Chapter 18 Pinaki R. Dutta, MD, PhD; Matthew Biagioli, MD, MS; Neha Vapiwala, MD In light of excellent disease control achievable with a variety of different treatment modalities, the optimal role of radiation in the curative and palliative management of genitourinary malignancies is one of the more high-profile topics in clinical oncology. Radiation may be used alone as curative treatment of some tumors or as an adjuvant to surgery or in combination with systemic or other chemotherapy. It is also used as salvage local therapy for disease recurrence in the postoperative setting or as a palliative therapy to alleviate symptoms in patients with advanced or metastatic disease. The continued advancement in radiation technology has not only provided new tools to further improve the therapeutic index but has also raised important questions regarding its uses. Appropriate utilization of radiation requires knowledge of its mechanisms and techniques to fully understand the potential and the limitations of this modality. X-rays, gamma rays, electrons, and proton beams are types of electromagnetic energy used to treat cancer. First discovered in 1895 by Wilhelm Rontgen, x-rays were rapidly put to use to treat malignancy. In March of 1896, Emil Grubbe, a 21-year-old medical student, irradiated the chest wall of a woman with relapsed breast cancer and was the first to document a local response with radiation. In the early 1900s with the discovery of radium, tumors were implanted with gold wires infused with radium to deliver more focal radiation therapy. Decades later, with the advent of linear accelerators, cyclotrons, and complex computer algorithms, radiation dose can be conformed and adapted to “wrap around” the targeted structure and account for internal motion, all while avoiding critical normal tissues. Some forms of radiation can even be engineered to stop on a dime. Gamma rays are radiation produced by the decay of radioactive isotopes, either natural or man-made, whereas x-rays, or photons, are artificially produced when charged particles strike a target. Photons are typically produced in a linear accelerator in which electrons are accelerated to hit a tungsten target to emit the photons. When that tungsten target is removed from the path of the beam, electron beams are generated; funnellike applicators and collimators can be inserted to help shape the beam. Gamma rays have well-defined energies characteristic of the isotope that emits them, whereas x-rays have a broader spectrum that is determined by the energy of the machine that produced them. Protons are heavy charged particles that are accelerated to strike a target and that deliver their energy at a specific point in the tissue. Radiation dose delivered to the body is expressed in gray (Gy) or for protons in Gy (relative biologic effectiveness), abbreviated Gy(RBE). For photons, the energy of electromagnetic radiation determines the ability of radiation to penetrate tissue, with higher energies allowing deeper penetration. Higher energies scatter their radiation dose in a more forward direction. In high-energy machines, the maximum dose is deposited below the skin surface. For this reason, a low-energy machine operating at peak energy of 140,000 volts would be preferable in treating superficial tumors such as skin cancer because the maximum dose is deposited in the skin, and the dose decreases exponentially to less than 25% of the maximum dose at a depth of 4 cm. For tumors deeper in the body, a more penetrating beam from a linear accelerator, such as one operating at a peak energy of 10 million to 15 million volts (10-15 MV) is preferable. A 15-MV beam deposits its maximum energy at a depth of 2.8 cm and is still able to deliver 50% of its maximum dose at a depth of 20 cm. Unlike the higher energy photons, electrons deposit their maximum dose at or near the surface, penetrate to a predictable depth with a relatively constant dose, and then decrease rapidly. Protons, on the other hand, deliver their energy at the end of their Bragg peak. Like photons, the ability of the proton beam to penetrate deeper in the body depends on its energy. The main difference, however, lies in the exit dose. Photons continue to deliver small amounts of energy over a range. Therefore tissues beyond the target will also receive dose from photon irradiation. Because almost all of the energy in protons is delivered at the end of the Bragg peak, virtually no dose is delivered to tissues beyond that point. In some situations, it is preferable to deliver radiation to the tumor from an internal source. This technique is known as brachytherapy, meaning short-distance therapy. Radiation dose is determined by the ability of the radiation to penetrate tissue and the distance from the