DEFINITION OF OXIDANT STRESS
Oxidative stress is a pathologic state in which reactive oxygen intermediates (ROI) or reactive oxygen species (ROS) can cause oxidation of cellular and matrix macromolecules including sugars, proteins, deoxyribonucleic acid (DNA) bases, and lipids (1). ROS are generated in mitochondria from the use of molecular oxygen for the efficient cellular energy production for routine aerobic metabolic activity. ROS are the detrimental by-products resulting in oxidative damage to cellular organelles and have been associated with several disease states, including cancer, diabetes, Alzheimer’s disease, Parkinson’s disease, aging, inflammation, and atherosclerosis (2,3). Oxidative stress is an imbalance between the quantities of oxidants produced during normal metabolism of our cells and the quantities of antioxidant gene products [superoxide dismutase (SOD), catalases (CATs), glutathione (GSH) peroxidases, etc.], required to maintain the equipoise to prevent accumulation of oxidant molecules.
Increasing evidence suggests that oxidative stress may have a significant role in the development of complications in patients with chronic kidney disease (CKD). Understanding the role of oxidant stress in the pathogenesis of multisystem involvement in patients with CKD or dialysis-associated pathology will ultimately help to develop the strategies for the prevention and treatment of increased oxidative stress in CKD and its associated complications.
GENERATION OF REACTIVE OXYGEN SPECIES IN THE BIOLOGIC SYSTEM
In biologic systems, cells generate energy aerobically to produce adenosine triphosphate (ATP) by reducing molecular oxygen (O2) to water. The cytochrome-C oxidase-catalyzed reactions involve transfer of four electrons (e−) to oxygen; the consumption of oxygen at the mitochondrial level is associated with production of oxygen intermediates. One percent to 2% of total oxygen consumption may, in fact, be converted to superoxide anion radical (O2−). Formation of O2− anion radical leads to a cascade of other ROS production (4) (FIGURE 19.1). The biologic toxicity of superoxide is due to its capacity to inactivate iron-sulfur containing enzymes (these enzymes are critical for different metabolic pathways) and liberate free iron in the cell, which can undergo Fenton chemical reaction and generate highly reactive hydroxyl radicals (OH−). Hydroxyl radical immediately develops into HO2 (hydroperoxyl radical), HO2 in combination with superoxide anion can initiate lipid peroxidation of polyunsaturated fatty acids, react with carbonyl compounds and halogenated carbons to create toxic peroxy radicals. Superoxide reacts avidly with nitric oxide (NO) to form peroxynitrite (ONOO−). As such, superoxide in combination with HO2, and ONOO− are the main causes of oxidative stress.
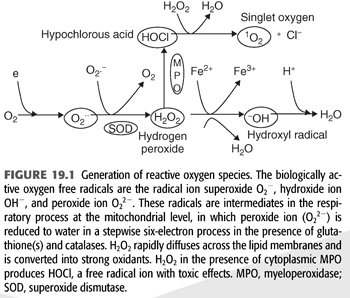
Superoxide dismutase (SOD) rapidly converts O2− to hydrogen peroxide (H2O2) and oxygen. Because O2− is more toxic than H2O2, its rapid removal is of paramount importance for the proper function of the microenvironment (5). H2O2 is a versatile ROS because it can cross plasma membranes, increase intracellular hydroxyl radicals, trigger peroxidation of cell membrane lipids (CMLs), promote protein aggregation, and damage and/or cleave DNA (5,6). H2O2 converts carboxylic acid (RCOOH) into peroxy acids (PRCOOH), which are used as oxidizing agents. H2O2 reacts with acetone to form acetone peroxide, and it interacts with ozone to form hydrogen trioxide. Its reaction with urea produces carbamide peroxide. In aqueous solution, hydrogen peroxide can oxidize or reduce a variety of inorganic ions. When H2O2 acts as a reducing agent, oxygen gas is also produced. In acid solution, Fe2+ is oxidized to Fe3+ (EQUATION 19.1).

Also, through catalysis, H2O2 can be converted into hydroxyl radicals (.OH), the most powerful ROS in the biologic system. H2O2 is removed by several different pathways in vivo.
1. Catalase (CAT) and the reduced form (GSH) of glutathione peroxidase (GPx) catalyze H2O2 to H2O + O2. This is presumably a detoxification mechanism.
2. H2O2 is converted by the abundant myeloperoxidase (MPO) enzyme in neutrophils to hypochlorous acid (HOCl) (6). HOCl is a strong oxidant that acts as a bactericidal agent in phagocytic cells. Reaction of HOCl with H2O2 yields singlet oxygen (1O2) and H2O.
