Paul J. Turek, MD, FACS, FRSM
Hypothalamic-Pituitary-Gonadal Axis
Basic Endocrine Concepts
Two classes of hormones mediate communication in the reproductive axis: peptide and steroid. Peptide hormones are small, secretory proteins that act through cell surface receptors. Hormone signals are transduced by one of several second-messenger pathways (Fig. 20–1). Ultimately, most peptide hormones induce phosphorylation of proteins that alter cell function. Examples of peptide hormones are luteinizing hormone (LH) and follicle-stimulating hormone (FSH). In contrast, steroid hormones are derived from cholesterol. They are not stored in secretory granules; consequently, steroid secretion rates directly reflect rates of production. In plasma, these hormones are usually bound to carrier proteins, and because they are lipophilic, steroid hormones are cell membrane–permeable. After binding to intracellular receptors, steroids are translocated to nuclear DNA recognition sites and regulate the transcription of target genes. Examples of reproductive axis steroid hormones are testosterone and estradiol.
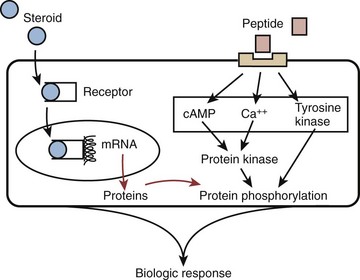
(Adapted from Turek PJ. Male infertility. In: Tanagho EA, McAninch JC, editors. Smith’s urology. 16th ed. Stamford, CT: Appleton & Lange; 2008 [chapter 44–1].)
Hormonal signaling within the HPG axis is hierarchically governed by a free-running pulse generator within the hypothalamus. The specific amplitude and frequency with which hormone secretions occur within the reproductive axis determine downstream organ responsiveness. Feedback control is the principal mechanism through which hormonal regulation occurs in the HPG axis (Fig. 20–2). With feedback control, a hormone can regulate the synthesis and action of itself or of another hormone. In the HPG axis, negative feedback activity is primarily responsible for minimizing hormonal perturbations and maintaining homeostasis.
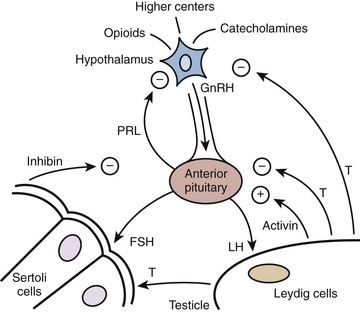
(Adapted from Turek PJ. Male infertility. In: Tanagho EA, McAninch JC, editors. Smith’s urology. 16th ed. Stamford, CT: Appleton & Lange; 2008 [chapter 44–2].)
Components of the Reproductive Axis
Hypothalamus
As the integrative center of the HPG axis, the hypothalamus receives neuronal input from the amygdala, thalamus, pons, retina, olfactory cortex, and many other areas (see Fig. 20–2). The pulse generator for the cyclical secretion of pituitary hormones, the hypothalalmus, is anatomically linked to the pituitary gland by both a portal vascular system and neuronal pathways. By avoiding the systemic circulation, the portal vascular system allows direct delivery of hypothalamic hormones to the anterior pituitary.
The most important hypothalamic hormone for reproduction is gonadotropin-releasing or LH-releasing hormone (GnRH or LHRH), a 10–amino acid peptide secreted from the neuronal cell bodies in the preoptic and arcuate nuclei. Currently, the only known function of GnRH is to stimulate the secretion of luteinizing hormone (LH) and follicle-stimulating hormones (FSH) from the anterior pituitary. GnRH has a plasma half-life of approximately 5 to 7 minutes, and is almost entirely removed on the first pass through the pituitary either by receptor internalization or enzymatic degradation. GnRH secretion results from integrated input from the effects of stress, exercise, and diet from higher brain centers, gonadotropins secreted from the pituitary, and circulating gonadal hormones. Substances known to regulate GnRH secretion are listed in Table 20–1. In Kallman syndrome, characterized by congenital hypogonadotropic hypogonadism, the GnRH precursor neurons fail to migrate normally with a subsequent absence of hypothalamic secretion of GnRH (Bick et al, 1992; Dode et al, 2003). Affected individuals present with delayed puberty or infertility due to lack of testosterone production.
Table 20–1 Substances That Modulate GnRH Secretion
GnRH MODULATOR | TYPE OF FEEDBACK | EXAMPLES |
---|---|---|
Opioids | Negative | β-Endorphin |
Catecholamines | Variable | Dopamine |
Peptide hormones | Negative | FSH, LH |
Sex steroids | Negative | Testosterone |
Prostaglandins | Positive | PGE2 |
Insulin | Positive | Insulin |
Kisspeptins | Positive | Kisspeptin (puberty) |
Leptins | Positive | Leptin |
FSH, follicle-stimulating hormone; LH, luteinizing hormone; PGE2, prostaglandin E2.
