be advantageous. In 2006, Chung and colleagues were the first authors to report the generation of mouse embryonic stem cell lines in this manner. Their method was based on a technique used to obtain a single cell embryo biopsy for preimplantation genetic diagnosis. Cells were taken from eight-cell blastomeres rather than from blastocysts. The cells differentiated into derivatives of all three embryonic germ layers in vitro, and as well as into teratomas in vivo. In addition, the mouse embryos that resulted from the biopsied blastomeres developed to term without a reduction in their developmental potential.
Obtaining Cells from Arrested Embryos
hES cell lines can also be derived from arrested embryos (Zhang et al, 2006a). During in vitro fertilization, only a small proportion of zygotes produced will develop successfully to the morula and blastocyst stages. Over half the embryos stop dividing (Hardy, 1993; Geber et al, 1999) and are, therefore, considered dead embryos (Landry et al, 2004). Such embryos have unequal or fragmented cells and blastomeres and are usually discarded. Not all the cells within these arrested embryos, however, are abnormal (Martinez et al, 2002; Zhang et al, 2006a), and these embryos might be a source of hES cells. More studies are needed to characterize the full proliferation and differentiation potential of embryonic stem cells derived from arrested embryos.
Altered Nuclear Transfer
Altered nuclear transfer is a variation of somatic cell nuclear transfer (SCNT) in which a genetically modified nucleus from a somatic cell is transferred into a human oocyte. This embryo, which contains a deliberate genetic defect, is capable of developing into a blastocyst, but the induced defect prevents the blastocyst from implanting in the uterus. This process has the potential to generate customized hES cells from the blastocyst stage (Hurlbut, 2005). Human embryos with this genetic defect might lack the capacity to develop into viable fetuses, as a result of their inability to implant, thus providing a source of stem cells without destroying viable embryos. Proof of concept was obtained in mice by Meissner and Jaenisch (2006) by using embryos lacking the CDX2 homeobox gene.
The viability of human embryos lacking the CDX2 gene is unclear, as is whether this mutation restricts human developmental potential into certain lineages. Although much research must be done before therapeutic strategies based on this technique could ever enter the clinic, at this time, hES cells derived from altered nuclear transfer can provide opportunities to study pluripotency in hES cells without the need for destruction of viable embryos. The exact effects of CDX2 gene knockout on the development of human embryos are not well known. The effects of this gene however, have been thoroughly investigated in the gastric and intestinal epithelium (Benahmed et al, 2008; Vauhkonen et al, 2008).
Therapeutic Cloning (Somatic Cell Nuclear Transfer)
At this point, it is extremely important to differentiate between the two types of cloning that exist: reproductive cloning and therapeutic cloning. Both involve the insertion of donor deoxyribonucleic acid (DNA) into an enucleated oocyte to generate an embryo that has identical genetic material to its DNA source. However, the similarities end there. In reproductive cloning, the embryo is then implanted into the uterus of a pseudopregnant female to produce an infant that is a clone of the donor. A world-famous example of this type of cloning resulted in the birth of a sheep named Dolly in 1997 (Wilmut et al, 1997). However, there are many ethical concerns surrounding such practices, and as a result, reproductive cloning has been banned in most countries.
Although therapeutic cloning also produces an embryo that is genetically identical to the donor, this process is used to generate blastocysts that are explanted and grown in culture, rather than in utero. Embryonic stem cell lines can then be derived from these blastocysts, which are only allowed to grow to a 100-cell stage. At this time the inner cell mass is isolated and cultured, resulting in ES cells that are genetically identical to the patient. This process is detailed in Fig. 19–1. It has been shown that nuclear-transferred ES cells derived from fibroblasts, lymphocytes, and olfactory neurons are pluripotent and can generate live pups after injection into blastocysts. This shows that cells generated by SCNT have the same developmental potential as blastocysts that are fertilized and produced naturally (Hochedlinger et al, 2002; Eggan et al, 2004; Brambrink et al, 2006). In addition, the ES cells generated by SCNT are perfectly matched to the patient’s immune system, and no immunosuppressants would be required to prevent rejection should these cells be used in regenerative medicine applications.
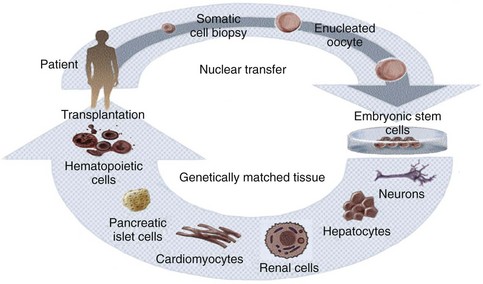
Figure 19–1 Therapeutic cloning strategy and its application to the engineering of tissues and organs.
Although ES cells derived from SCNT contain the nuclear genome of the donor cells, mitochondrial DNA (mtDNA) contained in the oocyte could lead to immunogenicity after transplantation. To assess the histocompatibility of tissue generated using SCNT, Lanza and colleagues (2002b) microinjected the nucleus of a bovine skin fibroblast into an enucleated oocyte. Although the blastocyst was implanted (reproductive cloning), the purpose was to generate renal, cardiac and skeletal muscle cells, which were then harvested, expanded in vitro, and seeded onto biodegradable scaffolds. These scaffolds were then implanted into the donor steer from which the cells were cloned to determine if cells were histocompatible. Analysis revealed that cloned renal cells showed no evidence of T-cell response, suggesting that rejection will not necessarily occur in the presence of oocyte-derived mtDNA. This finding represents a step forward in overcoming the histocompatibility problem of stem cell therapy.
