Abstract
Potassium levels in the blood are tightly regulated. Deviations from this normal range can be life-threatening, and treatment should be based on an understanding of the underlying physiology and pathophysiology of potassium metabolism. Here we discuss normal potassium metabolism, with specific emphasis on renal potassium handling. We further discuss the clinical assessment of abnormalities of blood potassium levels to guide rational treatment.
Keywords
potassium, hyperkalemia, hypokalemia, KCNJ1, aldosterone, KCNJ10
- •
Abnormalities in Plasma Potassium Can Reflect Changes in Internal Balance (Shift Across the Cell Membranes) or External Balance (Discrepancy Between Intake and Excretion of Potassium)
- •
Changes in Internal Balance Are Typically Acute, Whereas Disturbance of the External Balance Is More Likely to Result in Chronic Changes
- •
The Kidneys Are the Key Organs for Maintaining the External Balance by Adjusting Excretion to Intake
- •
The Distal Convoluted Tubule Is Critical for Potassium Sensing, Whereas the Aldosterone-Sensitive Nephron Is Responsible for Potassium Secretion
Normal Metabolism
General Background
Potassium is the most abundant cation in the human body, yet only 2% of total body potassium is contained in the extracellular fluid, which is the compartment accessible to clinical assessment. Its concentration in extracellular fluid is tightly regulated between 3.5 and 5.0 mmol/L. The vast majority of potassium (98%) is located intracellularly (mainly in muscle) at concentrations between 100 and 150 mmol/L, depending on cell type ( Fig. 3.1 ). This large difference between intracellular and extracellular potassium content is of great importance to maintain the resting membrane potential of excitable cells, such as neurons, muscle cells, and cardiac cells. In addition, redistribution between intracellular and extracellular potassium provides a first defense mechanism against hyperkalemia or hypokalemia. The normal distribution of total body potassium is shown in Fig. 3.1 .

To maintain normokalemia, there needs to be a strict control between the regulation of the internal and external potassium balance. The first refers to the concentration gradient across cell membranes caused by the difference between intracellular and extracellular potassium levels, which is maintained by the influx or efflux of potassium from intracellular to extracellular spaces in different conditions. The latter refers to the regulation of total body potassium, by balancing potassium excretion with potassium intake. Although total body homeostasis mainly relies on the excretion of potassium by the kidneys, the response of the kidneys after having a meal rich in potassium is relatively slow. Therefore a transient but rapid translocation of the excess potassium from the extracellular into the intracellular space is needed to prevent potential life-threatening hyperkalemia to occur, thus showing that the internal and external potassium balance is constantly interacting with each other.
Regulation of Internal Potassium Balance
Because of the high gradient between intracellular and extracellular potassium levels, potassium constantly leaks out of the cell via ubiquitously expressed selective potassium channels, which helps establish a voltage gradient across the membrane. This passive potassium efflux is counteracted by active transport of potassium into the cell by Na + ,K + -ATPase, which pumps two potassium ions into and three sodium ions out of the cells, at the expense of the hydrolysis of one adenosine triphosphate (ATP) molecule. Factors that can influence this balance are shown in Table 3.1 .
Factors That Increase Intracellular Potassium (Decreasing Serum Potassium) | Factors That Decrease Intracellular Potassium (Increasing Serum Potassium) |
---|---|
Physiologic Factors | |
Insulin | |
Aldosterone T3 | |
β-adrenergic stimulation | α-adrenergic stimulation |
Pathologic Factors | |
Alkalosis | Acidosis |
Hyperosmolality | Hypoosmololality |
Hyperglycemia/uncontrolled diabetes mellitus | |
Heavy exercise | |
Cell lysis |
After ingestion of a meal, approximately 80% of the potassium that is absorbed by the gut is rapidly translocated into the intracellular space. This rapid uptake of potassium is mainly induced by increased insulin levels after the absorption of glucose and amino acids. Insulin increases the activity of the Na + ,K + -ATPase in hepatocytes, muscle cells, adipose cells, and brain cells, thereby increasing their potassium uptake. This process is independent of its effect on glucose transport. Meanwhile, any rise in serum potassium levels will decrease the transmembrane potassium gradient, thereby decreasing the passive efflux of potassium out of the cells. Increased potassium intake also stimulates the secretion of aldosterone, which further stimulates potassium excretion by both the kidneys and the colon. Triiodothyronine (T3) increases Na + ,K + -ATPase in muscle cells, increasing their potassium uptake. β-Adrenergic stimulation promotes cellular potassium uptake by liver cells, muscle cells, and cardiac cells. In contrast, α-adrenergic stimulation promotes the efflux of potassium from hepatocytes.