source of the radiation. Like visible light, the intensity of the radiation decreases in proportion to the square of the distance from the source (1/d2). Because doubling the distance from the source of radiation will decrease the intensity by 75% and tripling the distance will decrease the intensity by 89%, it is reasonable to consider placing the source of radiation within the tumor to minimize the dose that would be delivered to the surrounding normal tissue. Radioactive isotopes produce radiation with a decreasing dose rate as time passes, a process known as radioactive decay. Every isotope has a half-life, defined as the time required for the dose rate to decrease by 50%. Depending on the half-life, certain isotopes are used for either high–dose rate (HDR) brachytherapy or low-dose rate (LDR) brachytherapy. For HDR, an applicator or catheter is inserted into the target structure, and the radioactive isotope is inserted for a specific amount of time (usually a few minutes) and then removed. The procedure can be repeated as often as needed to deliver the adequate total dose. In LDR, the isotope is left in the applicator for several hours to days or may be inserted directly into the target organ and left there permanently. Radiation causes its effects in tissue by producing ionizations within the cell. The target in cells is the DNA molecule, and the ionizations will produce breaks in the DNA strand. Nonmalignant, healthy cells can repair this damage if it is confined to a single strand because the opposite strand serves as a template for repair. However, if two strand breaks occur on opposite chains in close proximity, the two ends of the chromosome may drift apart. These fragmented chromosomes may adhere to other chromosomes or they may sort out randomly when the cell divides, resulting in the daughter cells to be missing critical segments of DNA or have excess and unregulated copies of other genes, either of which may be fatal to the cell. Thus the damaged cells are observed to die a mitotic death; the lethal effect of radiation is not expressed until the cell replicates. Radiobiologic research is ongoing to identify factors that affect the survival of cells and methods to selectively increase radiation effects on tumor cells. The easiest method to assay the effect of radiation is to deliver a dose of radiation to a suspension of single cells and then measure the percentage of surviving cells. The technique uses a suspension with a known concentration of cells. A sample is placed on a culture plate and the colonies are counted. At the same time a similar-sized sample is radiated and plated and the number of colonies counted. The ratio of the number of colonies developing on the two plates is the surviving fraction. Because a Gy represents a specific amount of energy transferred to tissue, each Gy in theory should have an equal physical effect on the cell. However, the biologic effect is less for the first Gy than it is for the 101st Gy, suggesting that cells are capable of accumulating a certain amount of damage that is not lethal. Interestingly, if the radiation dose is split up and delivered over several fractions with time between fractions for cells to repair the damage, each fraction of radiation will kill the same proportion of the surviving cells. If the cells are allowed to completely repair the damage from the first dose, the second dose of radiation will have the same effect on the surviving cells as it would have if they had never been radiated the first time. This effect demonstrates the importance of uninterrupted radiation treatments. In vitro studies have shown normal cells to repair radiation-induced damage more efficiently than tumor cells, and attempts to deliver two doses of radiation per day, known as hyperfractionation, may increase the efficacy of treatment. Oxygen is known to enhance the effects of radiation both in vivo and in vitro. Cells are more sensitive when radiated under oxygenated conditions than when they are hypoxic. Normal tissues are always oxygenated, but experimental measurements in tumors have shown that these cells pile up around a capillary and consume the available oxygen, limiting diffusion into the deeper tissues. This scenario results in well-oxygenated, relatively sensitive cells adjacent to the capillary and necrotic material at a distance away from the capillary. Between these two areas is a region of viable but hypoxic cells that are more resistant to radiation. In vitro studies show that hypoxic cells require 1.5 to three times as much radiation to achieve the same cell kill. Current efforts are being taken to develop substances that act like oxygen in cells but are not metabolized like oxygen. Ideally, these substances will help to deal with the