3. H2O2 also reacts spontaneously with intracellular iron to form highly reactive hydroxy radical (OH−) (7) by the Haber-Weiss cycle or Fenton reaction (8). The hydroxyl radical reacts instantaneously with the hydrogen moiety of other biologic molecule to produce long-lived ROS called reactive amines (RH) (9).
4. Superoxide (O2−) anion regulates the bioavailability of NO. Superoxide anion can react with NO and result in the formation of peroxynitrite (ONOO−) (9). ONOO− is a strong oxidant with vasoconstrictor activities as compared with the vasodilator potential of NO (10). In addition, ONOO− reacts nonenzymatically with arachidonic acid components of phospholipids resulting in the formation of isoprostanes (8-iso-PGF2α), a potent vasoconstrictor with a long half-life.
ROS have a very short life, and excessive production and prolonged exposure of cell constituents to these evanescent radicals can result in oxidative modification of cellular macromolecules. In vivo measurements of ROS-modified cellular macromolecules serve as indirect markers of prevailing oxidative stress. These include products of carbohydrate and protein oxidation (11), lipid peroxidation (12), and nucleic acid oxidation with DNA fragmentation leading to DNA instability and mutation (13).
PHYSIOLOGIC ANTIOXIDANT SYSTEM
An imbalance due to increased production of ROS and the available antioxidants results in the excessive exposure of the tissue macromolecules to ROS, resulting in the production of biomarkers of oxidant stress. Under physiologic conditions, protection against oxidant stress is offered by the presence of different types of antioxidants—enzymatic and nonenzymatic antioxidants (1).
Enzymatic antioxidants include glutathione dismutase (GPx), glutathione reductase (GR) and glutathione transferase (GT), SOD, and CAT. GPx is a selenium-dependent enzyme present in the plasma and red blood cell (RBC) and is mainly produced in the kidneys. GPx exists in three different forms [GST, oxidized glutathione (GSSG), and GSH]. GR uses nicotinamide adenine dinucleotide phosphate (NADPH) to convert GSSG to its reduced form (GSH).
Recent discovery of the crystal structure of nicotinamide nucleotide transhydrogenase (TH) that facilitates the disposal of ROS and prevents the development of oxidative stress at the cellular level has further advanced the basic mechanisms of how ROS are disposed (14). The mitochondrial ROS defense system transforms reactive superoxide anions (O2−) formed by electrons leaking to molecular oxygen during aerobic respiration to hydrogen peroxide (H2O2). H2O2 is detoxified to water by peroxidases that use reduced GSH as a substrate. Cellular levels of GSH are maintained by reduced NADPH-dependent GR (FIGURE 19.2 and EQUATION 19.2).
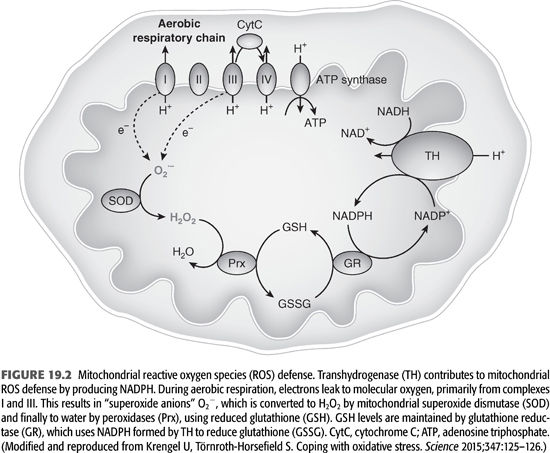

The resultant NADPH is used for amino acid biosynthesis and by GR and GPx to remove ROS (15).
Nonenzymatic antioxidants: Majority of nonenzymatic antioxidants have a thiol as a functional group—SH (reduced) and can be oxidized via disulphide bond formation (oxidized). Thiols have the ability to react with almost all the tissue oxidants and maintain the tissue redox (reduction/oxidation) state. The major biologic thiol/disulphide couples include GSH, thioredoxin, and other cysteine-containing proteins. GSH is particularly unique as it is able to detoxify H2O2, OH−, and chlorinated oxidants. Extracellular thiols present in the plasma and interstitial fluid are important antioxidants to promote redox state. When these protective thiols are decreased as in acute as well as CKD patients, oxidized thiols lead to endothelial injury, and increased vascular morbidity (16).