Besides the abovementioned hormonal influence on internal potassium balance, extracellular potassium levels can also be affected by several pathologic conditions. These include disturbances of acid-base homeostasis, because serum pH inversely correlates with serum potassium levels. In conditions causing altered serum pH, sodium and potassium are exchanged for hydrogen. Moreover, hydrogen ions impair the activity of Na + ,K + -ATPase, thus decreasing intracellular potassium uptake. Acid-base disturbances due to metabolic conditions cause greater potassium shifts compared with respiratory conditions, as do acute changes compared with chronic acid-base derangements. Changes in serum osmolality also affect extracellular potassium concentration. An increase in serum osmolality shifts water out of the cells, which inevitably leads to an increase of intracellular potassium concentration. This increased transmembrane potassium gradient promotes the passive leakage of potassium out of the cells into the extracellular fluid. In heavy exercise, intracellular ATP depletion impairs potassium uptake into muscle cells, thus increasing serum potassium levels. And lastly, in case of cell death, such as in tumor lysis syndrome, the Na + ,K + -ATPase can no longer function and the intracellular potassium stores are released into the extracellular space.
Regulation of External Potassium Balance
Routes of Potassium Excretion
The regulation of total body potassium is mainly controlled by renal potassium excretion, which is described in more detail later. The average daily potassium intake is approximately 1 mmol/kg per day. Although children need a positive potassium balance to grow, adults maintain a zero potassium balance. Of all ingested potassium, 80% to 90% is excreted by the kidneys and 10% to 20% is lost in the stool.
Potassium can be found in gastrointestinal secretions in various amounts. Saliva contains approximately 20 mmol/L, esophageal and gastric secretions 11 to 35 mmol/L, and pancreatic secretions 3.5 to 5.0 mmol/L. Most of this potassium is reabsorbed throughout the intestinal tract. Meanwhile, the colon is the most important site for potassium excretion. Colonic cells can actively secrete potassium via the basolateral pumps Na + ,K + -ATPase and Na + -K + -2Cl − -cotransporter (NKCC) and the apical potassium “big” conductance (BK) channel. Colonic potassium absorption is mediated by two apical H + -K + -ATPases. The fecal potassium content can be increased by several hormones, including aldosterone, epinephrine, and prostaglandins. Increased colonic expression of BK channels in end-stage renal disease can enhance the fecal potassium excretion to up to 30% to 80% of the ingested amount of potassium. Other pathologic conditions such as secretory diarrhea and ulcerative colitis can also be associated with increased expression or activation of BK channels, thus increasing intestinal potassium losses. Emesis can be another route of potassium loss because it contains approximately 5 times more potassium than serum. Factors that can influence gastrointestinal losses are summarized in Table 3.2 .
Factors That Increase Gastrointestinal Potassium Loss | Factors That Decrease Gastrointestinal Potassium Loss |
---|---|
Physiologic Factors | |
Aldosterone | Low dietary potassium intake |
Epinephrine | |
Prostaglandins | |
Pathologic Factors | |
Secretory diarrhea | |
Vomiting | |
End-stage renal disease | |
Ulcerative colitis | |
Medications | |
Prolonged use of laxatives a | Nonsteroidal antiinflammatory drugs |
Intestinal potassium binders b |
a Mainly diphenolic laxatives (e.g., phenolphthalein and bisacodyl) and anthroquinones (e.g., senna glycosides and danthron).
b Polystyrene sulfonate (resonium) or sodium zirconium cyclosilicate.