Although promising, SCNT has certain limitations that require further improvement before its clinical application, in addition to the ethical considerations regarding the potential of the resulting embryos to develop into cloned embryos if implanted into a uterus. In addition, to date this technique has not been shown to work in humans. The initial failures and fraudulent reports of nuclear transfer in humans reduced enthusiasm for human applications (Simerly et al, 2003; Hwang et al, 2004; Hwang et al, 2005), although it was recently reported that nonhuman primate ES cell lines were generated by SCNT using nuclei from adult skin fibroblasts (Byrne et al, 2007; Mitalipov, 2007).
Reprogramming (Induced Pluripotent Stem Cells)
Recently, reports of the successful transformation of adult cells into pluripotent stem cells through a type of genetic “reprogramming” have been published. Reprogramming is a technique that involves de-differentiation of adult somatic cells to produce patient-specific pluripotent stem cells, eliminating the need to create embryos. Cells generated by reprogramming would be genetically identical to the somatic cells (and thus, the patient who donated these cells) and would not be rejected. Yamanaka’s group was the first to discover that mouse embryonic fibroblasts (MEFs) and adult mouse fibroblasts could be reprogrammed into an “induced pluripotent state” (iPS) (Takahashi and Yamanaka, 2006). These iPS cells possessed the immortal growth characteristics of self-renewing ES cells—expressed genes specific for ES cells, and generated embryoid bodies in vitro and teratomas in vivo. When iPS cells were injected into mouse blastocysts, they contributed to a variety of cell types. However, although iPS cells selected in this way were pluripotent, they were not identical to ES cells. Unlike ES cells, chimeras made from iPS cells did not result in full-term pregnancies. Gene expression profiles of the iPS cells showed that they possessed a distinct gene expression signature that was different from that of ES cells. In addition, the epigenetic state of the iPS cells was somewhere between that found in somatic cells and that found in ES cells, suggesting that the reprogramming was incomplete.
These results were improved significantly by Wernig and colleagues in July 2007 (Wernig et al, 2007). In this study, DNA methylation, gene expression profiles, and the chromatin state of the reprogrammed cells were similar to those of ES cells. Teratomas induced by these cells contained differentiated cell types representing all three embryonic germ layers. Most importantly, the reprogrammed cells from this experiment were able to form viable chimeras and contribute to the germ line like ES cells, suggesting that these iPS cells were completely reprogrammed.
It has recently been shown that reprogramming of human cells is possible (Yu et al, 2007). Yamanaka’s group generated human iPS cells that are similar to hES cells in terms of morphology, proliferation, gene expression, surface markers, and teratoma formation. These were generated via retroviral transduction of the stem cell markers OCT4, SOX2, NANOG, and LIN28 (Takahashi et al, 2007). However, in both studies, the human iPS cells were similar but not identical to hES cells. Although reprogramming is an exciting phenomenon, our limited understanding of the mechanism underlying it currently limits the clinical applicability of the technique, but the future potential of reprogramming is quite exciting.
Amniotic Fluid– and Placenta-Derived Stem Cells
The amniotic fluid and placental membrane contain a heterogeneous population of cell types derived from the developing fetus (Priest et al, 1978; Polgar et al, 1989). Cells found in this heterogeneous population include mesenchymal stem cells (in ’t Anker et al, 2003b; Tsai et al, 2004). In addition, the isolation of multipotent human and mouse amniotic fluid– and placenta-derived stem (AFPS) cells that are capable of extensive self-renewal and give rise to cells from all three germ layers was reported in 2007 (De Coppi et al, 2007a). AFPS cells represent approximately 1% of the cells found in the amniotic fluid and placenta. The undifferentiated stem cells expand extensively without a feeder cell layer and double every 36 hours. Unlike human embryonic stem cells, the AFPS cells do not form tumors in vivo. Lines maintained for over 250 population doublings retained long telomeres and a normal complement of chromosomes. AFPS cell lines can be induced to differentiate into cells representing each embryonic germ layer, including cells of adipogenic, osteogenic, myogenic, endothelial, neural-like and hepatic lineages. In addition to the differentiated AFPS cells expressing lineage-specific markers, such cells can have specialized functions. Cells of the hepatic lineage secreted urea and α-fetoprotein, while osteogenic cells produced mineralized calcium. In this respect, they meet a commonly accepted criterion for multipotent stem cells, without implying that they can generate every adult tissue.
Since the discovery of the AFPS cells, other groups have published on the potential of the cells to differentiate to other lineages, such as cartilage (Kolambkar et al, 2007), kidney (Perin et al, 2007), and lung (Warburton et al, 2008). Muscle differentiated AFPS cells were also noted to prevent compensatory bladder hypertrophy in a cryoinjured rodent bladder model (De Coppi et al, 2007b).
Adult Stem Cells
Adult stem cells, especially hematopoietic stem cells, are the best understood cell type in stem cell biology (Ballas et al, 2002). However, adult stem cell research remains an area of intense study, because their potential for therapy may be applicable to a myriad of degenerative disorders. Within the past decade, adult stem cell populations have been found in many adult tissues other than the bone marrow and the gastrointestinal tract, including the brain (Taupin, 2006), skin (Jensen et al, 2008; Jiao et al, 2008), and muscle (Crisan et al, 2008). Many other types of adult stem cells have been identified in organs all over the body and are thought to serve as the primary repair entities for their corresponding organs (Weiner, 2008) (Fig. 19–2). The discovery of such tissue-specific progenitors has opened up new avenues for research.