Other nonenzymatic antioxidants: Important ones include α-tocopherol (vitamin E); vitamin C (ascorbic acid); albumin; and other proteins such as transferrin, ceruloplasmin, and microamounts of extracellular superoxide dismutases (ecSOD) (17). α-Tocopherol (vitamin E) is perhaps the major lipid-soluble chain-breaking antioxidant (reacting with chain-propagating radicals such as peroxyl radicals) (18). This antioxidant is particularly important because it prevents lipid peroxidation. Ascorbic acid, on the other hand, has mostly scavenging properties and other minor antioxidant properties. It scavenges the singlet oxygen (1O2), O2−, and H2O2 at a constant rate at optimal pH (7.4). It is a powerful scavenger of HOCl and also can be a substrate for the enzyme MPO to prevent the generation of HOCl (19). Vitamin C deficiency in CKD and dialysis patients is perhaps more common than thought given that such patients often avoid the consumption of fresh fruits, vegetables, and also loss of water-soluble vitamins with dialysis therapy. However, ascorbic acid in high doses can also be a pro-oxidant (20).
Acute-phase reactive proteins such as ferritin, transferrin, ceruloplasmin, haptoglobin, and others also function as effective antioxidants by binding to transitional metals such as iron, copper, and bromide to prevent the formation of halides, hemes, and chloramines (21).
PATHOPHYSIOLOGY OF OXIDATIVE STRESS IN DIALYSIS PATIENTS
CKD is associated with qualitative and quantitative changes in the physiologic antioxidants. This is further complicated by the increased production or decreased removal of ROS and its related oxidation products. These sequences of events culminate in excessive tissue damage from the ROS and its end products.
Increased Generation of Oxidant Radicals (Reactive Oxygen Species) in Dialysis Patients
Advanced kidney disease is associated with increased production of ROS, and this oxidant stress is further aggravated with the initiation of dialysis therapy. There are several pathways involved in the excessive generation of ROS in CKD and dialysis population.
Activation of neutrophil-macrophage system
1. The increased oxidative stress in CKD as well as in dialysis patients could be due to activation of monocyte-macrophage cells by the uremic milieu and further aggravated by exposure of blood to extracorporeal circulation (see Chapter 20).
Activation of phagocytes results in respiratory burst activity with the production of ROS, necessary for host defenses, cell activation, and cell signaling (22). The respiratory burst activity is associated with activation of mitochondrial NADPH-oxidases that catalyzes the single electron transfer and generates superoxide anion (O2−) at the electron transfer chain level (23). The NADPH-oxidases are highly regulated group of homologous proteins comprising of cytoplasmic (p47, p67, p40) and membrane-bound proteins (gp91 and p22) domains (24). Deficiency or dysfunction of NADPH-oxidases is associated with increased risk of bacterial infections and results in the development of chronic granulomatous disease (CGD). Similar deficiency can lead to unbalanced effects of O2−.
In addition, phagocytic cells have abundant MPO in the azurophilic granules, which is released on stimulation of mononuclear cells. The MPO enzyme system in phagocytic cells catalyzes the oxidation of halides like chloride, bromide, and iodide. MPO in the presence of chloride (Cl−) ions converts H2O2 to HOCl. In addition to its primary function of bactericidal killing, HOCl is a potent oxidant as it can oxidate the thiol groups of membrane proteins and intracellular enzymes, and it also inhibits the mitochondrial cytochrome system, perpetuating the production of ROS. HOCl can react with endogenous amines (R-NHI) to result in the production of long-lasting oxidants called chloramines (RNH-Cl) (6).
Himmelfarb et al. (25) studied phagocytic function in patients on hemodialysis (HD) and peritoneal dialysis (PD) and demonstrated an exaggerated respiratory burst activity of phagocytes after in vitro stimulation. This respiratory burst production of ROS coincides with the peak of complement activation during dialysis treatment (26). Furthermore, Tepel et al. (27) showed that increased ROS production from peripheral blood cells either spontaneously or after phorbol-myristate-acetate (PMA)-induced stimulation in patients on maintenance dialysis, remained unchanged before and after dialysis using biocompatible membranes.
In summary, during the last 10 years, despite the advances in dialysis therapy and introduction of high-performance dialysis membranes and improvements in the quality of dialysates and water purification methods, the rate of progression of chronic inflammation and vascular disease in dialysis-dependent patients has remained unchanged due to inability of conventional dialysis membranes to remove unconventional uremic toxins such as the products of glycation and oxidation (28,29). Therefore, the burden of proinflammatory and pro-oxidant state associated with chronic dialysis therapy has not changed (FIGURE 19.3). On the other hand, evidence supports that the levels of proinflammatory and pro-oxidant levels decrease significantly following kidney transplantation (30).
