The successful transition from single cells to complex multicellular organisms has required the development of mechanisms for cells to communicate with each other, so as to act in concert during processes such as nutrient acquisition, motility, and defense. The most fundamental of these are cell–cell junctions that serve as structural organizers, but also provide information that individual cells can utilize to orient themselves in relation to the remainder of the organism. In larger species that contain multiple organs and cell types, the need to communicate information over long distances has led to the development of diffusible factors that are secreted by one cell and travel to distant cells. These factors can be delivered locally, via the circulation (e.g., hormones and cytokines) or via the nervous system (e.g., neurotransmitters), and are recognized by the appropriate cell surface receptor on the recipient cell. The complex nature of the numerous signals presented to the cell at any given point in time has led to the development of an intricate array of receptor-activated intracellular second messengers that, by undergoing a coordinated series of interactions and enzymatic alterations, can transduce the information presented on the cell surface to effector molecules that mediate the appropriate cellular response.
Introduction
The successful transition from single cells to complex multicellular organisms has required the development of mechanisms for cells to communicate with each other, so as to act in concert during processes such as nutrient acquisition, motility, and defense. The most fundamental of these are cell–cell junctions that serve as structural organizers, but also provide information that individual cells can utilize to orient themselves in relation to the remainder of the organism. In larger species that contain multiple organs and cell types, the need to communicate information over long distances has led to the development of diffusible factors that are secreted by one cell and travel to distant cells. These factors can be delivered locally, via the circulation (e.g., hormones and cytokines) or via the nervous system (e.g., neurotransmitters), and are recognized by the appropriate cell surface receptor on the recipient cell. The complex nature of the numerous signals presented to the cell at any given point in time has led to the development of an intricate array of receptor-activated intracellular second messengers that, by undergoing a coordinated series of interactions and enzymatic alterations, can transduce the information presented on the cell surface to effector molecules that mediate the appropriate cellular response.
The kidney serves to protect the internal milieu of higher organisms from perturbations due to the accumulation of metabolic products, as well as those resulting from fluctuations in the intake or loss of water and various salts. To regulate this intricate function, the body must continuously monitor the composition and quantity of the extracellular fluid, and then signal the nephron to appropriately regulate glomerular filtration and tubular cell function in response to changes in these parameters. Regulation of these exquisitely precise events requires that the cells of the kidney are able to respond to signals emanating from distant sites, and then efficiently communicate in an intercellular and intracellular manner to coordinate the response. This chapter will provide an overview of several of the most common receptors and intracellular second messenger pathways that are utilized in this process.
Cell Surface Receptors
In the best studied pathway of cell signaling, a first messenger is secreted by one group of cells and travels either to distant cells (endocrine factors) or to local cells (autocrine or paracrine factors), where it binds to a specific receptor. The first messengers in these classic pathways are generally either proteins (growth factors, cytokines), catecholamines (epinephrine, dopamine) or steroids (mineralocorticoids, sex hormones), although receptors have been identified for multiple circulating factors including lipids (e.g., lysophosphatidic acid), ions (e.g., calcium), eicosanoids (e.g., prostaglandin E 2 ), sugars (e.g., glucose), nucleosides (e.g., ATP), and gases (e.g., nitric oxide). Most of these receptors are located on the cell surface and have an extracellular region (domain) that recognizes and binds to the specific ligand. This ligand-binding domain is connected via one or more transmembrane segments to the intracellular (cytosolic) domain that undergoes a change in conformation or activity in response to ligand binding, and thus initiates the activation and/or modification of intracellular second messengers. In contrast to these cell surface receptors, steroid receptors, which are discussed later in this chapter, are typically located in the cytoplasm. The lipophilic steroid ligands are capable of crossing the cell membrane and binding the receptor, which then initiates signaling events by translocating into the nucleus where the ligand–receptor complex can regulate gene transcription.
Based on their structure, the type of ligand that they bind, and the principle second messengers that are activated, classical cell surface receptors can be grouped into G-protein coupled receptors, receptor tyrosine kinases (RTKs), serine/threonine kinase receptors, and receptor-like phosphatases. However, it has become increasingly clear that other surface proteins serve as signaling initiators to transduce information about the environment surrounding the cell. Thus, cell–cell and cell–matrix adhesion molecules initiate signaling cascades that regulate cell shape, differentiation, proliferation, and survival. The following section will provide a brief overview of these various signaling initiators, focused on those presently considered to be important in regulating normal renal development and maintaining adult kidney homeostasis.
G-Protein Coupled Receptors
The receptor sub-type that is responsible for mediating the signaling responses of the greatest number of ligands in the kidney is probably the G-protein-coupled receptor (GPCR). GPCRs make up the largest family of cell surface receptors, with over 800 members predicted from the sequence of the human genome (reviewed in ) ( Figure 13.1 ). GPCRs are transmembrane proteins with their amino terminus on the cell exterior, seven transmembrane α helical segments, and the carboxyl terminus in the cell interior. This arrangement results in three extracellular loops and three intracellular loops joining the transmembrane segments. They bind to extracellular ligands such as epinephrine, dopamine, angiotensin II, adenosine, vasopressin, calcium, and parathyroid hormone, and mediate their intracellular actions.

The extracellular loops serve as the primary binding site for the specific GPCR ligand, with the amino terminus also contributing to the binding site for some ligands. The intracellular loops, most critically the 5–6 loop, serve as the binding site for the principal GPCR intracellular effectors, the heterotrimeric G-proteins. These small GDP/GTP-binding protein complexes are made up of α-, β-, and γ-subunits, with the α-subunit serving as the GDP/GTP-binding site, and the βγ-subunits acting both as regulators of α-subunit localization, and independently as intracellular signaling effectors. The existence of multiple different α-, β-, and γ-subunits allows for hundreds of potential combinations of heterotrimeric G-proteins, and thus imparts specificity of response to the individual GPCR and its ligand.
In the absence of receptor activation, the α-subunit is bound to GDP, and associates with the βγ-subunits at the membrane. However, following ligand binding to the extracellular surface of the GPCR, a conformational change of the receptor results in disassociation of GDP, and binding of GTP to the α-subunit. The binding of GTP stimulates disassociation of the α-subunit from the βγ-subunits and the receptor. The GTP-loaded α-subunit can then associate with its intermediary effectors (such as adenylyl cyclase and phospholipase), while the βγ-subunits can associate with and regulate independent effectors, such as ion channels and the β a drenergic r eceptor k inase (βARK) (reviewed in ).
The protein products of the 16 mammalian genes encoding Gα-subunits have been grouped into four classes, the G sα (stimulatory for adenylyl cyclase), G iα (inhibitory for adenylyl cyclase), G q/11α (regulators of phospholipase Cβ (PLCβ)), and G 12α (regulators of RhoGEF). Binding of the appropriate GTP-loaded Gα-subunit to its primary effector results in the activation or inhibition of effector function; for example, adenylyl cyclase catalyzes the cyclation of ATP to form 3′,5′-cyclic AMP (cAMP), an intracellular second messenger that can bind and activate downstream signaling proteins such as p rotein k inase A (PKA). This reaction is activated by GTP-G sα binding to adenylyl cyclase and inhibited by GTP-G iα binding. In addition, βγ binding to adenylyl cyclase can augment its activation by GTP-G sα .
An important concept in all forms of signal transduction is the ability of the cell to carefully control the location, amplitude, and duration of the signal. Signal amplification is the process whereby the cell can regulate the amplitude of the signal. For example, a single GPCR can generate between tens and hundreds of GTP-coupled Gα-subunits, which can subsequently bind to and activate similar numbers of adenylyl cyclase enzymes, which in turn generate multiple copies of cAMP. The number and availability of the intracellular effector enzymes and substrates thus determines the level of signal amplification following the activation of relatively few receptors on the cell surface.