Anterior Pituitary
Located within the bony sella turcica of the cranium, the pituitary has two lobes: posterior and anterior. The posterior lobe, or neurohypophysis, secrets two hormones, oxytocin and vasopressin, and is driven by neural stimuli. In contrast, the anterior pituitary or adenohypophysis is regulated by blood-borne factors and is the site of action of GnRH (see Fig. 20–2). GnRH stimulates the production and release of FSH and LH by a calcium flux–dependent mechanism. The sensitivity of the pituitary gonadotrophs for GnRH varies with patient age and hormonal status. LH and FSH are the primary pituitary hormones that regulate testis function. They are glycoproteins composed of two polypeptide chain subunits, termed α and β, each coded by a separate gene. The α subunit of each hormone is identical and is similar to that of all other pituitary hormones; biologic and immunologic activities are conferred by the unique β subunit. Both subunits are required for endocrine activity. Sugars linked to these peptide subunits, consisting of oligosaccharides with sialic acid residues, differ in content between FSH and LH and likely account for differences in plasma clearance of these hormones. Secretory pulses of LH vary in frequency from 8 to 16 pulses in 24 hours and vary in amplitude by one- to threefold. These pulse patterns closely reflect GnRH release. Both androgens and estrogens regulate LH secretion through negative feedback. On average, FSH pulses occur approximately every 1.5 hours and vary in amplitude by 25%. The FSH response to GnRH is more difficult to measure than that of LH for two reasons: (1) FSH has a smaller amplitude response and a longer serum half-life, (2) the gonadal proteins inhibin and activin may affect FSH secretion and are thought to account for the relative secretory independence of FSH from GnRH secretion.
A third anterior pituitary hormone, prolactin, can also affect the HPG axis and fertility. Prolactin is a large, globular protein of 199 amino acids (23 kD) that is responsible for milk synthesis during pregnancy and lactation in women. No human mutations have been found in either the human prolactin gene or its receptor (Goffin et al, 2002). The normal role of prolactin in men is less clear, but it may increase the concentration of LH receptors on Leydig cells and sustain normal, high intratesticular testosterone levels. It may also potentiate the effects of androgens on the growth and secretions of the male accessory sex glands (Wennbo et al, 1997; Steger et al, 1998). Normal prolactin levels may be important to maintain libido. Although low prolactin levels are not necessarily pathologic, hyperprolactinemia abolishes gonadotropin pulsatility by interfering with episodic GnRH release. In addition, the anterior pituitary contains cells that secrete other glycoprotein hormones: adrenocorticotropic hormone (ACTH), growth hormone (GH), and thyroid-stimulating hormone (TSH). These glycoprotein hormones can also have significant effects on male reproductive function.
Testis
Normal male virility and fertility requires the collaboration of the exocrine and endocrine testis (see Fig. 20–2). The interstitial compartment, composed mainly of Leydig cells, is responsible for steroidogenesis. The seminiferous tubules produce spermatozoa.
The primary site of FSH action is on Sertoli cells within seminiferous tubules. In response to FSH, Sertoli cells produce androgen-binding protein, transferrin, lactate, ceruloplasmin, clusterin, plasminogen activator, prostaglandins, and growth factors. Through these FSH-mediated factors, seminiferous tubule growth is stimulated during development, and sperm production is initiated during puberty. Interestingly, mice FSH knockout studies suggest that FSH is not essential for spermatogenesis, because affected mice can be fertile (Levallet et al, 1999). In humans, it is thought that FSH is required for normal spermatogenesis (Tapanainen et al, 1997).
The testis also produces the protein hormones inhibin and activin (Itman et al, 2006). Inhibin is a 32-kD protein made by Sertoli cells that inhibits FSH release from the pituitary. Within the testis, inhibin production is stimulated by FSH and acts by negative feedback at the pituitary or hypothalamus. Activin, a testis protein with close structural homology to transforming growth factor–β, exerts a stimulatory effect on FSH secretion. Activin receptors are found in a host of extragonadal tissues, suggesting that this hormone may have growth factor or regulatory roles in the body.
Negative feedback suppression of GnRH release by testosterone occurs through androgen receptors (AR) present in hypothalamic neurons and in the pituitary. In studies of genetic mutations, it is clear that both testosterone and estrogen participate in negative feedback (Shupnik and Schreihofer, 1997). Steroid negative feedback results mainly from AR binding to testosterone, with a smaller contribution from estradiol binding. Testosterone feedback occurs mainly at the hypothalamus, whereas estrogens feedback to the pituitary (Santen, 1975). It also appears that although testosterone is the primary regulator of LH secretion, estradiol (along with inhibin from Sertoli cells) is the predominant regulator of FSH secretion (Hayes et al, 2001).
Development of the HPG Axis
Sex determination is genetically determined in humans. A critical gene for sex determination is SRY (sex-determining region Y gene) on the short arm of the Y chromosome. The SRY gene product is a protein with a high mobility group box (HMG) sequence, a highly conserved DNA-binding motif that kinks DNA. This DNA bending effect alters gene expression, leading to testis formation and subsequently to the male phenotype. However, the SRY gene does not act in isolation to determine human sex. DAX1, a nuclear hormone receptor gene, can alter SRY activity during development by suppressing genes downstream to SRY that would normally induce testis differentiation. A second gene, WNT4, largely confined to the adult ovary, may also serve as an “antitestis” gene. The discovery of these genes has significantly altered theories of sex determination. In the past, the female genotype was considered the “default,” SRY-negative, developmental pathway. It is now clear that genes such as WNT4 and DAX1 can proactively induce female gonadal development, even in the presence of SRY (DiNapoli and Capel, 2008).
Once gonadal sex is determined, Leydig cells make testosterone that induces development of the internal genitalia (Fig. 20–3). Leydig cells also synthesize insulin-like-3 to promote transabdominal testis migration into the scrotum. DHT masculinizes the genital anlage to form the external genitalia (see Fig. 20–3). In addition, Sertoli cells within the developing testis synthesize müllerian-inhibiting substance (MIS, AMH) that prevents the müllerian duct from developing into uterus and fallopian tubes and keeps the early germ cells quiescent in the testis (Fig. 20–4). Deficiencies in these developmental pathways generally result in either birth defects or intersex disorders.