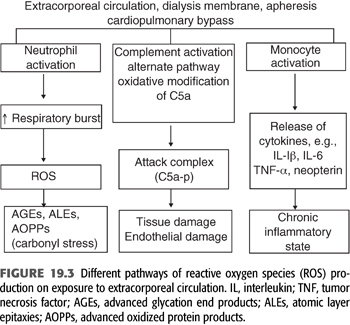
During maintenance dialysis, exposure of monocyte-macrophage system to the uremic solutes along with exposure to extracorporeal circulation, the two in combination, changes the phenotype of these cells to the state of proinflammatory phenotype with unbalanced production of both pro- and anti-inflammatory cytokines. These phenotypic changes result in increased production of adhesion molecules and chemotactic factors such as vascular cell adhesion molecules (VCAM), leukocyte adhesion molecules [intercellular adhesion molecule (ICAM-1) and P-selectins], monocyte chemoattractant protein-1 (MCP-1), and others (31). These changes increase leukocyte activation with migration and adhesion to subendothelial space and to atherosclerotic plaques (32), particularly in the presence of already activated platelets and endothelial cells (33). Cumulatively, these dynamic interactions at the molecular level may contribute to the pathogenesis of accelerated atherosclerosis in dialysis patients (34).
2. Qualitative or quantitative changes in physiologic antioxidants The effects of increased production of ROS in dialysis patients are further aggravated by decreased levels or impaired function of both enzymatic and nonenzymatic antioxidants. Therefore, imbalance between the increased ROS production and reduced availability of antioxidants aggravates the prevailing oxidative stress.
GPx converts H2O2 to 2H2O. GPx is mainly produced in the kidney, and its levels in both serum and RBC decreases in proportion to the decreasing glomerular filtration rate (GFR). All three forms of glutathione (GST, GSSG, and GSH) decrease in patients with advanced kidney disease and in patients on kidney replacement therapy (HD or PD). Ross et al. (35) and Mimić-Oka et al. (36) demonstrated that whole blood and RBC glutathione and CAT levels (CATs also convert H2O2 to H2O and O2) were significantly decreased in patients with kidney disease (CKD) as well as in dialysis-dependent patients.
The levels of nonenzymatic antioxidants like vitamin C and vitamin E have been demonstrated to be low normal in dialysis patients as compared with healthy controls. The reasons for decreased levels of antioxidants in patients with kidney disease are multifactorial: increased consumption resulting from increased burden of ROS, relative deficiency of vitamin C and vitamin E (possibly related to a decreased intake and dietary restriction of fresh fruits and vegetables to avoid hyperkalemia), and the excessive losses of nutrients of different molecular weights with dialysis (37,38). It is also possible that uremic toxins that persist despite an adequate dialysis dose may cause functional deficiency of these physiologic antioxidants. Hypoalbuminemia is often present in dialysis-dependent patients and results in decreased antioxidant stores in blood (37,39).
BIOMARKERS OF OXIDATIVE STRESS
Because ROS have a very short half-life, their in vivo presence is extremely transient. Therefore, to understand the consequences of the presence of excessive amounts of ROS, one has to study the downstream effects of excessive ROS, called footprints of increased oxidative stress. Wolff and Dean (1987) introduced the concept of the Maillard reaction to describe the role of reducing sugars as catalysts in the oxidative modification and cross-linking of proteins, defined as reactive carbonyls (RCO): autoxidative glycosylation leading to production of reactive dicarbonyls such as methylglyoxal, 3-deoxyglucosone, and glyoxal. These Schiff bases undergo spontaneous rearrangements (Amadori products) resulting in the formation of advanced glycation end products (AGEs) (40).
ADVANCED GLYCATION END PRODUCTS
Aging and hyperglycemia are associated with increased production of ROS resulting in the modification of long-lived extracellular proteins such as collagen, elastin, laminin, and myelin sheath proteins. Similar phenomenon has been demonstrated in patients with CKD (41,42).
Three different carbohydrate-derived oxidation products are identified as Nε-(carboxymethyl)lysine (CML), Nε-(carboxymethyl)hydroxylysine (CMhL), and pentosidine (41). Intravenous administration of experimentally prepared AGE-modified albumin or enzymatically prepared AGE-peptides results in widespread vascular leakage, increased macrophage chemotactic activity, impaired NO-induced vasodilatation, and glomerulosclerosis (42).