Just as important as signal amplification is the ability of the cell to downregulate the signaling pathway once the desired response has been initiated. For GPCRs, this occurs in several ways. First, the α-subunit is itself a GTPase, meaning that it hydrolyzes GTP to form GDP and inorganic phosphate. This hydrolysis occurs spontaneously following GTP binding to Gα, but can be augmented by the association of specific RGS proteins ( r egulators of G -protein s ignaling) with the GDP/Gα complex, as this interaction stabilizes the inactivated state. Once the Gα-subunit is in the GDP-bound state, it can associate again with the βγ-subunit to regenerate the inactive heterotrimeric G-protein.
In addition, many GPCRs are themselves inactivated by a process called homologous desensitization. As has been noted above, the βγ-subunits can associate with the cytosolic protein βARK. βARK, also known as GRK2, is a member of the G -protein-coupled r eceptor k inases (GRKs) that, following association with G βγ , phosphorylate the intracellular loops and/or C terminus of ligand-associated GPCRs on serine and/or threonine residues. This phosphorylation results in the association of β-arrestin with the receptor, mediating the uncoupling of the ligand–receptor complex from the heterotrimeric G-proteins, and thus diminishing its activity. Binding of β-arrestin has also been shown to target the receptor–ligand complexes to clathrin-coated pits on the cell surface, followed by internalization and either lysosomal degradation or recycling of the inactivated receptor to the cell surface.
The downstream GPCR effector adenylyl cyclase is also subject to phosphorylation-dependent inhibition. As noted above, activated adenylyl cyclase catalyzes the formation of cAMP, which in turn associates with and activates PKA. This enzyme, an intracellular serine/threonine kinase, has multiple phosphorylation substrates within the cell. Phosphorylation of these substrates can regulate their activity, cellular localization, and/or their interaction with other proteins. One phosphorylation substrate is adenylyl cyclase itself, resulting in inhibition of cAMP production. A second substrate is the GPCR. In a process known as heterologous desensitization, activation of PKA by a non-GPCR signal can result in phosphorylation of the GPCR and subsequent inhibition of ligand-mediated GPCR activation.
Receptors for Dopamine and AVP are GPCRs that Regulate Adenylyl Cyclase
A prototypic family of GPCRs in the kidney is the dopamine receptors. There are five dopamine receptors presently described (D 1 –D 5 ), and they are further sub-classified into D 1 -like (D 1 and D 5 ) or D 2 -like (D 2 –D 4 ). The D 1 -like receptors are associated with G sα , and therefore activate adenylyl cyclase, whereas the D 2 -like receptors inhibit adenylyl cyclase activity (reviewed in ). In the kidney, D 1 -like and D 2 -like receptors are expressed throughout the tubules. The net effect of activating these receptors is the induction of a salt and water diuresis, although by different mechanisms in the different tubular segments. Dopamine-mediated activation of D 1 receptors in the proximal tubule results in activation of adenylyl cyclase, leading to cAMP-dependent inhibition of the activity of NHE-3, NaPi-2, and the Na,K-ATPase, thus inhibiting proximal sodium reabsorption. In contrast, activation of the D 2 -like receptor D 4 in the cortical collecting duct leads to a water diuresis by preventing vasopressin-stimulated adenylyl cyclase activation. Dopamine receptors also mediate renal vasodilation, and appear to regulate renin secretion. Due to these effects, defects in dopamine receptor function in mice are associated with salt retention, vasoconstriction, and increased blood pressure.
Arginine vasopressin (AVP, also known as anti-diuretic hormone (ADH)) binds to three GPCRs, the G q/11α -linked V1a and V1b receptors, and the G sα -linked V2 receptor. V1 receptors are located on several cell types, including smooth muscle cells of blood vessels, where they mediate the vasoconstrictive (“pressor”) response of vasopressin, while V2 receptors are located on epithelial cells in the collecting duct and mediate water reabsorption. Binding of vasopressin to the V2 receptor stimulates adenylyl cyclase-mediated cAMP production, which in turn causes insertion of vesicles containing the water channel aquaporin-2 into the apical membrane of collecting duct cells. By inhibiting adenylyl cyclase activation in these cells, dopamine can partially counteract this water-reabsorptive effect of vasopressin.
GPCRs Can also Signal through Phospholipase C and MAPK
An example of a GPCR that is coupled to PLCβ signaling is the type 1 receptor for angiotensin II (AT 1A R). Angiotensin II is the eight amino acid peptide product of the angiotensin converting enzyme (ACE)-mediated cleavage of angiotensin I. Angiotensin II is capable of binding to and activating two distinct G-protein coupled receptors, the type 1 receptor (AT 1 R) and the type 2 receptor (AT 2 R). The predominant actions of angiotensin in the kidney and adrenal gland are mediated by the AT 1 R, including vasoconstriction, smooth muscle hypertrophy, sodium retention, and aldosterone secretion. Most data presently support the idea that the AT 2 R receptor acts as an antagonist to AT 1 R signaling, although exactly how the AT 2 R signals has been much less apparent. The topology of the AT 2 R is consistent with a seven transmembrane G-protein coupled receptor, yet it has been controversial as to whether AT 2 R in fact signals via traditional G-proteins. There have been several reports that AT 2 R can signal via G iα , although this has not been universally accepted (reviewed in ). Other groups have suggested that AT 2 R signaling is pertussis-toxin insensitive (i.e., not dependent on G iα ), and is instead mediated by production of cyclic GMP. It remains unclear whether the generation of cGMP is a direct result of AT 2 R activation or is mediated in an autocrine/paracrine fashion by AT 2 R-stimulated bradykinin production.
The AT 1 R is in the G q/11α family of GPCRs, meaning that binding of angiotensin II to the receptor stimulates GTP-loading of G qα which in turn associates with and activates phospholipase Cβ (PLCβ) ( Figure 13.2 ). The active form of PLCβ mediates the hydrolysis of phosphotidylinositol 4,5 bisphosphate (PI 4,5 P 2 ) in the membrane to produce diacylglycerol (DAG) and inositol trisphosphate (IP 3 ). IP 3 is hydrophilic and enters the cytoplasm where it activates the IP 3 receptor on the surface of the endoplasmic reticulum, thereby stimulating calcium release from internal stores. The simultaneous production of DAG at the membrane, and local release of stored calcium, leads to the recruitment and activation of the classic, calcium-dependent protein kinases C (PKCs). Activation of PKC appears to be required for angiotensin II-mediated renal efferent arteriole vasoconstriction, Na,K-ATPase recruitment to the membrane in proximal tubule cells (resulting in increased proximal sodium reabsorption), and stimulation of aldosterone secretion by adrenal zona glomerulosa cells.

In addition to activation of PLCβ, a second signaling pathway that is activated by the AT 1 R is the m itogen a ctivated p rotein k inase (MAPK) pathway. As mentioned earlier, phosphorylation of GPCRs by βARK results in the recruitment of β-arrestin to the receptor complex, and subsequent receptor internalization. β-arrestin has been found to act as a binding scaffold for the core components of the MAPK pathway, including Raf, MEK, and ERK (see “Intracellular Signaling Pathways,” below; reviewed in ), resulting in the activation of this pathway that mediates cell growth and proliferation. This scaffolding function of β-arrestin appears to be both cell type and receptor specific, and can mediate activation of additional intracellular signaling pathways, including the phosphoinositide 3-kinase (PI 3-K) pathway that will be discussed later in this chapter. Activation of these signaling pathways appears to play an important role during kidney development, since newborn mice null for the type I angiotensin receptor or in which angiotensin signaling has been inhibited, have significant renal developmental abnormalities, including renal arterial hypertrophy and papillary atrophy.