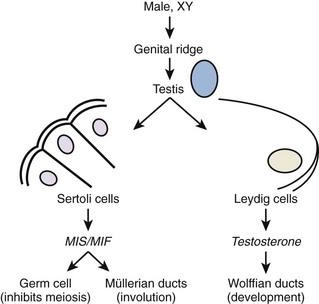
Figure 20–4 Early differentiation pathway of the male. MIF/MIS, müllerian inhibiting factor/substance.
(Adapted from Turek PJ: Male infertility. In: Tanagho EA, McAninch JC, editors. Smith’s urology. 16th ed. Stamford, CT: Appleton & Lange; 2008 [chapter 44–13].)
The hormonal feedback relationships within the HPG axis also become established during gestation. The expression of kisspeptin protein is in part responsible for activating GnRH neurons and triggering GnRH release. In addition, SF-1, an orphan nuclear receptor, is secreted by developing Sertoli cells and contributes to HPG axis development (Val et al, 2003). After the withdrawal of placental steroids at birth, there is a period of high gonadotropin secretion in the neonate. Subsequently, as axis sensitivity to gonadotropins increases, FSH and LH secretions fall to the low levels characteristic of childhood. Puberty begins with GnRH pulsing, leading gonadotropins to increase to adult levels and, subsequently, to increase sex hormones. The hypothalamic capacity to generate GnRH pulses arises at puberty, usually starting around the 12th year. Puberty begins at critical growth, weight, and nutritional rates for boys and girls, and is likely initiated by kisspeptin, melatonin, and leptin (Clement et al, 1998). The adipocyte hormone leptin is the body’s regulatory signal governing the size of the fat stores, and there is increasing evidence that leptin modulates hypothalamic and pituitary activity (Caprio et al, 1999; Kiess et al, 1999; Quinton et al, 1999).
Aging and the HPG Axis
A progressive decline in testosterone and sperm production occurs with age, such that men in the 7th decade have mean plasma testosterone levels 35% lower than young men (Vermeulen et al, 1995). The consequence of this is a phenomenon has been variously termed male menopause, male climacteric, andropause, or, more appropriately, partial androgen deficiency in the aging male (PADAM). The changes to the seminiferous epithelium with age include decreases in seminiferous tubule volume and length. An age-related decrease in sperm production in older testes appears to stem from a decrease in germ cell proliferation rather than cellular degeneration. Correspondingly, FSH levels also increase with age, with mean values threefold higher in older than younger men. The etiology of the age-related decline in HPG axis function is multifactorial. Testosterone production is reduced because of decreased numbers of Leydig cells and an increase in testosterone binding proteins. Diurnal variation of testosterone secretion is also lost in elderly men. There is also evidence for a blunted HPG feedback response to low testosterone (despite generally high levels of gonadotropins) and to GnRH stimulation. Finally, normal pulsatile GnRH release is replaced by irregular pulses that are less effective in stimulating gonadotropin release (Mulligan et al, 1997). A combination of these effects is likely responsible for diminished HPG axis function with age in men.
The Testis
Gross Architecture
The testis is a white, ovoid organ that is normally 15 to 25 mL in volume (Prader, 1966) and has a length of 4.5 to 5.1 cm (Tishler, 1971; Winter and Faiman, 1972). The tunica albuginea has smooth muscle cells that course through predominantly collagenous tissue (Langford and Heller, 1973). These smooth muscle cells may impart contractile capability to the capsule (Rikmaru and Shirai, 1972), may affect blood flow into the testis (Schweitzer, 1929), and promote the flow of seminiferous tubule fluid out of the testis (Davis and Horowitz, 1978).
The testis parenchyma is divided into compartments separated by septa. Each septum divides seminiferous tubules into lobes that each contain a centrifugal artery. Individual seminiferous tubules harbor developing germ cells and interstitial tissue. Interstitial tissue is composed of Leydig cells, mast cells, macrophages, nerves, and blood and lymph vessels. In humans, interstitial tissue comprises 20% to 30% of total testicular volume (Setchell and Brooks, 1988). The relationship between seminiferous tubules and interstitial tissue anatomy is demonstrated in Fig. 20–5. Seminiferous tubules are long, highly coiled, and looped. Both ends usually terminate in the rete testis. It is estimated that the combined length of the 600 to 1200 tubules in the human testis is approximately 250 meters (Lennox and Ahmad, 1970) (Fig. 20–6). The “hub” of the testis, also termed the rete testis, coalesces to form 6 to 12 ductuli efferentes that carry testicular fluid and spermatozoa into the caput epididymis (see Fig. 20–6).
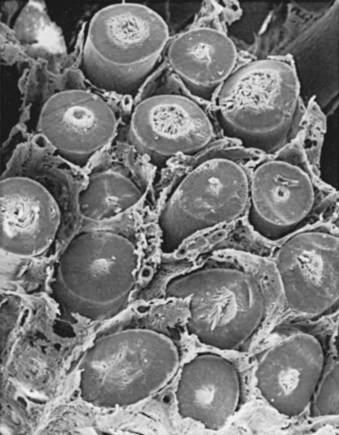
(From Christensen AK. Leydig cells. In: Greep RO, Astwood EB, editors. Handbook of physiology. Washington, DC: American Physiology Society; 1975. p. 57–94.)
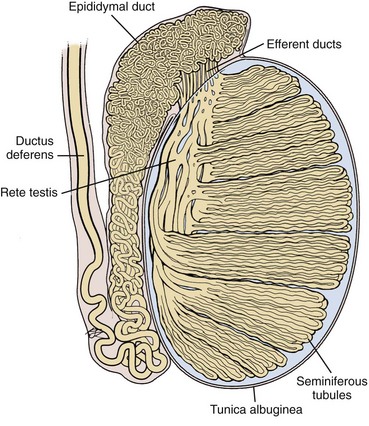
(Based on Hirsh AV. The anatomical preparations of the human testis and epididymis in the Glasgow Hunterian Collection. Hum Reprod Update 1995;1:515–21.)