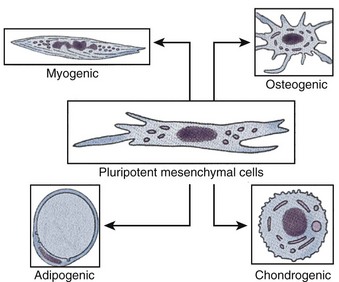
Figure 19–2 Schematic diagram of pluripotential stem cell lineages that can be derived from postnatal tissue.
A notable exception to the tissue specificity of adult stem cells is the mesenchymal stem cell, also known as the multipotent adult progenitor cell. This cell type is derived from bone marrow stroma (Devine, 2002; Jiang et al, 2002). Such cells can differentiate in vitro into numerous tissue types (Caplan, 2007; da Silva Meirelles et al, 2008) and can also differentiate developmentally if injected into a blastocyst. Multipotent adult progenitor cells can develop into a variety of tissues including neuronal (Duan et al, 2007), adipose (Crisan et al, 2008), muscle (Crisan et al, 2008; Luttun et al, 2006), liver (Ikeda et al, 2008; Mimeault et al, 2008), lungs (Nolen-Walston et al, 2008), spleen (in ’t Anker et al, 2003a), and gut tissue (Jiang et al, 2002), but notably not bone marrow or gonads.
Research into adult stem cells has, however, progressed slowly, mainly because investigators have had great difficulty in maintaining adult nonmesenchymal stem cells in culture. Some cells, such as those of the liver, pancreas, and nerve, have very low proliferative capacity in vitro, and the functionality of some cell types is reduced after the cells are cultivated. Isolation of cells has also been problematic, because stem cells are present in extremely low numbers in adult tissue (Hristov et al, 2008; Mimeault et al, 2008). Although the clinical utility of adult stem cells is currently limited, great potential exists for future use of such cells in tissue-specific regenerative therapies. The advantage of adult stem cells is that they can be used in autologous therapies, thus avoiding any complications associated with immune rejection.
Native Targeted Progenitor Cells
In the past, one of the limitations of applying cell-based regenerative medicine techniques to organ replacement was the inherent difficulty of growing certain human cell types in large quantities. Native targeted progenitor cells, or native cells, are tissue specific unipotent cells derived from most organs. The advantage of these cells is that they are already programmed to become the cell type needed, without any extralineage differentiation. By noting the location of the progenitor cells, as well as by exploring the conditions that promote differentiation and/or self-renewal, it has been possible to overcome some of the obstacles that limit cell expansion in vitro. One example is the urothelial cell. Urothelial cells could be grown in the laboratory setting in the past, but only with limited success. It was believed that urothelial cells had a natural senescence that was hard to overcome. Several protocols have been developed over the last two decades that have improved urothelial growth and expansion (Cilento et al, 1994; Liebert et al, 1997; Scriven et al, 1997; Puthenveettil et al, 1999). A system of urothelial cell harvesting was developed that does not use any enzymes or serum and has a large expansion potential. Using these methods of cell culture, it is possible to expand an urothelial strain from a single specimen that initially covers a surface area of 1 cm2 to one covering a surface area of 4202 m2 (the equivalent area of one football field) within 8 weeks (Cilento et al, 1994).
An additional advantage in using native cells is that they can be obtained from the specific organ to be regenerated, expanded, and used in the same patient without rejection, in an autologous manner (Atala et al, 1992b, 1993b, 1993c, 1994, 1995b, 1997, 1998; Cilento et al, 1994; Yoo et al, 1997; Fauza et al, 1998a, 1998b; Machluf et al, 1998; Yoo et al, 1998b; Amiel et al, 1999; Kershen et al, 1999; Oberpenning et al, 1999; Park et al, 1999).
Bladder, ureter, and renal pelvis cells can equally be harvested, cultured, and expanded in a similar fashion (Fig. 19–3). Normal human bladder epithelial and muscle cells can be efficiently harvested from surgical material, extensively expanded in culture, and their differentiation characteristics, growth requirements, and other biologic properties can be studied (Liebert et al, 1991; Cilento et al, 1994; Tobin et al, 1994; Harriss, 1995; Freeman et al, 1997; Liebert et al, 1997; Fauza et al, 1998a, 1998b; Lobban et al, 1998; Solomon et al, 1998; Nguyen et al, 1999; Puthenveettil et al, 1999; Rackley et al, 1999). Major advances in cell culture techniques have been made within the past decade, and these techniques make the use of autologous cells possible for clinical application. However, even now, not all human cells can be grown or expanded in vitro. Liver, nerve, and pancreas are examples of human tissues where the technology is not yet advanced to the point where these cells can be grown and expanded.
When cells are used for tissue reconstitution, donor tissue is dissociated into individual cells that are either implanted directly into the host or expanded in culture, then attached to a support matrix or reimplanted after expansion. The implanted tissue can be heterologous, allogeneic, or autologous. Ideally, this approach allows lost tissue function to be restored or replaced in toto, and with limited complications (Atala, 1997). Native cells and tissues are usually preferable for reconstruction. In most cases, the replacement of lost or deficient tissues with functionally equivalent cells and tissues would improve the outcome for these patients. This goal may be attainable with the use of regenerative medicine techniques.