A second means by which GPCRs can activate MAPK signaling was discovered when the levels of β-arrestin were depleted using RNA interference (RNAi). Under these conditions, angiotensin II was still able to activate MAPK, although to a lesser degree. These experiments uncovered a β-arrestin-independent pathway of angiotensin II-dependent MAPK stimulation that occurs via activation of a second cell surface receptor, a process known as receptor transactivation. As noted above, stimulation of G qα by the AT 1A R leads to activation of PKC. PKC, in addition to regulating processes such as ion transport, can activate a cell surface protein called heparin-binding epidermal growth factor (HB-EGF). HB-EGF is one of the ligands for a separate cell surface receptor, the epidermal growth factor receptor (EGFR), and binding of HB-EGF to the EGFR results in the stimulation of multiple signaling events, including MAPK activation (see “Intracellular Signaling Pathways”).
Localization and Timing of Pathway Activation Promotes Diverse Cell type Specific Responses
These two independent pathways for activating MAPK signaling provide an example of how scaffolding proteins can compartmentalize signaling within the cell. The β-arrestin-mediated ERK activation is sustained for several hours and occurs in the cytoplasm, whereas the G qα /PKC-dependent ERK activation appears to be more transient and primarily within the nucleus. This ability to localize activated ERK in different cellular compartments allows the cell to differentially regulate specific effector proteins, and thus direct distinct cellular outcomes. In the heart, for example, AT 1 R-mediated G qα /PKC-dependent transactivation of the EGFR, and ultimately MAPK nuclear signaling, is believed to at least partially mediate angiotensin-stimulated cardiac hypertrophy.
Many GPCRs can activate multiple Gα-subunits, depending on cellular location and availability. For example, the parathyroid hormone (PTH) receptor can potentially activate G sα (thus activating adenylate cyclase and PKA), G qα (activating PLCβ and PKC), and G i (inhibiting adenylate cyclase). PTH is an 84 amino acid peptide hormone secreted by the parathyroid gland that acts on bone to increase calcium and phosphate release into the circulation, as well as on the proximal and distal tubules of the kidney to inhibit phosphate reabsorption and stimulate calcium reabsorption, respectively. PTH is proteolytically processed to generate multiple fragments which can bind to and activate the PTH receptor, a class B GPCR (defined by the six conserved cysteine residues that form disulfide bonds in the large extracellular amino terminal domain). Expression of a mutant form of the receptor that selectively fails to activate G qα -dependent PLCβ signaling in mice results in abnormalities in bone ossification without a change in serum calcium. The normal serum calcium in these animals suggests that renal tubular calcium handling in these mice is dependent on G sα – or G i -regulated adenylate cyclase signaling, while bone ossification appears to require G qα -PLCβ signaling. In support of this hypothesis, complete loss of PTH receptor signaling results in hypocalcemia in addition to bone abnormalities. In humans, this is recapitulated by an autosomal recessive mutation in the receptor in patients with Blomstrand chondrodysplasia, a lethal disorder characterized by excessive bone maturation and mineralization.
As noted previously, receptor internalization mediated by β-arrestin is frequently a means by which GPCR are uncoupled from their ligands and signaling is downregulated. For example, PTH related protein (PTHrP) can bind and activate the PTH receptor, leading to GTP-loading of G sα and a transient increase in cAMP followed by receptor desensitization. In contrast, PTH receptor ligands such as PTH 1–34 (the first 34 amino acids of PTH) can induce a more sustained increase in adenylyl cyclase activation and cAMP levels, leading to systemic responses such as increased vitamin D hydroxylation and higher serum calcium levels. Investigation into the mechanism of this difference has demonstrated that binding of PTH 1–34 to the PTH receptor leads to internalization of the active receptor–ligand complex in endosomes that also contain adenylyl cyclase, leading to sustained signaling from this intracellular site. These structures, referred to as signaling endosomes, have been shown to mediate signaling via multiple receptor types in addition to GPCR, and to regulate complex cellular responses such as migration, differentiation, and asymmetric division.
Kinase Receptors
A second class of transmembrane receptors is the kinase receptors. These proteins typically contain an extracellular ligand-binding domain at the amino terminus, a single membrane-spanning domain, and an intracellular carboxy terminus that includes the kinase domain. In most cases, binding of the ligand to the receptor results in homodimerization of two receptor molecules, bringing the intracellular kinase domains into close proximity where they phosphorylate substrate residues on the adjacent receptor. This phosphorylation step generates binding sites for the recruitment of intracellular signaling molecules, as well as further activating the kinase domain so that non-receptor substrates recruited to the complex can also be phosphorylated.
Tyrosine Kinase Receptors
The largest class of kinase receptors is the tyrosine kinase receptors, also known as receptor tyrosine kinases (RTKs) ( Figure 13.3 ). These molecules frequently serve as receptors for extracellular growth factors, circulating proteins that stimulate cell growth and division. Examples of ligand–receptor combinations in this family include epidermal growth factor (EGF) and its receptors ErbB1 (or EGFR) and ErbB2; platelet-derived growth factor (PDGF) and the PDGF receptor; insulin and the insulin receptor; and vascular endothelial growth factor and its major receptors VEGFR1 and VEGFR2 (also called Flt1 and Flk1).

Once the growth factor has bound to and activated the receptor, newly phosphorylated tyrosine residues on the intracellular carboxy terminus of the receptor serve as binding sites for cytosolic or membrane-associated proteins that contain phosphotyrosine-binding domains. The best characterized of these domains are the s rc- h omology 2 (SH2) domains that share characteristic features first described in the phosphotyrosine-binding region of the cytosolic tyrosine kinase Src. SH2 domains are approximately 100 amino acids in length, and provide specificity of interaction in two ways. First, the interaction of the binding pocket of the SH2 domain and the tyrosine residue is only stabilized when the tyrosine residue is phosphorylated. Second, the amino acids immediately flanking the phosphorylated tyrosine residue determine which SH2 domain interaction is preferred. For example, the SH2 domain on the p85 adaptor protein, α-subunit of the lipid enzyme phosphoinositide 3-kinase (PI 3-K, see “Intracellular Signaling Pathways,” below), strongly prefers to bind to phosphotyrosine with a methionine residue at the +3 position. Thus, receptors containing the sequence pTyr-X-X-Met (where X can be almost any amino acid) specifically recruit and activate the PI 3-K.
In the kidney, tyrosine kinase receptors have been implicated in controlling development, mediating hypertrophy, regulating the balance between repair and fibrosis after injury, and promoting the growth of renal carcinomas. During development, glial derived neurotrophic factor (Gdnf) is made by the embryonic metanephric mesenchyme and activates the c-Ret tyrosine kinase receptor that is expressed on the epithelial cells of the adjacent Wolffian duct. The activation of Ret is somewhat unusual, since Gdnf does not directly bind to Ret, but rather binds to a third membrane protein, Gfrα, that mediates dimerization of Ret in response to association with Gdnf (reviewed in ). Activation of Ret in this manner results in the activation of multiple intracellular signaling pathways, including the Erk-MAPK pathway, the PI 3-K pathway, members of the Src family of non-receptor tyrosine kinases, and phospholipase Cγ (PLCγ). Activation of the MAPK and Src pathways (see “Intracellular Signaling Pathways”) have been found to be critical for the outgrowth and branching of the ureteric bud from the Wolffian duct, the first step in the formation of the metanephric kidney.