The potassium content of sweat is generally twice as high as serum. Yet, given the low volume of daily sweat production, the potassium losses via this route are usually negligible.
Regulation of Potassium Excretion: Circadian Rhythm, Feedback, and Feedforward
The regulation of the daily excretion of potassium occurs via three mechanisms. The first is characterized by a circadian rhythm, which is one of the most consistent circadian processes that occurs in humans. It enables a higher potassium excretion during daytime, when typically more potassium is ingested, thus minimizing swings in serum potassium levels. This circadian rhythm remains present when potassium intake is equally distributed throughout the day and physical activity and light exposure are held constant. Changes in potassium intake can interfere with the amplitude of this rhythm but not with its intrinsic circadian periodicity.
The second mechanism of regulation of potassium excretion is the negative feedback system, which is controlled by serum potassium levels. It relies on both the internal and external potassium balance to maintain stable serum potassium levels after a potassium load, but also after potassium depletion. Mild increases in serum potassium promote the cellular uptake of the cation (e.g., by increased insulin and aldosterone levels) and vice versa, as discussed before in the paragraph on internal potassium balance. Moreover, the urinary excretion of potassium can be regulated according to serum potassium concentration. In general, the absorption of potassium throughout the nephron is quite stable. Changes in urinary potassium content are mainly established by altering the potassium excretion by the principal cell in the late distal convoluted tubule (DCT) and collecting duct. This is explained in more detail later.
A third mechanism of potassium regulation has been postulated in the form of a feedforward system. The exact pathways by which this system operates have not been completely elucidated, but the proposed existence of this feedforward system is based on the observation in animal studies that the kaliuretic response to a potassium load is greater with enteral intake compared with intravenous infusion. This feedforward system appears to be aldosterone independent and is thought to be mediated by receptors in the gut, portal vein, and/or liver, which, via undefined pathways, lead to decreased sodium uptake in the DCT and thus enhanced delivery of sodium to the collecting duct, where it can be reabsorbed in exchange for potassium secretion. This increased urinary excretion of potassium at the moment of enteral potassium intake prevents hyperkalemia when the enteral potassium load is being absorbed.
Renal Potassium Handling
The daily urinary potassium excretion depends on glomerular potassium filtration (determined by glomerular filtration rate [GFR] and serum potassium levels) and potassium reabsorption and excretion along the different sites of the nephron ( Fig. 3.2 ).

Potassium is freely filtered in the glomerulus. Thus, in a normal adult, approximately 755 mmol of potassium reaches the proximal tubule every day (180 L/day × average serum potassium concentration of 4.2 mmol/L). The reabsorption of potassium in the proximal tubule and loop of Henle is rather stable. According to the body’s needs, the most powerful regulation of urinary potassium excretion occurs in the late DCT and collecting duct. In this segment, under normal conditions, potassium will be secreted by the principal cells. The amount of potassium secreted can be increased in situations where there is a high potassium intake, to a level that can even exceed the daily amount of potassium that is filtered by the glomeruli. Besides the principal cells, in this situation the type B intercalated cells are also capable of secreting potassium. In cases of low potassium intake, potassium excretion by the principal cells is reduced and a simultaneously increase in potassium reabsorption by the type A intercalated cells in the same segment will result in a drop of net urinary potassium excretion.
However, this response of the kidney to long-term changes in potassium intake is sluggish. In adults given a diet that is consistently rich in potassium, it takes several days until maximum urinary potassium excretion is established. In contrast, in adults who are deprived of potassium, the minimum urinary loss of potassium will still be approximately 10 mmol/day and can thus cause hypokalemia in prolonged potassium deprivation. This is a well-described complication in patients who are starved after abdominal surgery and are given intravenous fluids without addition of potassium.