problem of hypoxic tumor cells without affecting the sensitivity of normal cells. In vitro work showed that these compounds, known as radiosensitizers, sensitized hypoxic tumor cells to the effects of radiation. However, clinical trials were limited by toxicity. Similarly, efforts to develop drugs that protect normal cells from the effects of radiation are underway. Although these trials also have failed to show in vivo benefit, efforts continue to develop a new generation of sensitizers and protectors. More importantly, chemotherapy and hormonal therapies have been observed to affect tumor sensitivity in vivo, and these agents form the basis of many current combined modality trials. The delivery of radiation to a tumor requires a physician to perform five functions: (1) identify and locate the structures to be treated, (2) identify those adjacent structures that need to be protected, (3) prescribe the appropriate dose of radiation for the tumor based on its size and histology, (4) consider the tolerance of tissue within the target that may be affected by treatment, and (5) consider the tolerance of tissues between the skin and the target (transit volume). Although a complex but solvable problem, most clinical situations require some compromise on one or more issues. The clinician must select those compromises that will minimize effects on normal tissue while maximizing the probability of tumor control, known as the therapeutic ratio. Modern equipment and computer software allow for sophisticated three-dimensional conformal radiation treatment (3D-CRT) and intensity modulated radiation therapy (IMRT). Both of these techniques have become the standard of care in most radiation oncology centers. Patients undergo computed tomography (CT)-based simulation in which a series of axial images are obtained of the target and the adjacent critical structures. Often fiducial markers are placed prior to simulation in the target organ to improve target localization during the treatments. The target or targets, depending on other areas at risk for tumor involvement, bony structures, and sensitive normal tissues, are defined on the CT imaging. Today’s treatment machines are able to deliver radiation from a wide range of angles to specifically shape dose intensities to conform around the target. For example, in the treatment of prostate cancer, newer techniques using IMRT are able to reduce the dose exposure to normal tissues and allow for higher doses to be delivered to the prostate without increased complications. Computers are used to plan the delivery of radiation using several smaller radiation fields, known as beamlets, from different beam directions. Each beamlet treats only a portion of the prostate, but when the dose intensity from all of the beamlets are added together, the entire prostate receives the full dose with slight inhomogeneity, while reducing the volume of normal tissue receiving a high dose. Although the part or the entire target receives the maximal dose, a larger volume of normal tissue is exposed to a small amount of radiation. Figure 18-e1 A-D shows the dose distribution around the target and the nearby organs. Because of the decreased exposure to normal tissues, higher and more effective doses can be delivered to the target with patients experiencing fewer side effects. As with conventional radiation delivery techniques, patient positioning is extremely critical in the delivery of IMRT; these techniques require consistent localization and patient positioning to allow tighter margins and potentially deliver higher doses. Immobilization casts or molds, rectal balloons, and fiducial markers are all tools used to assist in proper targeting of the radiation to the desired location. An alternative method is to deliver the radiation from within the tumor using interstitial radiation, or brachytherapy. LDR permanent implants in prostate cancer typically use radioactive seeds containing iodine or palladium isotopes. The patient is taken to the operating room, where epidural, spinal or general anesthesia is administered and the patient is placed in the lithotomy position. Using transrectal ultrasound guidance, hollow needles are placed through the perineum into the prostate, and the radioactive seeds are deposited interstitially. The needles are removed after the procedure. Seed positions within the prostate are determined prior to the procedure using reconstructed images from sophisticated CT, MRI, and/or ultrasound planning equipment. Intraoperative planning can also be accomplished with the assistance of a physicist in the operating room, allowing for real-time modification of the treatment plan at the time of the procedure to ensure accurate placement of seeds. After the procedure, reimaging confirms