Signaling by several other tyrosine kinase receptors has been implicated in kidney development, including the fibroblast growth factor (FGF) receptors, hepatocyte growth factor receptor (Met), and the epidermal growth factor receptor. FGF signaling is a complex process that includes 18 known ligands and 4 distinct tyrosine kinase receptors (FGFR1-4) (reviewed in ). Like many receptor ligands, FGFs are secreted glycoproteins that are concentrated in proximity to their cell surface receptor by binding to heparan sulfate proteoglycans on the cell and/or nearby matrix components. The interaction between FGFs and their receptors can be further regulated by cell- or tissue-specific expression of FGF co-receptors such as Klotho. Intracellular signaling by the FGFR is regulated in part by the cytosolic adaptor protein FGFR substrate 2 (FRS2), which is phosphorylated by the FGFR kinase domain, leading to the recruitment and activation of downstream MAPK and PI 3-K signaling. In the developing kidney, FGF7 and FGF10, signaling via the IIIb isoform of FGFR2, have been shown to be critical for normal branching and extension of the collecting system, while FGF8 appears to be required for nephrogenesis by the adjacent metanephric mesenchyme.
Many growth factor receptors are expressed in the mature kidney, and are believed to be critical for maintenance of normal tubule architecture and for regulating the cellular response to injury. Renal tubular epithelial cells express EGF receptors as well as Met, the receptor for hepatocyte growth factor (HGF). These tyrosine kinase receptors directly bind their respective ligands via their extracellular amino terminal domains, followed by homodimerization and activation of intracellular signaling. A major mediator of the intracellular signaling mediated by EGF and HGF is the Gab1 docking protein, which functions in a manner similar to that of FRS2 for the FGFR. Recruitment of Gab1 to Met or the EGFR results in its phosphorylation on multiple tyrosine residues and subsequent association with p85, PLCγ, Grb2, a second adaptor protein known as Crk, and the protein tyrosine phosphatase SHP2. Following acute kidney injury, the level of HGF increases in the kidney, resulting in activation of Met, mediating MAPK, PI 3-K, and PLC signaling. These pathways in turn are believed to be important for inhibition of apoptosis (the PI 3-K pathway), and stimulation of cell migration and proliferation during the repair process (PI 3-K, MAPK, and PLC pathways).
Serine-Threonine Kinase Receptors
A second group of transmembrane kinase receptors are the serine-threonine kinase receptors. Like the non-receptor kinases PKA and PKC, these receptors catalyze the phosphorylation of serine or threonine residues in their substrate molecules. Perhaps the best studied of these receptors in the kidney is the receptor for transforming growth factor β (TGFβ), a member of the TGFβ superfamily of secreted factors that also includes the bone morphogenic proteins (BMPs) and activin. TGFβ-like proteins signal into the cell via a heterotetrameric complex comprised of two subclasses of serine-threonine kinase receptors, the type I receptor and the type II receptor. Like the tyrosine kinase receptors, these proteins have an extracellular ligand recognition domain, a single transmembrane spanning domain, and an intracellular kinase domain. The different TGFβ-like ligands utilize distinct type I and II receptor combinations. For example, TGFβ1-3 signals via the combination of the type II receptor TβR-II and the type I receptors activin receptor-like kinase 1 (ALK-1) or ALK-5, while BMPs signal through the type II receptors ActR-II or BMPR-II and the type I receptors ALK-2, ALK-3 or ALK-6.
TGFβ receptor signaling begins when the ligand binds to the extracellular domain of its cognate type II receptor ( Figure 13.4 ). The kinase domain of type II receptors is constitutively active, and binding to the extracellular ligand results in the recruitment of the appropriate type I receptor to the complex, where it is phosphorylated and activated by the type II receptor. In this manner, the TGFβ1-dependent association of ALK-5 with TβR-II allows the constitutively active TβR-II to phosphorylate ALK-5 and activate its intracellular serine-threonine kinase domain. The specificity of signaling by TGFβ family members is further regulated by the presence in many cells of the accessory receptors betaglycan and endoglin. These transmembrane proteins lack intracellular kinase domains, and appear to regulate the affinity of TGFβ proteins for the various type II receptors, as well as modifying intracellular signaling by the ligand–receptor complex.

As opposed to tyrosine kinase receptors that signal primarily via recruitment of SH2 domain containing proteins to activate pathways such as MAPK and PI 3-K, TGFβ receptors signal primarily via a distinct signaling pathway, the Smad proteins. Smads are small cytoplasmic proteins that contain a DNA-binding domain and a TβR-I/Smad4-interacting domain. Based on their structure and function, Smads have been divided into three groups, the receptor activated Smads (Smad1, 2, 3, 5, 8), a regulatory Smad (Smad4), and the inhibitory Smads (Smad6, 7). Upon activation of TβR-I, the appropriate receptor activated Smads (e.g., Smad2 and 3 for ALK-5) are phosphorylated on regulatory serine residues in the TβR-I/Smad4 interacting domain, resulting in their disassociation from the receptor and association with Smad4. This Smad2–Smad4 complex then translocates to the nucleus, where the Smad DNA-binding domain can mediate direct association with S mad- b inding e lements (SBE) in the DNA of the promotor region of target genes, as well as association with other transcriptional regulators.
One of the transcriptional targets that is regulated by Smad2–4 signaling is another member of the Smad family, Smad7. Smad7 is an inhibitory Smad that can bind to TβR-I, and prevent Smad2 or Smad3 from associating and being activated. In this manner, TGFβ stimulation of Smad7 transcription provides a negative feedback loop that acts to prevent sustained Smad2 and Smad3 activation by the TGFβ receptor.
TGFβ Signaling in the Kidney
Studies in mice that have undergone genetic inactivation of various TGFβ family members demonstrate that Bmp2 and Bmp4 have important roles in normal kidney development (reviewed in ). In the mouse embryo, Bmp4 is expressed in the metanephric mesenchyme surrounding the Wolffian duct and adjacent to the ureteric bud (the epithelial structure that will branch to form the entire collecting system of the kidney), while Bmp2 is expressed in the condensing mesenchyme at the tips of the ureteric bud (the region that will differentiate into the glomerulus and proximal portions of the nephron through the connecting segment). The Bmp receptors Alk3 and Alk6 are expressed on the invading urteric bud itself. While complete loss of Bmp2 or Bmp4 results in embryonic lethality prior to kidney development, mice that are heterozygous for loss of Bmp4 expression exhibit multiple defects in the collecting system of the kidney, including doubling of the collecting system, hydroureter, and dysplastic kidneys, and Bmp2 heterozygotes demonstrate exaggerated uretic bud branching. Thus, it appears that Bmps normally act to inhibit ureteric bud outgrowth and branching during development.
In addition to their role in kidney development, TGFβ proteins have been shown to play a major role in regulating fibrotic responses of the adult kidney by both increasing new matrix deposition and inhibiting matrix degradation. In vitro studies have shown that TGFβ-dependent Smad3–Smad4 nuclear signaling can induce the expression of multiple collagen isoforms, along with their cellular binding partner β1 integrin, and activated Smad3 has been found to mediate decreased transcription of the gene for matrix metalloproteinase-1 ( MMP-1 ) . In support of an important role for the TGFβ-Smad signaling pathway in the development and progression of renal fibrosis in vivo , genetic overexpression of TGFβ in the rat has been shown to induce glomerulosclerosis due to increased extracellular matrix deposition, while mice lacking Smad3 demonstrate less fibrosis following ureteral obstruction.
Receptor-Like Phosphatases
Much attention has been focused in the field of signal transduction on the role of substrate phosphorylation by receptor kinases in regulating protein–protein interactions or altering the activity of effector proteins. However, a second class of proteins, the phosphatases, is emerging as equally important signaling regulators in determining cellular responses. Similar to kinases, phosphatases can be grouped into transmembrane receptor-like phosphatases and intracellular (cytosolic) phosphatases (to be discussed below). The receptor-like phosphatases that have been identified to date are protein tyrosine phosphatases (PTPs) with an extracellular domain, single transmembrane spanning segment, and intracellular phosphatase domain.