Sell and Monnier (43) reported increased tissue levels of the AGE-specific moiety pentosidine in collagen tissues of patients with end-stage kidney disease (ESKD) and aging patients. Makita et al. (44), with the use of radioreceptor assays, demonstrated significantly higher levels of AGEs in medium-sized arteries of diabetic patients and nondiabetic subjects. Diabetic patients with ESKD had twice the levels of tissue AGEs as compared with diabetic patients without kidney disease. Dialysis-dependent diabetic patients had mean AGE levels five times higher than those diabetic patients without kidney disease. Although serum creatinine decreases by 75% with each dialysis session, AGE levels decreases by less than 25%. Successful kidney transplantation, however, is associated with a decrease in the plasma levels of AGEs commensurate with the decrease in creatinine, but levels of AGEs continue to remain higher than in healthy controls.
Different sizes of AGEs have been noted in the blood of patients with diabetes and uremia: those with AGEs less than 10 kDa versus greater than 10 kDa. Makita et al. (44) measured peptide-linked degradation products of AGEs, labeled as low molecular weight AGE-modified molecules (LMW-AGEs), with a molecular weight of 2,000 to 6,000. Serum levels of LMW-AGE remain five- to sixfold higher in patients undergoing dialysis therapy with cuprophane or high-flux membranes as well as in PD patients than in healthy controls (45).
The use of high-flux membranes was associated with at least a 50% decrease in LMW-AGE in both diabetic and nondiabetic dialysis patients. However, these levels returned to baseline values within first 4 hours after dialysis. In vitro experiments demonstrated that LMW-AGEs retain strong chemical activity against tissue collagen and vascular endothelium; this interaction was abrogated with the use of inhibitors of AGE-cross-linking, such as aminoguanidine (AGN). These results suggest that LMW-AGEs are refractory to removal by current modes of dialysis therapy and may be a component of the “middle molecule” uremic toxin (46).
ADVANCED LIPID PEROXIDATION PRODUCTS
Nonenzymatic oxidation of polyunsaturated fatty acids either in the plasma or cell membranes by H2O2, and HOCl results in the formation of lipid hydroperoxides and reactive aldehydes (47,48). These lipid hydroperoxides and reactive aldehydes are unstable and undergo spontaneous rearrangements or break down into different smaller and stable compounds such as malondialdehyde (MDA), 4-hydroxynonenal, glyoxal, and acrolein (49). Boaz et al. (50) found that patients with highest levels of post-HD MDA were almost four times more likely to have accelerated atherosclerosis.
Isoprostanes (8-iso-PGF2a) are generated by peroxidation of arachidonic acid of CMLs. The peroxidation occurs in situ in membrane phospholipids; these are then cleaved by endogenous phospholipases and circulate as isoprostanes. These new classes of oxidative stress by-products are strong vasoconstrictors (51). Elevated levels of urinary isoprostanes decrease with antioxidant treatment in healthy volunteers (52).
ADVANCED OXIDIZED PROTEIN PRODUCTS
ROS can alter the primary, secondary, or tertiary structure of proteins resulting initially in denaturation and fragmentation and finally cross-linking of protein moieties (11). Such cross-linked proteins are less susceptible to proteolytic digestion, resulting in long-term accumulation. Witko-Sarsat et al. (53) developed a novel spectrophotometric assay for the detection of oxidatively modified proteins called advanced oxidized protein product (AOPP). The plasma levels of AOPP were significantly increased in patients on dialysis (HD or PD) and in patients with advanced kidney disease but not on dialysis when compared with healthy controls.
The plasma levels of AOPP in dialysis patients correlated well with increased levels of methylguanidine (a marker of oxidant-induced protein cross-linking and aggregation), thiobarbituric acid reactive substances (TBARS), and pentosidine as markers of advanced lipid oxidation product (ALE) and AGE, respectively. Similarly, the levels of AOPP were closely related to soluble markers of monocyte activation such as neopterin and tumor necrosis factor alpha (TNF-α) (54).
In vitro exposure of control plasma samples and human serum albumin (HSA) to HOCl (a strong oxidizing agent) results in the dose-dependent formation of AOPP. In vitro exposure of HSA-AOPP aggregate is capable of triggering the respiratory burst of isolated neutrophils in a dose-dependent manner. In view of these findings, it is postulated that neutrophils generate HOCl leading to the formation of AOPP, and these molecules will in turn stimulate phagocytes and release more HOCl (53). The molecular mechanisms that allow AOPP to stimulate monocytes may be dependent on ligand–receptor type interaction. These in vitro experiments demonstrate that AOPPs per se can perpetuate oxidative stress (54).