Biomaterials for Genitourinary Regenerative Medicine
Synthetic materials have been used widely for urologic reconstruction. The most common type of synthetic prostheses for urologic applications is made of silicone. Silicone prostheses have been used for the treatment of urinary incontinence with the artificial urinary sphincter and detachable balloon system, for treatment of vesicoureteral reflux with silicone microparticles, and for impotence with penile prostheses (Atala et al, 1992a; Riehmann et al, 1993; Levesque et al, 1996; Buckley et al, 1997). There has also been a major effort directed toward the construction of artificial bladders made with silicone. In some disease states, such as urinary incontinence or vesicoureteral reflux, artificial agents (Teflon paste, glass microparticles) have been used as injectable bulking substances; however, these substances are not entirely biocompatible (Atala, 1994).
For regenerative medicine purposes, there are clear advantages in using degradable, biocompatible materials that can function as cell delivery vehicles, and/or provide the structural parameters needed for tissue replacement. Biomaterials in genitourinary regenerative medicine function as an artificial extracellular matrix (ECM) and elicit biologic and mechanical functions of native ECM found in tissues in the body. Native ECM brings cells together into tissue, controls the tissue structure, and regulates the cell phenotype (Alberts et al, 1994). Biomaterials facilitate the localization and delivery of cells and/or bioactive factors (e.g., cell adhesion peptides, growth factors) to desired sites in the body; define a three-dimensional space for the formation of new tissues with appropriate structure; and guide the development of new tissues with appropriate function (Kim et al, 1998). Direct injection of cell suspensions without biomaterial matrices has been used in some cases (Ponder et al, 1991; Brittberg et al, 1994), but it is difficult to control the localization of transplanted cells. In addition, the majority of mammalian cell types is anchorage-dependent and will die if not provided with a cell-adhesion substrate.
Design and Selection of Biomaterials
The selected biomaterial should be biodegradable and bioresorbable to support the reconstruction of a completely normal tissue without inflammation. Such behavior of the biomaterials avoids the risk of inflammatory or foreign-body responses that may be associated with the permanent presence of a foreign material in the body. The degradation rate and the concentration of degradation products in the tissues surrounding the implant must be at a tolerable level (Bergsma et al, 1995).
The biomaterials should provide an appropriate regulation of cell behavior (e.g., adhesion, proliferation, migration, differentiation) to promote the development of functional new tissue. Cell behavior in engineered tissues is regulated by multiple interactions with the microenvironment, including interactions with cell-adhesion ligands (Hynes, 1992) and with soluble growth factors (Deuel, 1997). Cell adhesion–promoting factors (e.g., arg-gly-asp [RGD]) can be presented by the biomaterial itself or incorporated into the biomaterial to control cell behavior through ligand-induced cell receptor signaling processes (Barrera et al, 1993; Cook et al, 1997). The biomaterials provide temporary mechanical support sufficient to withstand in vivo forces exerted by the surrounding tissue and maintain a potential space for tissue development. The mechanical support of the biomaterials should be maintained until the engineered tissue has sufficient mechanical integrity to support itself (Atala, 2007). This potentially can be achieved by an appropriate choice of mechanical and degradative properties of the biomaterials (Kim et al, 1998).
The biomaterials need to be processed into specific configurations. A large ratio of surface area to volume is often desirable to allow the delivery of a high density of cells. A high-porosity, interconnected pore structure with specific pore sizes promotes tissue ingrowth from the surrounding host tissue. Several techniques, such as electrospinning, have been developed that readily control porosity, pore size, and pore structure (Lee et al, 2007; Yoo et al, 2007; Choi et al, 2008; Lee et al, 2008a, 2008b, 2008c).
Types of Biomaterials
Generally, three classes of biomaterials have been used for engineering of genitourinary tissues: naturally derived materials, such as collagen and alginate; acellular tissue matrices, such as bladder submucosa and small-intestinal submucosa; and synthetic polymers, such as polyglycolic acid (PGA), polylactic acid (PLA), and poly(lactic-co-glycolic acid) (PLGA). These classes of biomaterials have been tested in regard to their biocompatibility with primary human urothelial and bladder muscle cells (Pariente et al, 2001). Naturally derived materials and acellular tissue matrices have the potential advantage of biologic recognition. Synthetic polymers can be produced reproducibly on a large scale with controlled properties of strength, degradation rate, and microstructure.
Collagen is the most abundant and ubiquitous structural protein in the body, and it may be readily purified from both animal and human tissues with an enzyme treatment and salt/acid extraction (Li, 1995). Collagen has long been known to exhibit minimal inflammatory and antigenic responses (Furthmayr et al, 1976), and it has been approved by the U.S. Food and Drug Administration (FDA) for many types of medical applications, including wound dressings and artificial skin (Cen et al, 2008). Intermolecular cross-linking reduces the degradation rate by making the collagen molecules less susceptible to an enzymatic attack. Intermolecular cross-linking can be accomplished by various physical (e.g., ultraviolet radiation, dehydrothermal treatment) or chemical (e.g., glutaraldehyde, formaldehyde, carbodiimides) techniques (Li, 1995). Collagen contains cell-adhesion domain sequences (e.g., RGD) that exhibit specific cellular interactions. This may help to retain the phenotype and activity of many types of cells, including fibroblasts (Silver et al, 1992) and chondrocytes (Sams et al, 1995). This material can be processed into a wide variety of structures such as sponges (Fig. 19–4, top) fibers, and films (Yannas et al, 1980a, 1980b; Cavallaro et al, 1994).