The first receptor-like PTP to be cloned and sequenced was the neutrophil antigen CD45. This protein was found to be necessary for both T-cell development and T-cell activation following engagement of the T-cell receptor (reviewed in ). Although there has been no activating ligand identified for CD45, binding to extracellular galectin-1 inhibits CD45 phosphatase activity, resulting in T-cell death. Several intracellular substrates for CD45 have been identified, including members of the Src and JAK kinase families. One critical substrate of CD45 in T-cell signaling is the cytosolic tyrosine kinase Lck ( Figure 13.5 ). Lck, like the related tyrosine kinase Src, is normally maintained in the inactive state by the association of phosphotyrosine 505 near the carboxy terminus with its own SH2 domain closer to the amino terminus of the protein. This interaction results in folding of Lck, and thereby prevents the intervening tyrosine kinase domain from recognizing or phosphorylating its substrates. Dephosphorylation of phosphotyrosine 505 by CD45 following antigen presentation by a nearby dendritic cell allows Lck to unfold, and activates the kinase domain. The resultant phosphorylation of the ζ-chain of the T-cell receptor by activated Lck is necessary for recruitment of a second cytosolic tyrosine kinase, ZAP70, to the complex, and subsequent T-cell activation. The administration of monoclonal antibodies that prevent activation of CD45 has been shown to markedly diminish the occurrence of acute rejection in a rodent model of kidney transplantation.

In contrast to CD45, the receptor-like protein tyrosine phosphatase PTPζ/β is expressed on epithelial cells, and has been found to bind to several putative extracellular ligands, including contactin, neural cell adhesion molecule (NCAM), and pleiotrophin. Rather than activating PTPζ/β, as is typical of most ligand–receptor interactions, the association of pleiotrophin with PTPζ/β inhibits the phosphatase activity of PTPζ/β. In the kidney, pleiotrophin has been shown to markedly increase branching by the explanted ureteric bud, and thus is believed to play a significant role in determining the number of nephrons that form during kidney development. One substrate for PTPζ/β is the cytosolic protein Git1, a multifunctional adaptor protein that can regulate the signaling pathways that control actin cytoskeletal rearrangement. Dephosphorylation of Git1 by PTPζ/β is therefore proposed to play an important role in the regulation of cell adhesion and migration, as well as cytosolic vesicle trafficking.
Receptors Activated by Proteolytic Cleavage
The previously described receptors bind to their respective ligands and then signal into the cell via activation of substrate protein phosphorylation or dephosphorylation, thus regulating cytosolic signaling pathways that in turn mediate the activation or inhibition of downstream effectors. In contrast, cleavage-activated receptors such as Notch signal directly to the nucleus to regulate gene transcriptional events. Notch is a cell surface protein that contains an extracellular ligand-binding domain, a single transmembrane spanning segment, and an intracellular domain capable of binding and activating nuclear transcriptional factors (reviewed in ). The classic Notch ligands, Jagged and Delta, are also transmembrane proteins that contain extracellular EGF-like repeats and a unique domain for binding Notch. When Jagged on one cell engages Notch on an adjacent cell, a cleavage site is exposed on the extracellular side of Notch near the membrane, and Notch is cleaved by a member of the A D isintegrin A nd M etalloproteinase (ADAM) family of proteases. The remaining transmembrane/intracellular portion of Notch then becomes a target for further cleavage by presenilin (a member of the γ-secretase complex) at a conserved site in the intramembranous domain of Notch. Cumulatively, this process is termed regulated intramembranous proteolysis (RIP) ( Figure 13.6 ).

This final cleavage event releases the cytosolic domain of Notch (called the Notch intracellular domain (NICD)) that translocates to the nucleus where it can directly bind and regulate transcription factors. In mammals, Notch controls transcriptional regulation by interacting with the DNA-binding protein CSL (also called RBP-J), which in turn regulates transcriptional expression of members of the H airy and E nhancer of S plit (HES), and H airy- R elated T ranscription factor (HRT) family of transcription factors. These nuclear proteins control the expression of genes that are critical for regulating normal development.
In the kidney, activation of Notch signaling has been implicated in the specification of the proximal tubule and glomerular podocytes during development. The cleaved form of Notch is present in the developing S-shaped body that ultimately differentiates into the nephron, and inhibition of γ-secretase in organ culture leads to the loss of the proximal nephron, even though the distal nephron still develops and fuses to the collecting duct. Genetic interruption of Notch expression has revealed that nuclear signaling by Notch2, rather than Notch1, is required for this proximal specification. In the mature kidney, Notch expression is normally downregulated. However, in disease states such as diabetic nephropathy, re-expression of Notch can activate the transcription of cell cycle genes, leading to increased cell proliferation and ultimately exaggerated fibrosis.
More recently, it has become clear that receptor activation by regulated cleavage plays a role in signaling by other cell surface proteins. For example, the γ-secretase complex has been shown to cleave the EGF tyrosine kinase receptor ErbB4 and the adherens junction protein E-cadherin. In the case of ErbB4, this cleavage event is required for the normal proapoptotic effects of receptor activation, arguing that some cell outcomes previously ascribed to activation of tyrosine kinase cascades may in fact be due to receptor cleavage, and subsequent direct regulation of nuclear transcriptional events. While the nuclear targets of some of these cleaved receptors remain to be determined, the likely importance of this pathway in normal cell signaling is emphasized by the finding that the HGF receptor c-Met undergoes a similar cleavage event that regulates cell survival signaling.
Recently recognized targets of regulated intramembrane proteolysis in the kidney include the proximal tubule scavenger receptor megalin, as well as polycystin-1. In the proximal tubule, megalin can undergo ligand-dependent γ-secretase mediated cleavage, leading to release of the megalin intracellular domain that in turn downregulates mRNA expression for both megalin itself and the Na + /H + exchanger 3 (NHE3). Polycystin-1, the protein product of the PKD1 gene that is defective in the majority of patients with autosomal dominant polycystic kidney disease (ADPKD), has also been shown to undergo RIP, releasing an intracellular carboxy-terminal tail (CTT) that is believed to traffic to the nucleus, where it can regulate gene expression. γ-Secretase-mediated cleavage of polycystin-1 to generate the CTT appears to be dependent on the presence of polycystin-2, as well as mechanical stimuli such as those that might occur with urinary flow.
Receptors that Signal Cell Location
One of the most important roles of cell signaling is to organize when a given cell should undergo differentiation toward a highly specialized function (such as the increase in sodium transporters in the brush border of a proximal tubule cell in response to angiotensin II signals mediated by volume depletion ) or should revert to more basic processes such as cell division and migration (e.g., during development of the embryonic kidney or recovery of the adult kidney from acute kidney injury). While these widely divergent responses are primarily mediated by receptor–ligand interactions such as those mentioned above, cells also have surface proteins that provide important clues regarding cell location and density, and thus establish their level of differentiation, polarity, and responsiveness to outside signals.
Cell–Matrix Interactions can Signal Cell Location
The cells of the nephron reside on a complex basement membrane that provides specific clues regarding cell location. In the glomerulus this structure is highly specialized to not only support epithelial cell attachment (the podocyte), but also endothelial cell attachment, and to serve as a significant component of the glomerular filtration barrier. The basement membrane of the kidney has been shown to be composed of multiple matrix proteins, including collagen, laminin, perlecan, nidogen, nephronectin, and entactin. The specific isoforms and relative contributions of these proteins vary during the course of renal development, as well as along the length of the adult nephron (reviewed in ). These matrix proteins interact with members of a large family of specific cell surface receptors, the heterodimeric α/β integrins.