Proximal Tubule
Approximately 65% of the filtered potassium is reabsorbed in the proximal tubule, which is similar to the absorption of sodium and water in this segment. This is a passive transport, occurring both by diffusion and by paracellular solvent drag. The latter is a result of active sodium transport, often coupled to the transport of other solutes like glucose or amino acids, causing paracellular hypertonicity. This local osmotic force is driving water reabsorption, with potassium being carried along in the reabsorbate. For this sodium transport to occur and also for bicarbonate to exit the cell on the basolateral side, an electrogenic gradient is needed. This gradient is provided by potassium movement across the respective membranes through potassium channels that have not yet been well defined. Because of the combined reabsorption of organic solutes and sodium in the early proximal tubule, the luminal fluid in this part will have a negative charge. In the late proximal tubule, there is a higher lumen to blood chloride concentration gradient, causing a diffusion potential in which chloride diffuses across the paracellular pathway into the blood, thus causing the luminal fluid to become positively charged. This will provide a driving force for passive paracellular potassium absorption ( Fig. 3.3A ).

Thick Ascending Limb of the Loop of Henle
In this part of the nephron, approximately 20% to 25% of the total amount of filtered potassium is being absorbed by NKCC in the apical membrane. This furosemide-sensitive transporter translocates one sodium ion, one potassium ion, and two chloride ions into the cell. It is mainly driven by a low intracellular sodium concentration, which is maintained by the basolateral Na + ,K + -ATPase. For NKCC to be able to transport potassium into the cell, it needs persistent availability of luminal potassium. This is provided by the renal outer medullary potassium (ROMK) channel, which recycles the potassium back into the tubular lumen. In this way a lumen-positive transepithelial voltage is generated, which is driving the paracellular reabsorption of sodium, potassium, calcium, and magnesium (see Fig. 3.3B ).
Distal Nephron
Insights garnered from the rare inherited Gordon syndrome, or pseudohypoaldosteronism (PHA) type 2, have identified the DCT as critical for the regulation of potassium excretion. Although potassium secretion itself is occurring in the collecting duct, it is greatly dependent on the delivery of sodium: sodium is passively transported across the luminal membrane of the principal cell along the electrochemical gradient via the epithelial Na channel (ENaC). This uptake of sodium creates lumen negativity, which enables potassium secretion via potassium channels. These include the high-conductance BK channels and the small-conductance secretory potassium (SK) channel, the latter consisting of ROMK (see Fig. 3.3C ). Although potassium secretion via BK channels depends mainly on tubular flow rate, the potassium secretion via ROMK is more continuous and greatly depends on the electrochemical gradient provided by ENaC-medicated sodium reabsorption. Thus, by regulating sodium delivery to the collecting duct, potassium secretion can be adjusted: with increased sodium reabsorption in the DCT, little sodium is delivered downstream and thus potassium secretion is impaired. Conversely, if sodium transport in DCT is inhibited, distal sodium delivery is increased, enabling potassium secretion. The fine-tuning of renal potassium excretion is regulated by several factors ( Table 3.3 ). These obviously include aldosterone, which enhances sodium transport through ENaC. However, the observation that aldosterone apparently can enhance potassium secretion without sodium retention (and vice versa), created the so-called “aldosterone paradox.” A solution was provided by the discovery of mutations in WNK4 as a cause of Gordon syndrome. This kinase regulates activity of Na-Cl-Cotransporter (NCC), the sodium transporter in the DCT, as well as paracellular chloride flux and ROMK, so that under conditions of sodium and potassium excess, sodium reabsorption can be decreased, while maintaining potassium secretion (electrically balanced by chloride efflux). Conversely, in conditions of sodium and potassium depletion, sodium reabsorption is enhanced (via NCC) without stimulating potassium secretion.
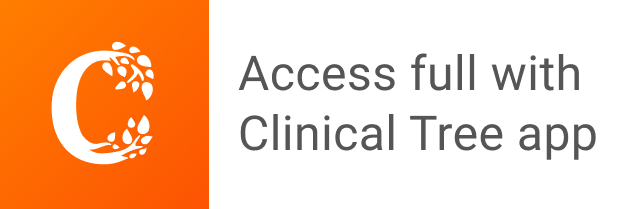