Alginate, a polysaccharide isolated from seaweed, has been used as an injectable cell delivery vehicle (Smidsrod et al, 1990) and a cell immobilization matrix (Lim and Sun, 1980) owing to its gentle gelling properties in the presence of divalent ions such as calcium. Alginate is a family of copolymers of D-mannuronate and L-guluronate. The physical and mechanical properties of alginate gel are strongly correlated with the proportion and length of the polyguluronate block in the alginate chains (Smidsrod et al, 1990). Efforts have been made to synthesize biodegradable alginate hydrogels with mechanical properties that are controllable in a wide range by intermolecular covalent cross-linking and with cell-adhesion peptides coupled to their backbones (Rowley et al, 1999).
Recently, natural materials such as alginate and collagen have been used as “bio-inks” in a newly developed bioprinting technique based on inkjet technology (Boland et al, 2006; Campbell et al, 2007). Using this technology, these scaffold materials can be “printed” into a desired scaffold shape using a modified inkjet printer. In addition, several groups have shown that living cells can also be printed using this technology (Laflamme et al, 2005; Nakamura et al, 2005). This exciting technique can be modified so that a three-dimensional construct containing a precise arrangement of cells, growth factors, and extracellular matrix material can be printed (Roth et al, 2004; Ilkhanizadeh et al, 2007; Xu et al, 2009). Such constructs may eventually be implanted into a host to serve as the backbone for a new tissue or organ.
Acellular tissue matrices are collagen-rich matrices prepared by removing cellular components from tissues (Fig. 19–4, center). The matrices are often prepared by mechanical and chemical manipulation of a segment of bladder tissue (Dahms et al, 1998; Piechota et al, 1998; Yoo et al, 1998b; Chen et al, 1999). The matrices slowly degrade after implantation and are replaced and remodeled by ECM proteins synthesized and secreted by transplanted or ingrowing cells. Acellular tissue matrices have been proved to support cell ingrowth and regeneration of genitourinary tissues, including urethra and bladder, with no evidence of immunogenic rejection (Probst et al, 1997; Chen et al, 1999). Because the structures of the proteins (e.g., collagen, elastin) in acellular matrices are well conserved and normally arranged, the mechanical properties of the acellular matrices are not significantly different from those of native bladder submucosa (Dahms et al, 1998).
Polyesters of naturally occurring α-hydroxy acids, including PGA, PLA, and PLGA, are widely used in regenerative medicine. These polymers have gained FDA approval for human use in a variety of applications, including sutures (Gilding, 1981). The degradation products of PGA, PLA, and PLGA are nontoxic, natural metabolites that are eventually eliminated from the body in the form of carbon dioxide and water (Gilding, 1981). Because these polymers are thermoplastics, they can easily be formed into a three-dimensional scaffold with a desired microstructure, gross shape, and dimension by various techniques, including molding, extrusion (Freed et al, 1994), solvent casting (Mikos et al, 1994), phase separation techniques, and gas foaming techniques (Harris et al, 1998). More recently, techniques such as electrospinning have been used to quickly create highly porous scaffolds in various conformations (Han et al, 2006; Choi et al, 2008; Lee et al, 2008a; Lee et al, 2008b).
Many applications in genitourinary regenerative medicine require a scaffold with high porosity and a high ratio of surface area to volume. This need has been addressed by processing biomaterials into configurations of fiber meshes (Fig. 19–4, bottom) and porous sponges using the techniques described previously. A drawback of the synthetic polymers is lack of biologic recognition. As an approach toward incorporating cell recognition domains into these materials, copolymers with amino acids have been synthesized (Barrera et al, 1993; Intveld et al, 1994; Cook et al, 1997). Other biodegradable synthetic polymers, including polyanhydrides and polyortho-esters), can also be used to fabricate scaffolds for genitourinary regenerative medicine with controlled properties (Peppas et al, 1994).
Nanotechnology, the ability to use small molecules that have distinct properties in a small scale, has been used to create “smart” biomaterials for regenerative medicine (Boccaccini et al, 2005; Harrison et al, 2007). Nanoscaffolds have been manufactured specifically for bladder applications (Harrington et al, 2006). The manufacturing of biomaterials can also lead to enhanced cell alignment and tissue formation (Choi et al, 2008).
Vascularization
The goals in regenerative medicine include the replacement of damaged, injured or missing body tissues with biological compatible substitutes. A limiting factor for the engineering of tissues is that cells cannot be implanted in volumes exceeding 3 mm3 (Folkman et al, 1973). Nutrition and gas exchange is limited by this maximal diffusion distance. If cells were implanted in volumes exceeding 3 mm3, only the cells on the surface would survive, and the central cell core would undergo necrosis resulting from a lack of vascularity. Therefore a critical obstacle in regenerative medicine is the ability to maintain large masses of cells alive upon transfer from the in vitro culture conditions into the host (in vivo) (Mooney et al, 1999). To achieve the goals of engineering large complex tissues, and possibly internal organs, vascularization of the regenerating cells is essential.
Formation of new blood vessels and capillaries comprises two different processes: vasculogenesis, the in-situ assembly of capillaries from undifferentiated endothelial cells (EC), and angiogenesis, the sprouting of capillaries from preexisting blood vessels. The formation of the first capillaries takes place mostly during the early stages of embryogenesis (Folkman et al, 1996; Yancopoulos et al, 1998).