seed placement, and the quality of the implant can be judged. Figure 18-e2A shows axial slices through the prostate demonstrating the placement of seeds in the prostate. Figure 18-e2B shows a 3-D reconstruction of the prostate obtained from the postimplant CT scan demonstrating radioactive dose clouds from the seeds within the prostate. Although the implant delivers lower doses to the periprostatic tissues, substantially higher doses are delivered to the interior of the prostate. As with LDR brachytherapy, in HDR brachytherapy the patient is sedated and anesthetized, after which hollow catheters are inserted percutaneously through the perineum into the prostate. A radiation plan is designed based on the 3-D rendering of the prostate, rectum, urethra, bladder, and catheters through which the radioactive sources will travel, with a goal of emitting adequate dose throughout the prostate gland from the designated positions. The radiation oncologist can preferentially deliver a higher dose to certain parts of the organ, while sparing critical areas such as tissue surrounding the urethra. Images of HDR brachytherapy of the prostate are shown in Figure 18-e3 A-C. Because many prostate tumors have some degree of extracapsular extension (ECE), the reduced periprostatic dose may not effectively treat the tumor volume. Patients with very large prostate glands may have anatomical constraints because of the pelvic bones (i.e., pubic arch interference) that could limit placement of the needles or applicators. However, with appropriate intraoperative positioning techniques, many larger prostates can be implanted. With HDR brachytherapy, radiation doses can be delivered beyond 3 mm, which is the distance from the capsule that 90% of microscopic ECE extends. Furthermore, Merrick et al demonstrated that prostates greater than 60 cc had no greater risk of postimplant obstruction. The role of radiation for renal cancers is currently evolving with ongoing studies looking at stereotactic radiosurgery for unresectable tumors. Furthermore, the use of radiation in the treatment of patients following resection remains controversial. Among patients with tumor transgressing the renal capsule, the risk of local recurrence is high. Rafla et al reviewed his experience and found an apparent benefit to the use of adjuvant radiation therapy for patients with involvement of the renal capsule (T3). Among patients receiving adjuvant radiation, the 5-year survival was 57% compared to 28% for patients treated with surgery alone. It is presumed that the improved survival was related to the reduced incidence of local recurrence, although this finding could not be documented because these patients were treated in the pre-CT era. In contrast, subsequent trials reported by Finney et al and Kjaer et al found no benefit with adjuvant radiation. The results of the Finney trial although limited by the small number of patients, demonstrated an 8% treatment-related mortality as a result of liver injury in the radiation arm. The results reported by Kjaer are clouded by the registration of node-positive patients in whom Rafla demonstrated no benefit. This study also was complicated by the fact that 16% of the patients randomized to radiation did not receive radiation and those patients who were treated experienced a 44% incidence of major toxicity. Both Finney and Kjaer used anterior and posterior fields, and the toxicity occurred in patients with right-sided tumors. In such cases, large parts of the liver were treated to 45 Gy, which is well above liver tolerance. Because many tumors today are found incidentally at the time of a diagnostic CT scan for other reasons, it is not clear that these patients have the same prognosis after surgery as patients in these older studies who presented with hematuria, mass, or other more advanced findings. Given the potential serious side effects and little data demonstrating a benefit, radiation is not generally indicated in the adjuvant setting for renal cell cancers. Radiation, however, is often used for metastatic renal cell carcinoma to palliate bleeding, pain, or neurologic symptoms related to spinal or central nervous system (CNS) involvement. These tumors have traditionally been thought to be radioresistant, arguing for surgical excision of limited metastatic disease. In a review of their experiences, Onufrey and Halpern reported that although these tumors tended to respond slowly and did not seem to respond to higher doses of radiation, patients still achieved good palliation of symptoms in most cases. Zelefsky et al and Yamada et al have demonstrated that renal cell carcinomas can respond to higher hypofractionated radiation doses in the range of those typically used with stereotactic body radiation therapy.