THE EUROPEAN UREMIC TOXIN WORK GROUP
Owing to recent advances in proteomics and metabolomics and a reanalysis of uremic solutes, “The European Uremic Toxin (EUTox) Work Group” defined more than 90 compounds that were classified as uremic toxins. These toxins have different molecular weights and have been distributed in three different groups based on their molecular weights; of these 96 toxins, 68 are smaller than 500 Da (small molecules), another 10 toxins are between 500 and 12,000 Da (middle molecules), and the remaining exceed 12,000 Da (large molecules). More than 25% of these toxins are protein-bound (46). This definition was later revised to classify uremic molecules into two categories: protein-bound solutes and middle molecules. For the latter group, the Work Group proposed that middle molecules comprise a group of proteins with a molecular weight (500 to 60,000 Da), which incorporates many toxins identified since the original middle molecule hypothesis, for which the upper molecular weight limit was approximately 2,000 Da. The low molecular weight peptides and proteins (LMWPs) comprise nearly the entire middle molecule category in the new scheme (55,56). Most of the toxins generated under the influence of increased oxidative stress fall in the category of middle molecules and are not amenable to removal by the routinely available dialysis techniques. In addition, the EUTox group demonstrated that kinetics of urea removal do not correlate with clearance of the middle and the large molecules, hence leading to the accumulation of these molecules that could potentially perpetuate the oxidative stress (46,57).
As middle molecules are modified by the ROS and reactive nitrogen species (RNS), such modifications affect their mass transfer (filterability), molecular weight, and net charge. These physiochemical changes impede their removal by the conventional dialysis methods (55–57). This concept is important to understand so that effective strategies for the removal of these factors can be developed in the future to prevent the progression in atherosclerosis and inflammation in dialysis patients.
ADVANCED NUCLEIC ACID PEROXIDATION PRODUCTS
The interaction of OH− with nucleobases of DNA strand, such as guanine, leads to the formation of C8-hydroxyguainine or its nucleoside form named 8-hydroxy 28-deoxyguanosine (8-OH-dG). 8-OH-dG undergoes keto-reaction and results in the formation of oxidized product leading to the formation of 8-oxo-dG. Both 8-OH-dG and 8-oxo-dG are important biomarkers of free radical–induced oxidative DNA damage. Increased levels of these compounds have been demonstrated in HD as well as continuous ambulatory peritoneal dialysis (CAPD) patients (58,59). Electron spin resonance spectroscopy can be used to measure plasma oxidant activity. Roselaar et al. (60) used this technique to measure 8-OH-dG in CKD patients, and these levels did not change with dialysis therapy. Similarly, increased levels of 8-OH-dG levels in peripheral mononuclear cells have been demonstrated in dialysis patients compared to healthy controls (59).
CONSEQUENCES OF INCREASED OXIDATIVE STRESS
Increased markers of oxidant stress (especially in patients with diabetes mellitus) correlate with the severity of diabetic complications. In patients with kidney disease, oxidant stress-related processes are more diverse and intense, with an attendant alteration of a variety of cellular components irrespective of the level of blood glucose. These oxidized products play a prominent role in a variety of complications and may contribute a significant degree of morbidity and mortality in dialysis patients (FIGURES 19.4 and 19.5).
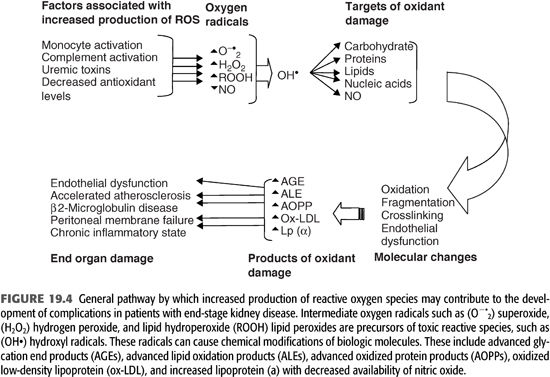
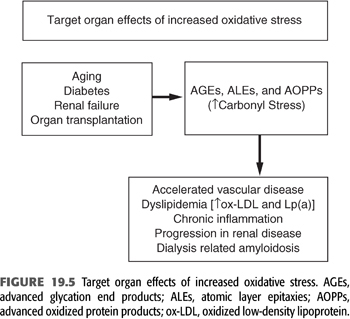
Role of Low-Density Lipoprotein Oxidation
Perhaps the most common modification is the oxidation of LDL, resulting in the formation of ox-LDL. As LDL becomes oxidized, the lipoprotein loses its ability to be recognized by the LDL receptor, and it becomes easily available to be phagocytosed by other scavenger cells such as macrophages. The macrophages become lipid loaded with decreased degradation and decreased mobility, and these changes result in the conversion of such macrophages into foam cells. The accumulation of foam cells in the intima of blood vessels is an initial step in the development of the fatty streak of the atherosclerotic lesion. Steinberg and others demonstrated the role of the early fatty streak (the precursor lesion) that subsequently leads to the development of an intermediate lesion and finally the complicated lesion of atherosclerotic plaques leading to the development of plaque rupture and acute cardiovascular event (61,62).