Vasculogenesis can be divided into five consecutive steps: (1) EC are generated from precursor cells, called angioblasts, in the bone marrow; (2) EC form the vessel primordia and aggregates that establish cell-to-cell contact but have no lumen; (3) a nascent endothelial tube is formed, composed of polarized EC; (4) a primary vascular network is formed from an array of nascent endothelial tubes; and (5) pericytes and vascular smooth muscle cells are recruited (Drake et al, 1998).
Angiogenesis is a morphogenic process, which describes the formation of new blood capillaries from EC of preexisting vessels. There are six basic steps in angiogenesis: (1) vasodilatation of the parental vessel, reducing the contact between adjacent EC, (2) degradation of the basement membrane of a parental vessel by secretion and activation of a wide range of proteolytic enzymes, (3) EC migration and proliferation to form a leading edge of the new capillary, (4) generation of the capillary lumen and formation of a tubelike structure, (5) basement membrane synthesis, and (6) recruitment of pericytes and vascular smooth muscle cells (Folkman et al, 1992).
When converting to the angiogenic stage, EC will capture new properties that enable them to neovascularize the tissue (Hanahan et al, 1996). When the new vessels are in place and the vascular network matures, the neovascular EC resume their quiescent phenotype (Darland et al, 1999). Several growth factors serve as stimuli for EC conversion to the angiogenic phenotype (Battegay, 1995). Vascular endothelial growth factor (VEGF) and fibroblast growth factor (FGF) are two well-characterized and important angiogenic molecules that have a direct effect on EC.
The understanding of the angiogenic process and the isolation of potent and specific angiogenic growth factors has encouraged the use of these factors therapeutically (Loges et al, 2009; Phelps et al, 2009). Efforts have been aimed at incorporating the knowledge acquired in angiogenesis of ischemic tissues into practical approaches to vascularize bioengineered tissues (Stosich et al, 2009). Bioengineered tissues are usually supported by scaffolds of biocompatible matrices made from natural or artificial sources (Hubbell et al, 1991). Successful vascularization is dependent on the porosity of the supporting matrix. A positive correlation between the pore size of poly-L-lactic acid (PLLA) implants and the rate of vascularization has been observed (Mikos et al, 1993).
Three approaches have been used for vascularization of bioengineered tissue: (1) incorporation of angiogenic factors in the bioengineered tissue, (2) seeding EC with other cell types in the bioengineered tissue, and (3) prevascularization of the matrix prior to cell seeding. Angiogenic growth factors may be incorporated into the bioengineered tissue before implantation, to attract host capillaries and to enhance neovascularization of the implanted tissue. Angiogenic growth factors can be embedded in specific biomaterials, and can be controlled for slow release (Eiselt et al, 1998). Cells can also be genetically engineered to secrete high levels of angiogenic proteins (Springer et al, 1998).
Another approach for enhancing angiogenesis employed cultured EC, which are incorporated into the bioengineered tissue prior to implantation. Human penile corpus cavernosum–derived smooth muscle cells and EC were seeded on biodegradable polymer scaffolds, in order to reconstruct penile corporeal tissue in vitro and in vivo (Park et al, 1999). The use of EC improved the formation of the engineered tissue. In another study, the addition of both EC and angiogenic growth factors (VEGF) accelerated the formation of engineered muscle tissue (De Coppi et al, 2005). An alternative direction in vascularization of bioengineered tissue is the prevascularization of the supporting polymer prior to cell seeding. In this manner, the bioengineered tissue will be organized around the vascular network, providing sufficient tissue perfusion (Fontaine et al, 1995).
Regenerative Medicine of Urologic Structures
Urethra
Various strategies have been proposed over the years for the regeneration of urethral tissue. Woven meshes of PGA without cells (Bazeed et al, 1983; Olsen et al, 1992) or with cells (Atala et al, 1992b) were used to regenerate urethras in various animal models. Naturally derived collagen-based materials, such as bladder-derived acellular submucosa (Chen et al, 1999), and an acellular urethral submucosa (Sievert et al, 2000), have also been tried experimentally in various animal models for urethral reconstruction.
The bladder submucosa matrix (Chen et al, 1999), proved to be a suitable graft for repair of urethral defects in rabbits. The neourethras demonstrated a normal urothelial luminal lining and organized muscle bundles. These results were confirmed clinically in a series of patients with a history of failed hypospadias reconstruction wherein the urethral defects were repaired with human bladder acellular collagen matrices (Atala et al, 1999). The neourethras were created by anastomosing the matrix in an onlay fashion to the urethral plate. The size of the created neourethra ranged from 5 to 15 cm. After a 3-year follow-up, three of the four patients had a successful outcome in regard to cosmetic appearance and function (Fig. 19–5). One patient who had a 15-cm neourethra created developed a subglanular fistula. The acellular collagen-based matrix eliminated the necessity of performing additional surgical procedures for graft harvesting, and both operative time and the potential morbidity from the harvest procedure were decreased. Similar results were obtained in pediatric and adult patients with primary urethral stricture disease using the same collagen matrices (El-Kassaby et al, 2003). Another study in 30 patients with recurrent stricture disease showed that a healthy urethral bed (two or fewer prior urethral surgeries) was needed for successful urethral reconstruction using the acellular collage-based grafts (El Kassaby et al, 2008). More than 200 pediatric and adult patients with urethral disease have been successfully treated in an onlay manner with a bladder-derived collagen-based matrix. One of its advantages over nongenital tissue grafts used for urethroplasty is that the material is “off the shelf.” This eliminates the necessity of additional surgical procedures for graft harvesting, which may decrease operative time as well as the potential morbidity due to the harvest procedure.