The arterial supply to the testis and epididymis is derived from three sources: the internal spermatic artery, the deferential (vasal) artery, and the external spermatic (or cremasteric) artery (Harrison and Barclay, 1948). The internal spermatic artery arises from the abdominal aorta and is intimately associated with the pampiniform plexus of veins. The vascular arrangement within the pampiniform plexus, with the counterflowing artery and veins, facilitates the exchange of heat and small molecules. For example, testosterone passively diffuses from veins to the artery in a concentration-limited manner (Bayard et al, 1975). The countercurrent heat exchange supplies arterial blood to the testis that is 2° C to 4° C lower than the rectal temperature in normal men (Agger, 1971). A loss of the temperature differential is associated with testicular dysfunction in men with varicocele (Goldstein and Eid, 1989) and cryptorchidism (Marshall and Edler, 1982). As the spermatic cord is commonly dissected during varicocele repair, it is surgically relevant to know that a single artery is observed in 50% of spermatic cords, with two arteries in 30% and three arteries in 20% of cases (Beck et al, 1992).
Inferior to the scrotal pampiniform plexus and near the mediastinal testis, the spermatic artery is highly coiled and branches before entering the testis. Extensive interconnections, especially between the internal spermatic and deferential arteries, allow maintenance of testis viability even after division of the internal spermatic artery (Fig. 20–7). From angiographic studies, a single artery enters the testis in 56% of cases; two branches enter in 31% of cases and three or more branches in 13% of testes (Kormano and Suoranta, 1971). In men with a single testicular artery, its interruption can result in testicular atrophy (Silber, 1979). The testicular arteries penetrate the tunica albuginea and then travel inferiorly along the posterior surface of the testis within the parenchyma. Branching arteries pass anteriorly over the testicular parenchyma. Major testicular artery branches also travel over the inferior pole of the testis, pass anteriorly and branch out over the surface of the testis. The location of these vessels is clinically important, because they may be injured during orchiopexy or testis biopsy procedures (Jarow, 1991; Schlegel and Su, 1997). The midsection of the testis has relatively fewer vessels compared with superior or inferior areas of the testis. Individual arteries to the seminiferous tubules, termed centrifugal arteries, travel within the septa that contain tubules. Centrifugal artery branches give rise to arterioles that supply individual intertubular and peritubular capillaries (Muller, 1957). The intertubular capillaries are located within the columns of interstitial tissue, whereas the ladder-like capillaries running near the seminiferous tubule are called peritubular capillaries. Through this vascular complex, the human testis is provided with 9 mL of blood per 100 g of tissue per minute (Pettersson et al, 1973).
Veins within the testis are unusual in that they do not run with the corresponding intratesticular arteries. Small parenchymal veins empty into either the veins on the testis surface, or into a group of veins near the mediastinum testis that travels along the rete testis (Setchell and Brooks, 1988). These two sets of veins join together with deferential veins to form the pampiniform plexus as they ascend into the scrotum. Pampiniform plexus veins are thin walled, which likely contributes to the passive diffusion of testosterone and temperature exchange with the closely associated spermatic artery.
The testis has no known somatic innervation. It receives autonomic innervation primarily from the intermesenteric nerves and renal plexus (Mitchell, 1935). These nerves run along the testicular artery into the testis. It appears that testicular adrenergic innervation is restricted primarily to small blood vessels that supply Leydig cell clusters that may regulate Leydig cell steroidogenesis (Baumgarten et al, 1968; Turnbull and Rivier, 1997). It is thought that vascular tone in the testis may involve regulation at several levels (Linzell and Setchell, 1969), including autoregulation of capsular arteries (Davis et al, 1990), regional variation based on local metabolic need and governed by peptides such as atrial natriuretic peptide (Collin et al, 1997), and assisted transport of molecules such as LH across the vascular endothelium (Milgrom et al, 1997). Indeed, these observations suggest a highly specialized function for the microvasculature of the testis (see review by Desjardins [1989]).
Prominent lymphatics can be observed within the spermatic cord (Hundeiker, 1971). Obstruction of these ducts results in dilatation of the testis interstitium but not the seminiferous tubules, suggesting that the interstitial space is drained by lymphatics, but the seminiferous tubules are not. Lymphatic obstruction can also result in hydrocele formation, a known complication of certain varicocelectomy and herniorrhaphy procedures. The sperm containing intratubular fluid that baths Sertoli cells flows from the seminiferous tubules into the rete testis and, subsequently, into the caput epididymis. This fluid, isosmotic with plasma, is thought to be mainly of seminiferous tubule origin (Setchell and Brooks, 1988). Reabsorption of this fluid within the rete testis and efferent ductules is regulated by estrogens (Lee et al, 2000). Tubular fluid composition is markedly different from blood plasma or lymphatics, suggesting that substances are not freely diffusible into and out of the tubules (Setchell and Waites, 1975). This has led to the concept of a “blood-testis barrier” to be discussed later.