In the kidney, α 1 β 1 , α 2 β 1 , α 3 β 1 , α 6 β 4 , α 8 β 1 , and α V β 3 have been found to be highly expressed in developing and/or adult renal tubular cells (reviewed in ). The binding of the heterodimeric integrin complex to its matrix ligand in the basement membrane (α 1 β 1 integrin and type IV collagen, for example) results in clustering of the integrins on the basal surface of the cell at contact sites known as focal contacts or adhesions, and the concomitant accumulation of a large group of intracellular signaling proteins at these sites known as the focal adhesion complex. This complex typically includes the focal adhesion scaffolding proteins paxillin and HEF1, the non-receptor kinases Src, PI 3-K, integrin-linked kinase (ILK) and focal adhesion kinase (FAK), the small G-protein regulated signaling proteins PIX and PAK, and actin-binding proteins such as vinculin, talin, and actopaxin (reviewed in ). Signaling through this complex can occur in a traditional “outside-in” manner, in which integrin binding to matrix results in formation and activation of the signaling complex or in an “inside-out” manner, in which signals from other sites, such as activated growth factor receptors, can regulate the affinity of the integrin complex for its matrix ligand, for example during growth factor-stimulated cell adhesion and/or migration.
Signals emanating from focal adhesions provide critical clues regarding cell location, establishment of cell polarity, regulation of cell proliferation, and determination of cell differentiation. The recruitment of actin-binding proteins into the focal adhesion complex provides important clues for cell polarity, while the regulation of small G-proteins such as Rac and Cdc42 is critical for regulating cell differentiation and directed migration. Focal adhesion signaling through Src, FAK, and the P I3-K are required to normally activate the ERK MAPK pathway in response to proliferative growth factor stimuli, and thus promote entry into the cell cycle and subsequent cell proliferation. In the event that cell–matrix adhesion is lost, such as can occur in proximal tubule cells following ischemic renal injury, growth factor signaling is muted, the cells enter cell cycle arrest and eventually undergo anoikis (programmed cell death induced by cell detachment) due to activation of the JNK MAPK pathway (see “Intracellular Signaling Pathways”).
The importance of providing the right matrix environment for normal kidney development and function has been demonstrated in mice lacking specific matrix proteins and/or integrin receptors. For example, failure to express laminin-10 results in severe abnormalities in glomerular development, as does loss of expression of the laminin-10 receptor α 3 β 1 integrin, while loss of β1 integrin severely impairs branching of the ureteric bud. In contrast, mice lacking integrin α 1 expression demonstrate normal kidney development, but have increased fibrosis after glomerular injury, due to an increase in reactive oxygen species (ROS) generation ( Figure 13.7 ).

Cell–Cell Interactions such as Adherens Junctions and Gap Junctions can Signal Cell Density and allow Cells to act in Concert
A second means by which cells obtain clues about their immediate environment is via cell–cell interactions. Of the many types of cell–cell interactions, at least three, adherens junctions, tight junctions, and the gap junctions, play important roles in cell signaling. Adherens junctions form at the lateral border of adjacent cells due to the intercellular interactions of cadherins, a family of cell type specific transmembrane proteins (reviewed in ). There are multiple cadherin family members, including the classic epithelial cell member E-cadherin, the endothelial cell cadherin VE-cadherin, and the renal tubule associated cadherin Ksp-cadherin. The extracellular portion of cadherins contains five repeat sequences (known as EC repeats), that can interact in a homophilic, calcium-dependent manner with the EC repeats present on cadherins in adjacent cells. This interaction is important for providing cell sorting signals during tissue development.
The intracellular domain of the cadherins associates with a group of cytoplasmic proteins known as the catenins. One of these proteins, β-catenin, has a dual role in the cell. It directly binds to cadherins, and thus participates in the formation of cell–cell junctions, but it can also disassociate from adherens junctions and translocate to the nucleus, where it regulates signaling events involved in cell differentiation and proliferation. Originally it was believed that the direct interaction of cadherins with β-catenin created a stable binding site for the actin-binding protein α-catenin, thus generating a static site for lateral attachment of the actin cytoskeleton. However, more recent studies have demonstrated that this protein complex is a dynamic structure that can support actin filament rearrangement during the movement of cells, while maintaining cell–cell junctional integrity.
β-Catenin Signaling can Regulate Cell Differentiation and Proliferation
As noted, β-catenin can leave the adherens junction and enter the nucleus, where it acts as a transcriptional regulator by binding to the TCF/Lef transcriptional complex. Genes that are induced downstream of β-catenin typically lead to increased cell proliferation and regulation of differentiation, events that are important during normal development, but that are typically downregulated in the adult. This transcriptional activity of β-catenin is tightly regulated by controlling the free cytosolic pool of β-catenin that is available for translocation into the nucleus. In the adult renal tubule, the extensive array of intercellular adherens junctions that forms in the confluent monolayer of epithelial cells results in sequestration of the majority of β-catenin with cadherin. To further ensure that free β-catenin levels remain low in the cytosol, a serine-threonine kinase, glycogen synthase kinase-3β (GSK-3β), phosphorylates cytosolic β-catenin and targets it for degradation by the proteosomal pathway. GSK-3β is associated with the adenomatous polyposis coli (APC) protein, and mutations in this complex that prevent β-catenin phosphorylation and degradation lead to increased nuclear β-catenin signaling, cell proliferation, and subsequent tumor formation.
During organ development, and following some types of organ injury, β-catenin nuclear signaling is activated by destabilization of adherens junctions (thereby releasing β-catenin into the cytoplasm) and coincident inhibition of GSK-3β kinase activity. The classic developmental regulator that has been found to activate β-catenin signaling in this manner is the growth factor Wnt and its receptor Frizzled (Fz) ( Figure 13.8 ). Frizzled is a member of the GPCR family of seven membrane spanning cell surface receptors, and has been proposed to signal, at least in part, by activation of heterotrimeric G-proteins. However, in the canonical Wnt signaling pathway, binding of Wnt to Fz leads to the GSK-3β-dependent phosphorylation of a second membrane spanning protein, the Low-density lipoprotein Receptor-related Proteins 5 and/or 6 (LRP5/6), which in turn mediate the recruitment/activation of the cytosolic protein dishevelled (Dsh). This complex appears to inhibit GSK-3β-dependent phosphorylation of β-catenin and other substrates in the cytosol by sequestering GSK-3β into multivesicular endosomes, thus preventing β-catenin degradation.

During kidney development, Wnt4 and Wnt9b, acting at least in part via activation of β-catenin, have been shown to be required for both maintenance of the nephron progenitor pool and normal differentiation of these progenitor cells to form the nephron, suggesting that careful titration of the level of activation of canonical Wnt signaling is critical during mesenchymal-to-epithelial differentiation. In addition, multiple Wnts are upregulated following kidney injury, where they appear to play a role in normal repair, as well as in the promotion of fibrosis.