The above techniques, using nonseeded acellular matrices, were applied experimentally and clinically in a successful manner for onlay urethral repairs. However, when tubularized urethral repairs were attempted experimentally, adequate urethral tissue regeneration was not achieved, and complications ensued, such as graft contracture and stricture formation (De Filippo et al, 2002). Autologous rabbit bladder epithelial and smooth muscle cells were grown and seeded onto preconfigured tubular matrices. Entire urethra segments were resected and urethroplasties were performed with tubularized collagen matrices, either seeded with cells or without cells. The tubularized collagen matrices seeded with autologous cells formed new tissue that was histologically similar to native urethra. The tubularized collagen matrices without cells led to poor tissue development, fibrosis, and stricture formation. These findings were confirmed clinically. A clinical trial using tubularized nonseeded small intestinal submucosa (SIS) for urethral stricture repair was performed with eight evaluable patients. Two patients with short inflammatory strictures maintained urethral patency. Stricture recurrence developed in the other six patients within 3 months of surgery (le Roux, 2005). Other cell types have also been tried experimentally in acellular bladder collagen matrices, including foreskin epidermal cells and oral keratinocytes (Fu et al, 2007; Li et al, 2008). Vascular endothelial growth factor gene–modified urothelial cells have also been used experimentally for urethral reconstruction (Guan et al, 2008).
The normal wound-healing response to injury has been studied extensively, and this knowledge has been helpful in maximizing success for the engineering of tissues. At the time of tissue injury, cell ingrowth is initiated from the wound edges to cover the tissue defect. The cells from the edges of the native tissue are able to traverse short distances without any detrimental effects. If the wound is large, more than a few millimeters in distance or depth, increased collagen deposition, fibrosis, and scar formation ensue. Matrices implanted in wound beds are able to lengthen the distances that cells can traverse without initiating an adverse fibrotic response. However, these distances are also limited. The maximum distance that adjacent cells from the wound edge have to travel to create normal tissue over a biologic matrix is approximately 1 cm (Dorin et al, 2008). Tissue defects greater than 1 cm, which are treated with a matrix alone (without cells), usually have increased collagen deposition, increased fibrosis, and scar formation. Cell-seeded matrices implanted in wound beds are able to further lengthen the distance for normal tissue formation without initiating an adverse fibrotic response. Studies in the field of regenerative medicine have shown that very large defects (>30 cm), can be successfully treated using cell-seeded scaffolds. This explains the described experimental and clinical results noted with urethral repair. Nonseeded matrices are able to replace urethral segments when used in an onlay fashion, because of the short distances required for tissue ingrowth. However, if a tubularized urethral repair is needed, the matrices need to be seeded with autologous cells in order to avoid the risk of stricture formation and poor tissue development.
Bladder
Currently, gastrointestinal segments are commonly used as tissues for bladder replacement or repair. However, gastrointestinal tissues are designed to absorb specific solutes, whereas bladder tissue is designed for the excretion of solutes. When gastrointestinal tissue is in contact with the urinary tract, multiple complications may ensue, such as infection, metabolic disturbances, urolithiasis, perforation, increased mucus production, and malignancy (McDougal, 1992; Atala et al, 1993a; Kaefer et al, 1997, 1998). Because of the problems encountered with the use of gastrointestinal segments, numerous investigators have attempted alternative reconstructive procedures for bladder replacement or repair. These include autoaugmentation (Cartwright et al, 1989a, 1989b) (Fig. 19–6) and ureterocystoplasty (Bellinger, 1993; Churchill et al, 1993; Adams et al, 1998). In addition, alternative methods for bladder reconstruction have been explored, such as the use of tissue expansion and regenerative medicine with cell transplantation.
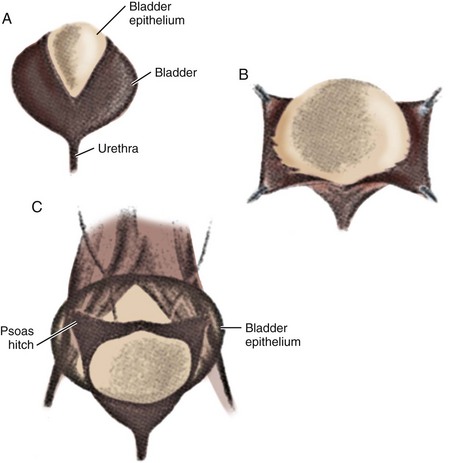
(From Cartwright PC, Snow BW. Bladder autoaugmentation: early clinical experience. J Urol 1989;142:505.)
Tissue Expansion for Bladder Augmentation
A system of progressive dilation for ureters and bladders has been proposed but has not been attempted clinically (Lailas et al, 1996; Satar et al, 1999). In an animal experiment, rabbits underwent unilateral ureteral ligation at the ureterovesical junction. A catheter was threaded into the proximal ipsilateral ureter and connected to an injection port that was secured subcutaneously. A saline-antibiotic solution was injected daily subcutaneously into the injection port. After 1 month of daily saline-antibiotic–solution injections, the ureteral units were dilated at least 10-fold, and the diameter exceeded that of adjacent colon in each instance (Fig. 19–7). Augmentation cystoplasty was performed with the reconfigured dilated ureteral segment, resulting in an increased bladder capacity ranging from 190% to 380% (Lailas et al, 1996). In a similar system, a dilating indwelling catheter was used to dilate ureteral tissue in pigs (Ikeguchi et al, 1998).