Testis Cytoarchitecture
Interstitium
Leydig Cells
The testis interstitium contains blood vessels, lymphatics, fibroblasts, macrophages, mast cells, and Leydig cells (Fig. 20–8). Leydig cells are responsible for the bulk of testicular steroid production. Leydig cells differentiate from mesenchymal precursor cells by the 7th week of gestation. The activation of Leydig cell steroidogenesis correlates with the onset of androgen-dependent differentiation of the male reproductive system. Although it is apparent that Leydig cells express steroidogenic enzymes before becoming responsive to LH (El-Gehani et al, 1998; Majdic et al, 1998), they also differentiate from precursor cells under the influence of LH, placental-derived human chorionic gonadotropin (HCG), and from the effect of local paracrine factors such as IGF-1 (Huhtaniemi and Pelliniemi, 1992; Teerds and Dorrington, 1993; Le Roy et al, 1999). At 2 to 3 months after birth, a second wave of Leydig cell differentiation occurs in response to gonadotropin production from the pituitary, briefly elevating testosterone levels in infants. Androgen produced during the the early male neonate’s life is thought to hormonally imprint the hypothalamus, liver, and prostate such that they respond appropriately to androgen stimulation later in life. After reactivation of the HPG axis at puberty, stereologic analysis has revealed that a single testis from a young adult contains approximately 700 million Leydig cells (Kaler and Neaves, 1978).
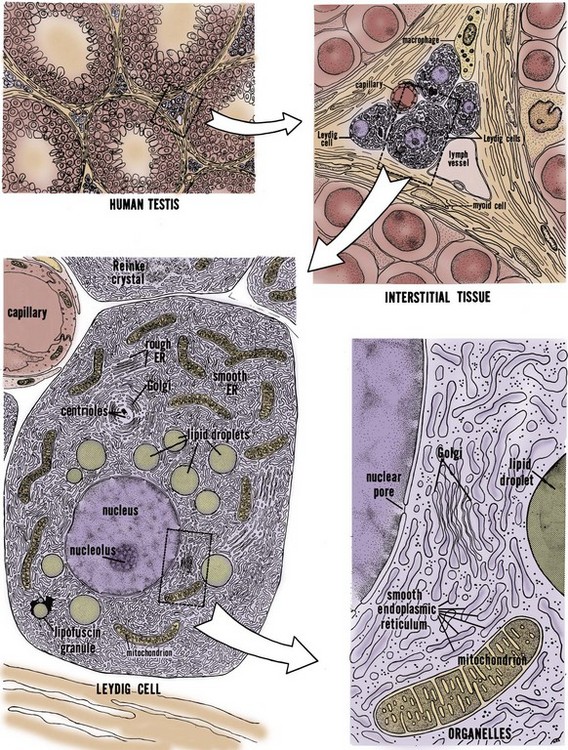
(From Christensen AK. Leydig cells. In: Greep RO, Astwood WB, editor. Handbook of physiology, section 7, Endocrinology. Baltimore: Williams & Wilkins; 1975.
Testosterone
Testosterone, synthesized from cholesterol, is the principal steroid produced by the testis (Lipsett, 1974). In addition, numerous C18, C19, and C21 steroids are also produced (Lipsett, 1974; Ewing and Brown, 1977). Cholesterol must be transported into Leydig cell mitochondria, where the cholesterol side-chain cleavage enzyme converts it to pregnenolone. The three main sources of cholesterol in the Leydig cell are (1) externally, from blood-borne lipoprotein and internalization of cholesterol-lipoprotein receptor complexes, (2) from de novo synthesis from acetate, and (3) from stored cholesterol esters in lipid droplets. Maintenance of cholesterol stores is part of the normal resting function of the Leydig cell; LH stimulation evokes cholesterol mobilization through cholesterol esterase activity. Pregnenolone is transported out of the mitochondrial membrane into the smooth endoplasmic reticulum, where it is converted into testosterone. Testosterone diffuses across the cell membrane and is trapped within the extracellular fluid and blood plasma by steroid-binding proteins.
Cholesterol transport to the inner membrane of the mitochondrion is regulated by two transport proteins: steroid acute regulatory protein (StAR) and peripheral benzodiazepine receptor (PBR). LH binding elicits protein synthesis in the Leydig cell, and the newly-synthesized StAR contains a signal sequence that enables it to be threaded through the outer mitochondrial membrane to faciliate cholesterol transport (Stocco, 2000). PBR forms a channel for cholesterol in the mitochondrial membrane (Culty et al, 1999), but it is not clear whether PBR functionally interacts with StAR (West et al, 2001).
The four major enzymes participating in testosterone biosynthesis from pregnenolone are cholesterol side-chain cleavage enzyme, 3β-hydroxysteroid dehydrogenase, cytochrome P450 17α-hydroxylase/C17-20-lyase, and 17β-hydroxysteroid dehydrogenase. The enzymology, chromosomal locations, and molecular genetics of these enzymes are well described (Payne and Hales, 2004). Gene mutations in the genes encoding these enzymes have been described and the resulting disorders of androgen biosynthesis are a relatively rare cause of sexual ambiguity in chromosomally normal males (Miller, 2002).
Control of Testosterone Synthesis
The control of Leydig cell steroidogenesis is complex and involves both pituitary and non-pituitary factors (Payne and Youngblood, 1995). The most important regulator of testosterone production is LH. LH binding, through the second messenger cyclic adenosine monophosphate (cAMP), initiates transport of cholesterol into mitochondria. Pituitary peptides other than LH (e.g., FSH and prolactin) modify the response to LH (Ewing, 1983). Other, nonpituitary factors capable of modifying steroid production by Leydig cells include GnRH (Sharpe, 1984), inhibin and activin (Bardin et al, 1989), epidermal growth factor (EGF), IGF-1, and transforming growth factor-β (TGF-β) (Ascoli and Segaloff, 1989; Saez et al, 1991), prostaglandins (Eik-Nes, 1975), and adrenergic stimulation (Eik-Nes, 1975). Moreover, direct inhibition of Leydig cell steroidogenesis may also occur through estrogens and androgens (Ewing, 1983; Darney et al, 1996).