Podocyte Slit Diaphragms are Highly Specialized Tight Junctions that Signal to the Cystoskeleton
Tight junctions are cell–cell junctions that are typically located at the interface of the apical and basolateral membranes of epithelial cells, where they serve to regulate the composition of the fluid that moves between cells into the interstitial space. In glomerular podocytes, tight junctions have evolved into elaborate structures that support the extensive interdigitation of adjacent cells along the glomerular basement membrane, and thus constitute a critical component of the glomerular filtration barrier. These cell–cell junctions are termed slit diaphragms, and form when Ig-like domains in the extracellular portion of the transmembrane proteins nephrin and Neph1 form homotypic and heterotypic interactions with nephrin/Neph1 molecules in the adjacent podocyte. Dynamic regulation of these interactions is critical for maintaining the integrity of the slit diaphragm, which is achieved by signaling from the slit diaphragm to the actin cytoskeleton (reviewed in ). This signaling involves phosphorylation of the intracellular domain of nephrin by the Src-family kinase Fyn, followed by recruitment of a signaling complex including the adaptor proteins Nck1/2, Grb2, and p85, which in turn mediate recruitment/activation of cytoskeletal regulatory proteins including WASP, Pak, and Rac that regulate actin polymerization/depolymerization in the foot process. Mutations that alter nephrin/NEPH interactions lead to disruption of this intracellular signaling complex, destabilization of the actin cytoskeleton and foot process retraction/fusion.
Gap Junctions Promote Rapid Signaling Between Groups of Cells
A second type of cell–cell interaction that is important for cell signaling is the gap junction. These junctions are formed by the alignment of hemichannels on the lateral borders of two adjacent cells to establish a direct cytoplasmic link between the cells, thus allowing the rapid movement of small molecules and electrical charge through multiple cells within a specified region of the organ. Gap junctions are primarily composed of a family of proteins known as connexins, and have traditionally been studied for their ability to rapidly transmit contraction signals through muscles. Investigation of gap junction function within the kidney has demonstrated that mesangial cells contain large numbers of gap junctions comprised of connexin 43 (Cx43), and that these are critical for mediating intercellular calcium-dependent coordinated mesangial contraction. In addition, tubular epithelial cells maintain intercellular gap junctions that can be regulated by growth factors, as well as by ischemic injury, although the precise role of these channels in normal tubule function is presently not well-understood.
The Cilia as a Signaling Structure
Many cells of the body, including renal epithelial cells, have a surface structure known as the primary cilium. Cilia are elongated membrane protrusions that surround a central core of microtubules arising from a microtubule organizing center known as the basal body (reviewed in ). Cells that express cilia with a microtubular arrangement of 9+2 (9 microtubule doublets arranged in a cylinder around a core of 2 microtubule singlets), such as those lining the trachea, are motile and can act to facilitate directional movement of fluid (reviewed in ). In other cells, such as those lining the renal tubules, cilia have a 9+0 arrangement, are non-motile, and were previously believed to be rudimentary structures. However, the finding that genetic mutations that interrupt cilia formation can result in cystic kidney diseases in rodents, along with the recent discovery that the two predominant gene products known to cause human autosomal dominant polycystic kidney disease, polycystin-1 (Pc-1) and polycystin-2 (Pc-2), localize to cilia, has resulted in intense investigations into the role of non-motile cilia as renal epithelial mechanosensors.
These studies have demonstrated that Pc-2 acts as a cation channel, and that regulation of this channel activity can be mediated by its interaction with Pc-1. In vitro studies have demonstrated that Pc-1 and Pc-2 co-localize on the primary cilium of the apical cell membrane in renal epithelial cells, and that physiological levels of fluid shear stress, such as that created by urine flow in the renal tubule, may be sufficient to stimulate cilia-dependent Pc-1/Pc-2-mediated calcium signaling. It is presently hypothesized that failure of this signaling pathway can result in abnormalities in both the rate and organization of cell proliferation, and thus can lead to cyst formation.
Intracellular Signaling Pathways
As is clear from the preceding section, activation of cell surface receptors results in the regulation of multiple intracellular signaling pathways. Although numerous studies from the past decade have emphasized the vast amount of cross-talk between the proteins involved in these pathways, it remains useful to identify core signaling cascades that can transduce signals from the receptor to effector proteins that mediate specific cellular responses. Several of these signaling cascades, including the heterotrimeric G-protein-adenylate cyclase-cAMP-PKA pathway, the TGFβ-Smad pathway, and the Wnt-Fz-Dsh-Gsk3β-β-catenin pathways, have been described in some detail in the section “Cell Surface Receptors.” This section will focus on several other signaling cascades that are believed to be fundamental regulators of cell survival and function in the kidney, including the PLC-Ca-PKC pathway, the MAPK pathway, and the PI 3-kinase pathway.
The Phospholipase C Pathway Regulates Intracellular Calcium Release and Activates PKC Signaling
Phospholipase C (PLC) is an enzyme that catalyzes the hydrolysis of the membrane lipid phosphoinositide 4,5 bisphosphate (PI 4,5 P 2 ) to generate diacylglycerol (DAG) in the membrane and release inositol trisphosphate (IP 3 ) into the cytoplasm (reviewed in ). DAG provides a binding site to recruit protein kinase C (PKC) to the membrane, while IP 3 binds to its receptor on the endoplasmic reticulum that mediates the intracellular release of stored calcium. Thus, activation of PLC regulates both PKC-dependent and calcium-dependent intracellular signaling.
In mammals, there are four known families of phospholipases C, PLCβ, PLCγ, PLCδ, and PLCε. While all four groups share the catalytic X and Y lipase domains, the regulatory domains are widely divergent, allowing activation by distinct upstream receptors. For example, PLCβ is activated following stimulation of certain GPCRs, because it has a carboxy terminal domain that recognizes and binds GTP-loaded G qα , as well as the free βγ heterodimer (see section “G-protein coupled receptors”). Activation of PLCδ and PLCε are less well-understood, although each appears to be mediated by interaction with small GTP-binding proteins. PLCδ can be activated by associating with the GTPase Ral, whereas PLCε has a Ras-binding domain and can be activated by associating with GTP-loaded Ras.
In contrast, PLCγ family members lack the Gα- and βγ-binding regions, but instead encode two SH2 domains and one SH3 domain that mediate their recruitment and activation by receptor tyrosine kinases (RTKs) such as the PDGF receptor, vascular endothelial growth factor (VEGF) receptor, and HGF receptor. Interestingly, PLCγ can also be phosphorylated and activated by non-receptor protein tyrosine kinases, such as Src family members. In this manner, PLCγ can be secondarily activated in immune cells downstream of T-cell receptor activation (see Figure 13.5 ), as well as following activation of certain GPCRs, such as the angiotensin II receptor.
For PLC to hydrolyze PI 4,5 P 2 , it must be recruited to the membrane. Members of both the PLCβ and PLCγ families have pleckstrin homology (PH) domains at their amino termini that promote membrane association by binding to select membrane phospholipids, such as PI 4,5 P 2 and PI 3,4,5 P 3 . PLCβ family members are further stabilized at the membrane because their PH domain can also interact with the membrane bound βγ G-protein heterodimer, while the SH2 domains of PLCγ proteins enhance membrane association by mediating recruitment to cell surface receptors. In this manner, PLC is recruited to specific sites at the membrane in the vicinity of the activating receptor, allowing the cell to selectively upregulate DAG and IP 3 production in that area.
PLC-dependent generation of DAG provides a membrane binding site for recruitment and activation of several members of the protein kinase C (PKC) family of non-receptor serine-threonine kinases ( Figure 13.9 ). PKCs are a large group of proteins that are subdivided into the conventional PKCs, novel PKCs, and atypical PKCs. The conventional PKCs (PKCα, PKCβ, PKCγ) are activated in a calcium-dependent fashion following recruitment to the cell membrane by binding to DAG and phospholipids such as phosphatidylserine (PS). The novel PKCs (PKCδ, PKCε, PKCη, and PKCθ) are also recruited to the membrane by binding to DAG and membrane phospholipids, but do not require calcium for activation. The atypical PKCs (PKCλ, PKCζ, PKCμ, and PKCι) lack both the DAG and calcium binding sites, and instead appear to be associated with the membrane and activated solely via their association with membrane phospholipids (reviewed in ). In addition to PS, it has been found that the 3-phosphorylated lipid products of the PI 3-K (such as PI 3,4 P 2 and PI 3,4,5 P 3 ) can bind and activate both novel and atypical PKCs.