Radiation Therapy
Physics
Radiobiology
Treatment planning
Clinical radiation oncology
Renal pelvic and ureteral carcinoma
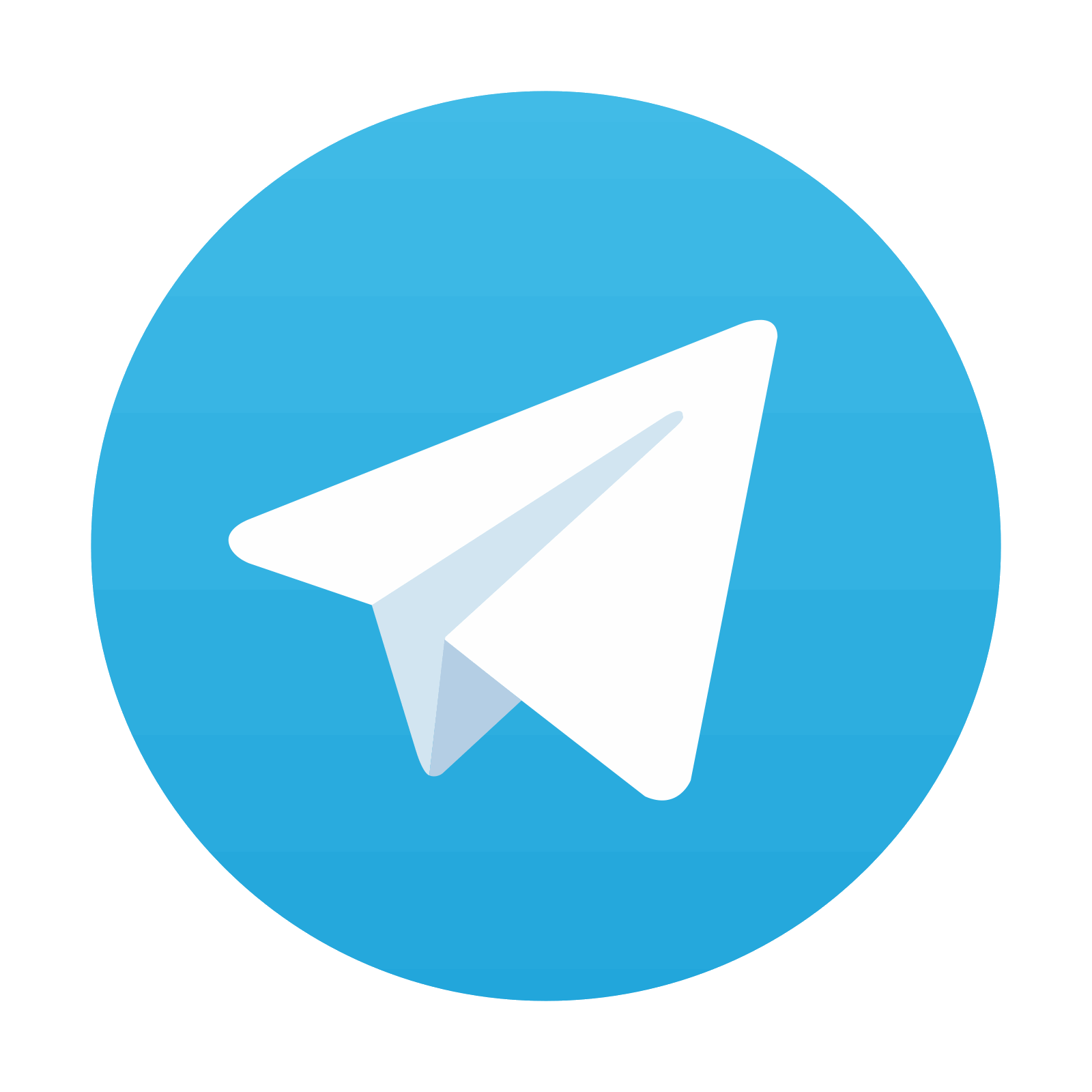
Stay updated, free articles. Join our Telegram channel
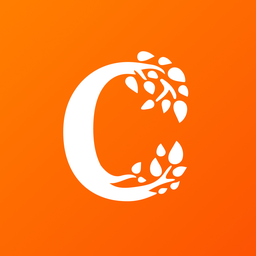
Full access? Get Clinical Tree
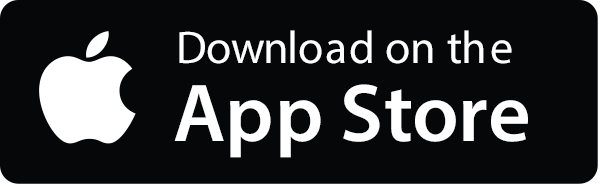
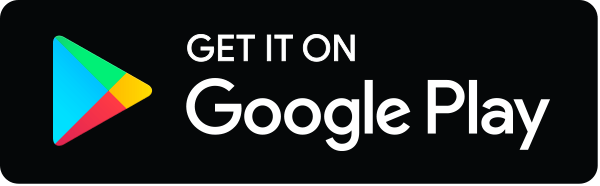