Testosterone Cycles
Testosterone blood levels change dramatically during human fetal, neonatal, and adult life. Fig. 20–9 shows that a testosterone peak occurs in the human fetus between 12 and 18 weeks of gestation. Another testosterone peak occurs at approximately 2 months of age. A third testosterone peak occurs during the 2nd or 3rd decade of life. After this, there is a plateau, and then a slow decline with age. Superimposed on this, there are annual and daily rhythms of testosterone production (see Fig. 20–9, insets A and B) and irregular daily fluctuations in testosterone (see Fig. 20–9, inset C). These temporal changes in testosterone production during human life reflect a complex interaction between the pituitary gland and the testis. The testosterone peaks correspond temporally to the following developmental events: (1) the differentiation and development of the fetal reproductive tract, (2) the neonatal organization or “imprinting” of androgen-dependent target tissues, (3) the masculinization of the male at puberty, and (4) the maintenance of growth and function of androgen-dependent organs in the adult. This topic has been reviewed thoroughly by Swerdloff and Heber (1981).
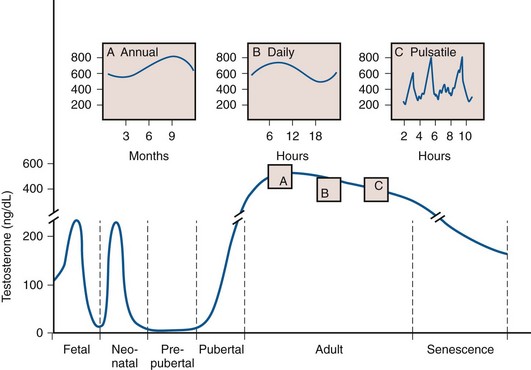
(From Ewing LL, Davis JC, Zirkin BR. Regulation of testicular function: a spatial and temporal view. In: Greep RO, editor. International review of physiology. Baltimore: University Park Press; 1980. p. 41.)
Seminiferous Tubules
Sertoli Cells
The seminiferous tubules are lined with Sertoli cells that rest on the tubular basement membrane and extend cytoplasmic ramifications into its lumen (Fig. 20–10). The ultrastructural features of Sertoli cells are well described (Bardin et al, 1994). They have irregularly shaped nuclei, prominent nucleoli, low mitotic index, and exhibit unique tight junctional complexes between adjacent Sertoli cells. These tight junctions are the strongest intercellular barriers in the body. They divide the seminiferous tubule space into basal (basement membrane) and adluminal (lumen) compartments (see Fig. 20–10). This anatomic arrangement forms the basis for the “blood-testis barrier” and allows spermatogenesis to occur in an immunologically privileged site. Sertoli cells serve as “nurse” cells for spermatogenesis, nourishing developing germ cells that are arranged between Sertoli cell cytoplasmic projections. The undifferentiated spermatogonia are near the basement membrane of the tubule, whereas the more advanced spermatocytes and spermatids are located near the luminal surface. Thus the Sertoli cell is a polarized epithelium in which the base approximates the plasma environment, and its apex harbors an environment unique to the seminiferous tubule (Ewing et al, 1980).
Sertoli cells nurture germ cell development by: (1) providing a specialized adluminal microenvironment, (2) supporting germ cells through gap junctions between Sertoli and germ cells, and (3) allowing migration of developing germ cells within the tubule (see Fig. 20–10). The tight junctions between Sertoli cells are constantly remodeled to allow “opening” and “closing” necessary for germ cell interaction and migration (Mruk and Cheng, 2004). Ligand-receptor complexes, such as c-kit and kit ligand, are likely involved in mediating communication between germ and Sertoli cells. Sertoli cells also participate in germ cell phagocytosis and produce and secrete fluid and important effector molecules. Androgen-binding protein (ABP) is one of earliest described Sertoli cell secretory products (Hansson and Djoseland, 1972). ABP is an intracellular carrier of androgen within the Sertoli cell. By binding testosterone, ABP maintains high levels of androgen (50-fold, as observed in serum) within the seminiferous tubules. Testosterone also plays an important role in the regulation of Sertoli cell function, including ABP production (Griswold, 1988). Inhibin is Sertoli cell–derived and plays an important regulatory role in the negative feedback loop of FSH secretion. Inhibin B is emerging as an important endocrine marker of Sertoli cell function in the male infertility evaluation.
As keepers of the immunological sanctuary in the testis, Sertoli cells maintain a germ cell microenvironment entirely distinct from that of plasma. As such, Sertoli cells secrete numerous other products including extracellular matrix components (lamin, collagen type IV, and collagen type I) and proteins such as ceruloplasmin, transferrin, glycoprotein 2, plasminogen activator, somatomedin-like substances, T proteins, H-Y antigen, clusterin, cyclic proteins, growth factors, and somatomedin (Mruk and Cheng, 2004). Steroids, such as DHT, testosterone, androstenediols, 17β-estradiol, and numerous other C21 steroids are also produced by Sertoli cells (Ewing et al, 1980; Mather et al, 1983). Although the function of many Sertoli cell– and peritubular-derived substances is unclear, further research will enlighten our understanding of how Sertoli cells orchestrate and support spermatogenesis.
Germ Cells
Within the human seminiferous tubule, germ cells give rise to approximately 123 × 106 (range: 21 to 374 × 106) spermatozoa daily (Amann and Howards, 1980). This equates to the production of about 1200 sperm per heartbeat. Within the seminiferous tubule, germ cells are arranged in a highly ordered sequence from the basement membrane to the lumen. Morphologic analysis of the various germ cells reveals at least 13 recognizable germ cell types in the human testis (Clermont, 1963; Heller and Clermont, 1964) (Fig. 20–11). Each cell type is thought to represent a different step in the spermatogenic process. Proceeding from the least to the most differentiated, based on morphologic appearance, they have been named dark type A spermatogonia (Ad); pale type A spermatogonia (Ap); type B spermatogonia (B); preleptotene (R), leptotene (L), zygotene (z), and pachytene primary spermatocytes (p); secondary spermatocytes (II); and Sa, Sb, Sc, Sd1, and Sd2 spermatids. The tight junctions maintain spermatogonia and early spermatocytes within the basal compartment and all subsequent germ cells in the adluminal compartment.