Once activated, PKCs have multiple potential phosphorylation targets in the cell. The determination of which targets are phosphorylated is dependent on cell type, the isoform of PKC that is activated, and targeting proteins that specify subcellular localization of the activated PKC. Proteins that are not phosphorylation substrates of PKC, but serve only to target specific PKCs to select sites in the cell, are collectively termed RACKs ( r eceptors of a ctivated C k inase). For example, the cell polarity proteins Par3 and Par6 associate with atypical PKCs, such as PKCζ, and specifically target them to epithelial tight junctions on renal tubular cells. In this location, PKCζ has been shown to regulate both tight junction assembly and disassembly, although the exact phosphorylation targets of PKCζ have yet to be identified.
Recent studies have demonstrated that PKC localization to the basolateral membrane can regulate Na,K-ATPase activity in the renal tubule as well. Several phosphorylation sites for classical PKCs (such as PKCα) have been identified in the amino terminus of the Na,K-ATPase α-subunit, and phosphorylation of these sites appears to increase cellular sodium pump activity by increasing membrane localization of the enzyme. Interestingly, activation of PKC downstream of the D 1 -type dopamine receptors appears to have the opposite effect, inhibiting sodium pump activity as part of the overall effect of the D 1 receptor in inhibiting tubular sodium reabsorption. Exploration of this response has demonstrated that novel PKCs such as PKCθ and PKCε are likely to mediate this Na,K-ATPase inhibitory effect. It is unknown whether these PKCs phosphorylate different sites on the sodium pump than PKCα or act indirectly via phosphorylation of intermediate proteins such as the sodium hydrogen regulatory factor Nherf-1.
Another group of PKC regulatory targets are transcription factors. PKC isoforms such as PKCδ, PKCε, and PKCθ have been found to regulate the activity of multiple transcription factors, including NF-κB (involved in immune and inflammatory responses), s ignal t ransducers and a ctivators of t ranscription (STATs, regulators of inflammatory responses, cell proliferation, and differentiation), and J un N -terminal k inase (JNK, involved in cell stress response and survival) (reviewed in ). By acting upstream of JNK as well as the Raf-MEK-ERK pathway (see below), PKC isoforms can cooperate to mediate increased activity of the immediate early response genes Jun and Fos.
An interesting example of convergence of PKC with other signaling pathways is seen during the activation of T-cells. The rise in intracellular calcium following T-cell stimulation results in the activation of calmodulin and binding of the calcium–calmodulin complex to the non-receptor serine-threonine phosphatase calcineurin (also known as protein phosphatase 2B or PP2B). Activated calcineurin dephosphorylates and activates the nuclear translocation of another protein, n uclear f actor of a ctivated T -cells or NFAT (reviewed in ). While originally described in T-cells, NFATs are expressed in multiple cell types and control the expression of genes such as Il-2, GM-CSF, interferon-γ, TNFα, and Cox2 that regulate processes as diverse as T- and B-cell proliferation in response to antigen stimulation, cardiac myocyte differentiation and hypertrophy, and sodium channel expression (reviewed in ). However, the DNA-binding sites of many of these gene targets contain nearby AP-1 promoter sites, and are only upregulated in an efficient manner following the concerted actions of NFAT and the AP-1 binding elements Jun and Fos. Thus, concerted activation of PKC (to activate Jun and Fos) and calcineurin (to activate NFAT) leads to maximal gene expression and cellular response. The importance of calcineurin in mediating immune cell activation has led to the extensive use of calcineurin inhibitors, such as cyclosporine and tacrolimus, for the prevention of transplant rejection.
The Mitogen Activated Protein Kinase (MAPK) Pathway Regulates Cell Survival, Proliferation, and Morphology
The MAPK pathway provides an excellent example of the way in which different extracellular signals can converge on the regulation of a single intracellular signaling pathway, and demonstrates how targeting of that pathway to specific sites in the cell via scaffolding proteins can determine which effector proteins are regulated, and what cell responses are affected. As the name implies, this protein cascade was originally identified based on its activation downstream of pro-proliferative growth factors such as insulin and EGF. In the classic MAPK cascade, binding of the growth factor to its receptor tyrosine kinase (RTK) initiates a series of protein–protein interactions that ultimately result in activation of the cytosolic serine-threonine kinase ERK, which can phosphorylate and regulate diverse effector substrates including transcription factors in the nucleus, focal adhesion proteins at the cell surface, and contractile proteins in the cytosol.
The core proteins of this classic MAPK cascade are three kinases, Raf, MEK, and ERK. Raf-1 (also called MEK kinase (MEKK) or MAPK kinase kinase (MAPKKK)) is a serine-threonine kinase that phosphorylates and activates two closely related MEK isoforms, MEK1 and 2. MEK1/2 are dual specificity (tyrosine as well as serine/threonine) kinases that phosphorylate ERK1 and 2 on a highly conserved amino acid motif, Thr-Glu-Tyr, contained in the activation loop of the protein. The efficient activation of ERK in this cascade requires that the three proteins (Raf, MEK, and ERK) are brought into close proximity on a single scaffolding protein. Present studies indicate that several different proteins can serve this scaffolding function, including β-arrestin, IQGAP, kinase suppressor of Ras (KSR), and paxillin. The location of the scaffolding protein and the regulation of Raf/MEK/ERK association determines which effector proteins are likely to be regulated (reviewed in ).
The core module of Raf, MEK, and ERK can be activated following binding of receptor tyrosine kinases to their extracellular ligands. The initial step in RTK-mediated MAPK activation is the recruitment of the GRB2 adaptor protein to the tyrosine phosphorylated receptor. GRB2 is a small molecule that is composed of one SH2 domain and two SH3 domains. As noted previously, proteins containing SH2 domains interact with other proteins that contain phosphorylated tyrosine residues flanked by the appropriate amino acids. The GRB2 SH2 domain preferentially binds to phosphotyrosine residues with an asparagine at the +2 position, such as tyrosine 1096 in the activated c-Ret receptor (pYA N W) or tyrosine 1356 in activated c-Met (pYV N V). In contrast, SH3 domains typically mediate constitutive association with short proline-rich sequences in target proteins. The g uanine nucleotide e xchange f actor (GEF) Sos contains such a sequence, and associates with the GRB2 SH3 domain in a constitutive fashion. Sos acts as a GEF for the membrane-associated small GTP-binding protein Ras.
Ras is structurally similar to the α-subunit of the αβγ heterotrimer that associates with GPCRs. However, Ras is activated by non-GPCR GEFs such as Sos and, in the GTP-bound state, associates with and activates Raf rather than adenylyl cylase. This activation step appears to involve the Ras-dependent dephosphorylation of Raf by PP2A, a non-receptor protein phosphatase. Thus, RTK activation results in recruitment of the GRB2-Sos complex to the membrane where it mediates GTP-loading of Ras, and activation of the Raf-MEK-ERK signaling pathway ( Figure 13.10 ).
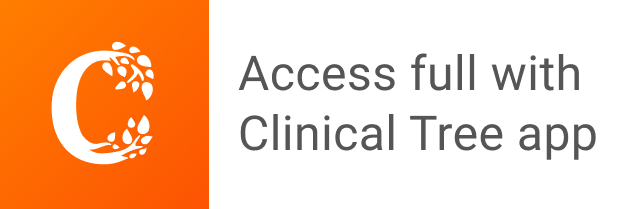