Increased levels of autoantibodies against the epitopes of ox-LDL and MDA-LDL have been demonstrated in the atherosclerotic plaques of coronary arteries and in the blood of dialysis patients with and without coronary artery disease. Several longitudinal studies in subjects with normal renal function have shown that the titers of circulating antibodies against ox-LDL are independent cardiovascular risk factors for progression in atherosclerosis (63). Maggi et al. (64) showed that LDL from patients with uremia and those dependent on dialysis is easily susceptible to in vitro oxidation. Increased ratio of plasma antioxidized LDL antibodies to anti-native LDL was present in patients with advanced uremia and in patients on maintenance dialysis compared with healthy controls (FIGURE 19.5).
Ox-LDL is generated by a number of pathways, including the MPO–hydrogen peroxide–nitrite (MPO–H2O2–NO2–) system (65). MPO is activated during each dialysis session due to the exposure of blood with extracorporeal circulation and other dialysis-related factors. This pathway is particularly relevant in dialysis patients (FIGURE 19.6). During the oxidation process of LDL, there is a concomitant generation of atherogenic oxidized choline glycerophospholipids (oxPCCD36) which reacts with its ligand called CD36 and had been demonstrated in vivo at sites of increased oxidative stress (66). CD36 is expressed on macrophages and endothelial cells. It functions as a signaling molecule and stimulates macrophage foam cell formation by uptake of ox-LDL. Recent evidence has shown that CD36 is also expressed on platelets. Interaction of oxPCCD36 with platelet CD36 increases platelet reactivity and facilitates prothrombotic phenotype (66). Therefore, it establishes a strong link between oxidant stress, dyslipidemia, and prothrombotic events in the vascular system.
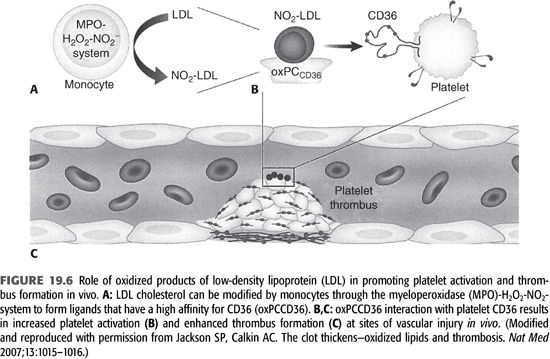
Role of Urea Oxidation
Carbamylation of amino acids and proteins can develop in dialysis patients due to spontaneous generation of cyanate from urea (67). The active form of cyanate (OCN−) is isocyanic acid that carbamylates amino acids, proteins, and other molecules. The carbamylation process changes their structure, charge, as well as function. In addition, carbamylated molecules can inhibit or potentiate other noncarbamylated proteins, producing various metabolic derangements including the production of reactive oxidant molecule, ε-N-C-lysine (homocitrulline) (67) (FIGURE 19.7). Using monoclonal antibodies, ε-N-C-lysine (homocitrulline) was demonstrated in neutrophils, monocytes, and more importantly, conjugated with low-density lipoprotein (called carbamylated-LDL); this transformation enhances the atherogenicity of LDL (68) (FIGURE 19.8).