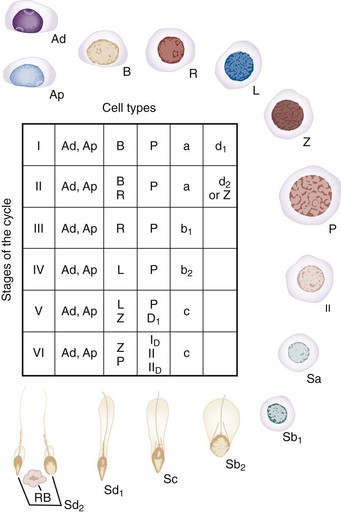
(Modified from Clermont Y. Renewal of spermatogonia in man. Am J Anat 1966;118:509.)
Peritubular Structure
The human seminiferous tubule is surrounded by several layers of peritubular tissue (Hermo et al, 1977) (Fig. 20–12). The outer adventitial layer consists of fibrocytes. In the middle layer are myoid cells interspersed with connective tissue lamellae. The inner layer consists of a collagen matrix. In humans the peritubular myoid cells are thought to have contractile function (Toyama, 1977). Myoid cells actively secrete extracellular matrix components fibronectin and collagen type I, and produce the inner collagenous layer (Tung et al, 1984). Myoid cells may also affect Sertoli cell function and are known to associate with Sertoli cells in a precise mesenchymal-epithelial interaction. Skinner and coworkers (1988) isolated a paracrine factor produced by myoid cells, P-Mod-S (peritubular modifies Sertoli), that profoundly affects Sertoli cell synthetic and differentiation functions in vitro. Human peritubular cells have also been shown to secrete testosterone, and exert regulatory Sertoli cell secretory activity (Cigorraga et al, 1994).
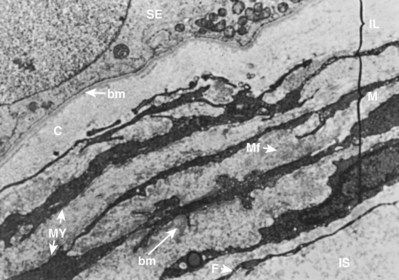
(From Hermo L, Lalli M, Clermont Y. Arrangement of connective tissue elements in the walls of seminiferous tubules of man and monkey. Am J Anat 1977;148:433–46.)
Blood Testis Barrier
Dyes and other substances, when injected into the bloodstream of animals, will rapidly appear throughout all body tissues but fail to penetrate regions of the brain and testis. From this observation, the concept of a “blood-testis barrier” was derived. More appropriately termed the “blood–seminiferous tubule barrier,” the barrier has two components: an anatomic or mechanical element and functional elements. The mechanical barrier is created, in part, by muscle-like myoid cells that surround seminiferous tubules (Dym and Fawcett, 1970; Fawcett et al, 1970). Regulation of molecular traffic also occurs at the level of capillary endothelial cells. However, the most important component of this barrier is the synaptic tight junctions between Sertoli cells that preclude the passage of large molecules and lymphocytes. These anatomical elements of the blood-testis barrier are necessary but not sufficient for maintaining the immunologic “sanctuary” status within the tubule, because they are not observed in other “protected” areas of the reproductive tract (Tung et al, 1971; Brown et al, 1972).
Thus, although the mechanical barrier contributes to the isolation of the testis, other “functional” components must also exist that suppress the normal immune response. Several mechanisms likely work in concert to protect sperm from destruction. First, lymphocytes are diverted by the vascular endothelium and are excluded from anatomically vulnerable regions in the germinal epithelium (Mahi-Brown et al, 1988). Second, these vulnerable regions harbor mainly T-suppressor cells (el-Demiry et al, 1985; Anderson and Hill, 1988). Due to deficiencies in antigen–HLA association, there may be a lack of sperm antigen presentation to lymphocytes, impairing the immune response (Jenkins et al, 1987; Anderson and Hill, 1988). There is also evidence to suggest that immunologic tolerance plays a role in the functional blood-testis barrier. Tung and colleagues have proposed that within the anatomically weaker areas (rete testis, efferent tubule, epididymis), there is a small, continuous leak of sperm antigens (Tung, 1980). This leak generates T-suppressor cells and immune tolerance, similar to desensitization protocols for common environmental allergens. However, with larger antigenic challenges, a true immune response results (Turek, 1997). Cytokines may contribute to immune tolerance, including interferon gamma, soluble Fc receptor, and transforming growth factor–β (Perussia et al, 1987; Ben Rafael, 1992; Turek, 1997). In addition, androgens have mild immunosuppressive activity and also appear to help regulate immunity (Diemer et al, 2003).
Why does the blood-testis barrier exist? Since it develops at spermarche, when spermatogenesis begins at puberty (Kormano, 1967), it is likely important for meiosis. In addition, it may immunologically isolate developing male gametes that are not recognized as “self” by the adult male immune system. In this sense, the value of a blood-testis barrier is fully realized after puberty, because foreign “antigens” on postmeiotic germ cells only exist after spermarche. A testicular insult such as biopsy, torsion, or trauma will not induce antisperm antibodies if it occurs before puberty. After puberty, however, immunologic infertility is a known risk (Turek, 1997). Clinically, the blood-testis barrier may also limit chemotherapy access to cancer cells sequestered behind it and result in isolated cancer recurrence within the testis.