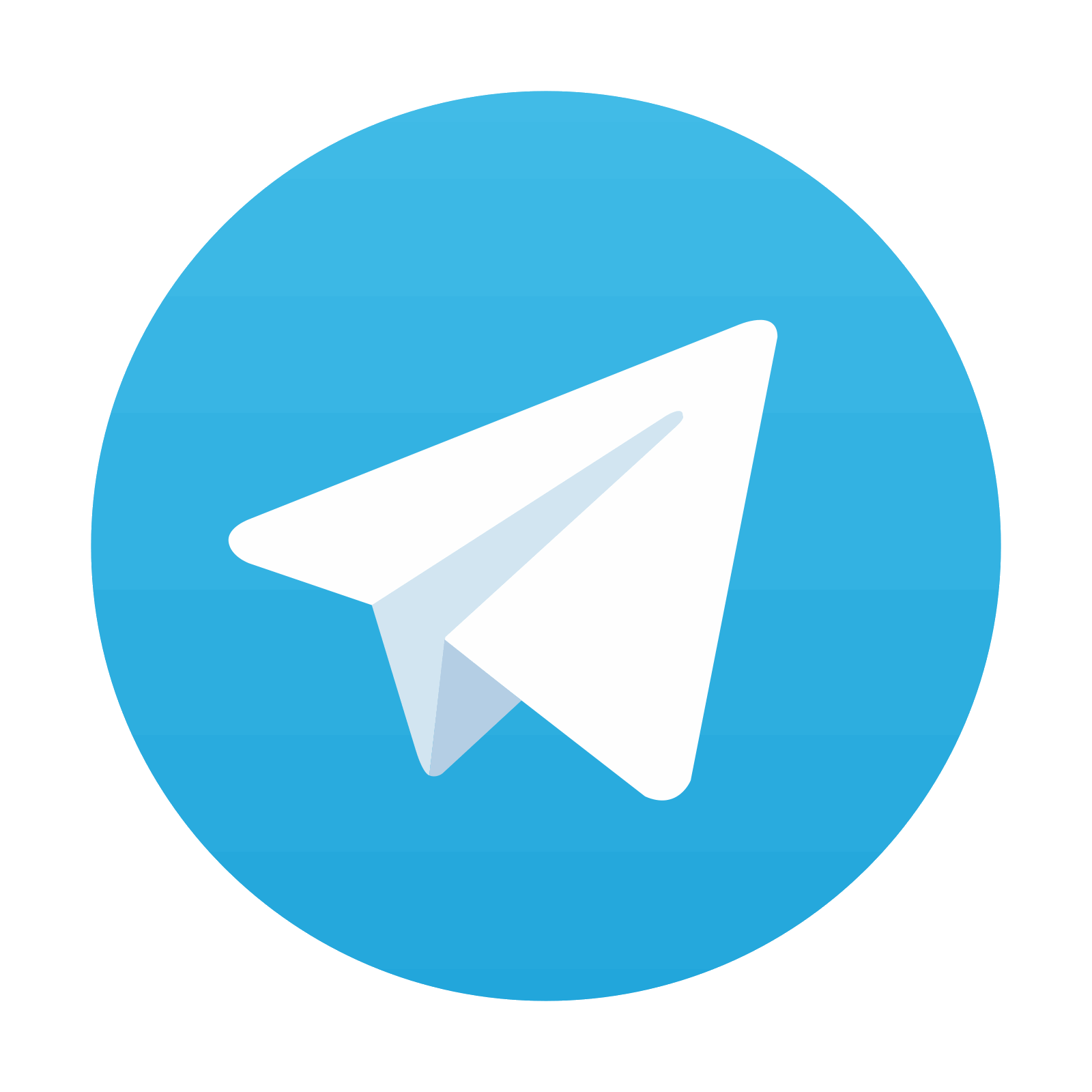
Stay updated, free articles. Join our Telegram channel
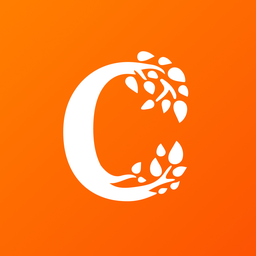
Full access? Get Clinical Tree
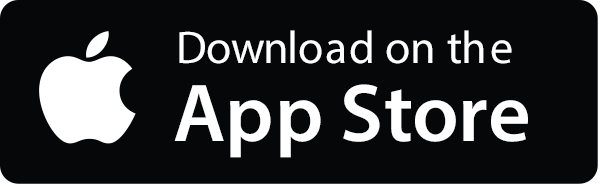
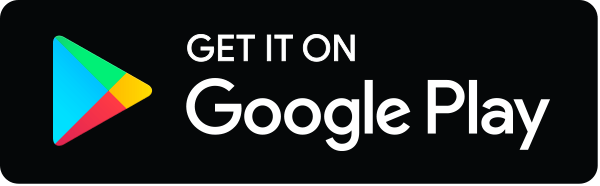