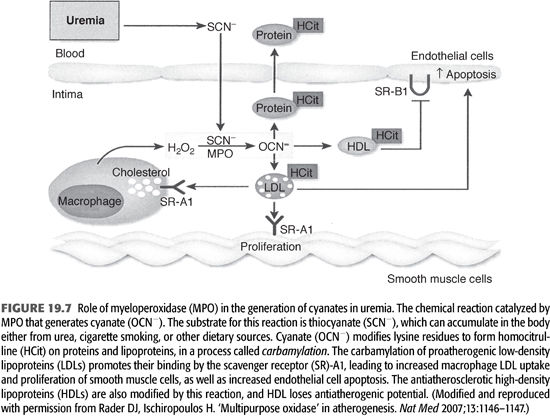
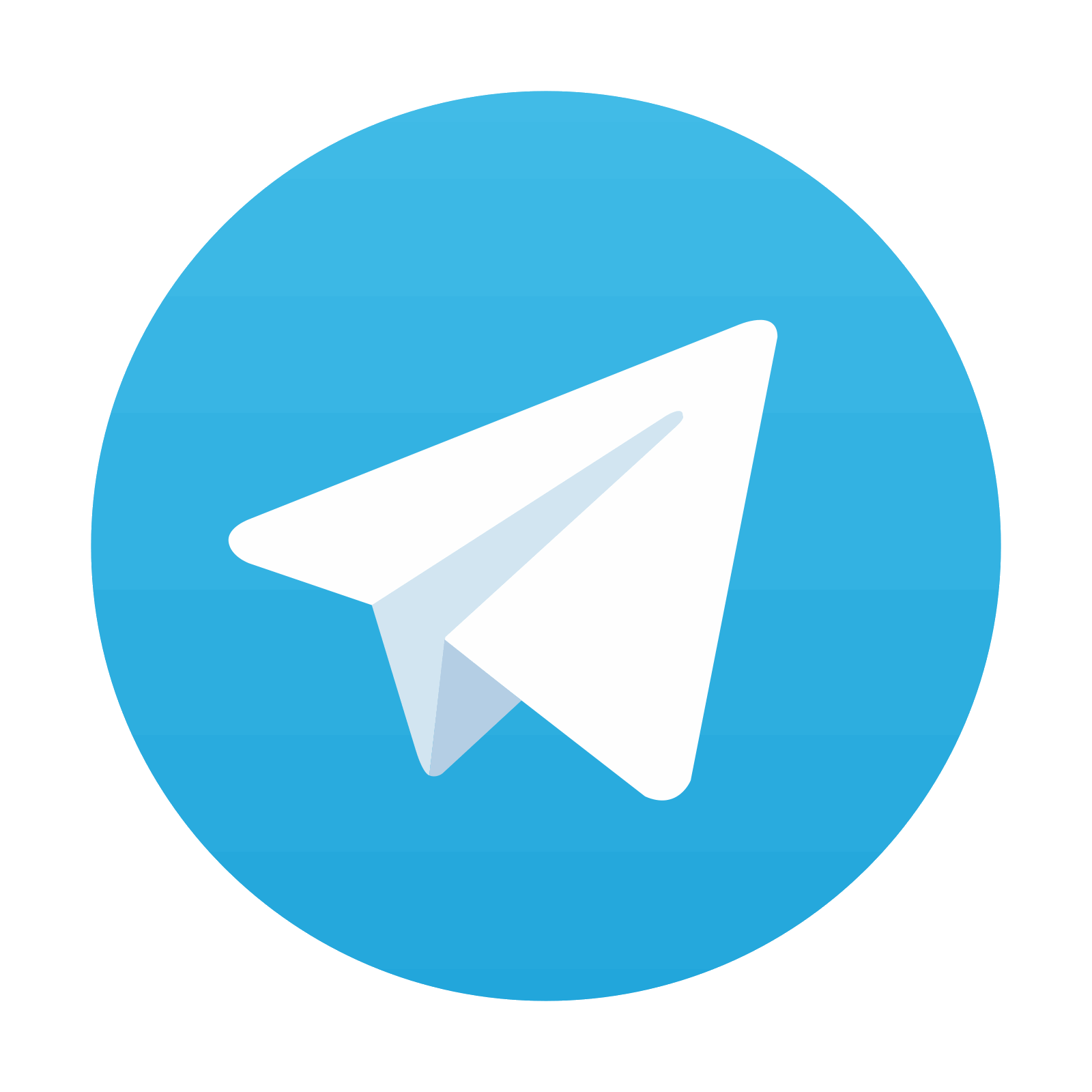
Stay updated, free articles. Join our Telegram channel
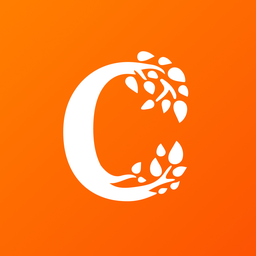
Full access? Get Clinical Tree
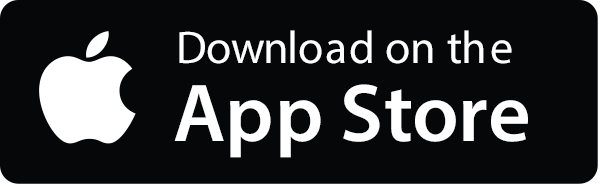
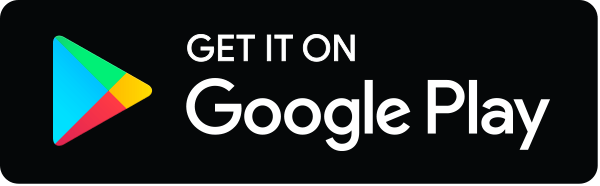