Spermatogenesis
Spermatogenesis is a remarkably complex and specialized process of DNA reduction and germ cell metamorphosis. Older studies have estimated that the entire process in humans requires approximately 64 days (Clermont, 1972). However, a recent in-vivo kinetic study in healthy men revealed that the total time to produce an ejaculated sperm ranges from 42 to 76 days, suggesting that the duration of spermatogenesis can vary widely among individuals (Misell et al, 2006) (Fig. 20–13). Spermatogenesis involves (1) a proliferative phase as spermatogonia divide to replace their number (self-renewal) or differentiate into daughter cells that become mature gametes; (2) a meiotic phase when germ cells undergo a reduction division, resulting in haploid (half the normal DNA complement) spermatids; and (3) a spermiogenesis phase in which spermatids undergo a profound metamorphosis to become mature spermatozoa. (For excellent reviews, see Steinberger, 1976, and de Kretser and Kerr, 1988.)
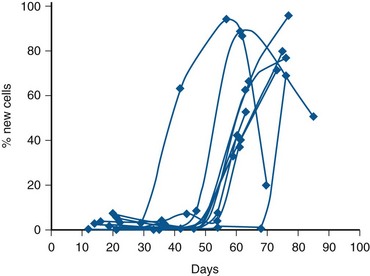
(From Misell LM, Holochwost D, Boban D, et al. A stable isotope/mass spectrometric method for measuring the kinetics of human spermatogenesis in vivo. J Urol 2006;175:242–46.)
A cycle of spermatogenesis involves the division of primitive spermatogonial stem cells into subsequent germ cells. Several cycles of spermatogenesis coexist within the germinal epithelium at any one time, and they are described morphologically as stages. If spermatogenesis is viewed from a single fixed point within a seminiferous tubule, six recognizable cellular associations or stages exist in a predictable and constant fashion in humans (Heller and Clermont, 1964) (see Fig. 20–11). In addition, there is also a specific organization of spermatogenic cycles within the tubular space, termed spermatogenic waves. The best evidence suggests that human spermatogenesis exists in a spiral or helical cellular arrangement that ensures sperm production is a continuous and not a pulsatile process (Schulze, 1989) (Fig. 20–14).
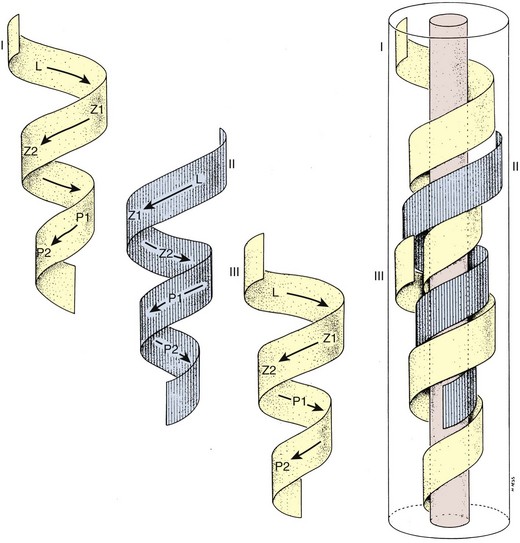
(From Schulze W, Rehder U. Organization and morphogenesis of the human seminiferous epithelium. Cell Tissue Res 1984;237:395–407.)
Testis Stem Cell Migration, Renewal, and Proliferation
Testis Stem Cell Migration
During early prenatal development, primordial germ cells migrate to the gonadal ridge and associate with Sertoli cells to form primitive testicular cords (Witschi, 1948). These primitive germline stem cells are termed gonocytes after the gonad differentiates into a testis by forming seminiferous cords. They are called spermatogonia after migration to the periphery of the tubule (Gondos and Hobel, 1971). Interestingly, these early migrating germ cells have properties very similar to embryonic stem cells and are likely the source of adult seminoma germ cell tumors (Ezeh et al, 2005).
Testis Stem Cell Renewal
Spermatogonia within the testis stem cell niche are replenished in a process termed stem cell renewal. The growth factor-receptor kit ligand/c-kit receptor system and the niche factor glial cell line-derived neurotrophic factor (GDNF) appear to be involved in this process (Oatley and Brinster, 2008). In fact, the c-kit receptor is a marker of spermatogonial stem cells in rats (Dym, 1994) and spermatogenesis in the rat is a c-kit–dependent process, whereas spermatogonial stem cell renewal may be c-kit–independent (Yoshinaga et al, 1991). Recent studies have also shown that human spermatogonial stem cells can be reprogrammed in vitro to become embryonic-like stem cells (Conrad et al, 2008; Kossack et al, 2009) (Fig. 20–15). Obtained from adult testis biopsies, the embryonic-like cells express distinct markers of pluripotency (OCT-4, SOX-2, STELLAR, GDF-3), can form all three germ layers, maintain a normal karyotype, form teratomas, and express appropriate levels of epigenetic markers and telomerase (Kossack et al, 2009). This finding suggests that, in the future, the testis may be a source of patient-specific, embryonic stem cells for cell-based therapy.
Testis Stem Cell Proliferation
In the human, pale type A (Ap) spermatogonia in the basal, stem cell niche of the seminiferous tubule divide at 16-day intervals (Clermont, 1972) to form B spermatogonia. B spermatogonia are committed to become spermatocytes, but the cytoplasm between spermatogonial daughter cells remains conjoined after mitosis, forming cytoplasmic bridges between adjacent cells. These cytoplasmic bridges are observed between all classes of germ cells throughout spermatogenesis (Ewing et al, 1980
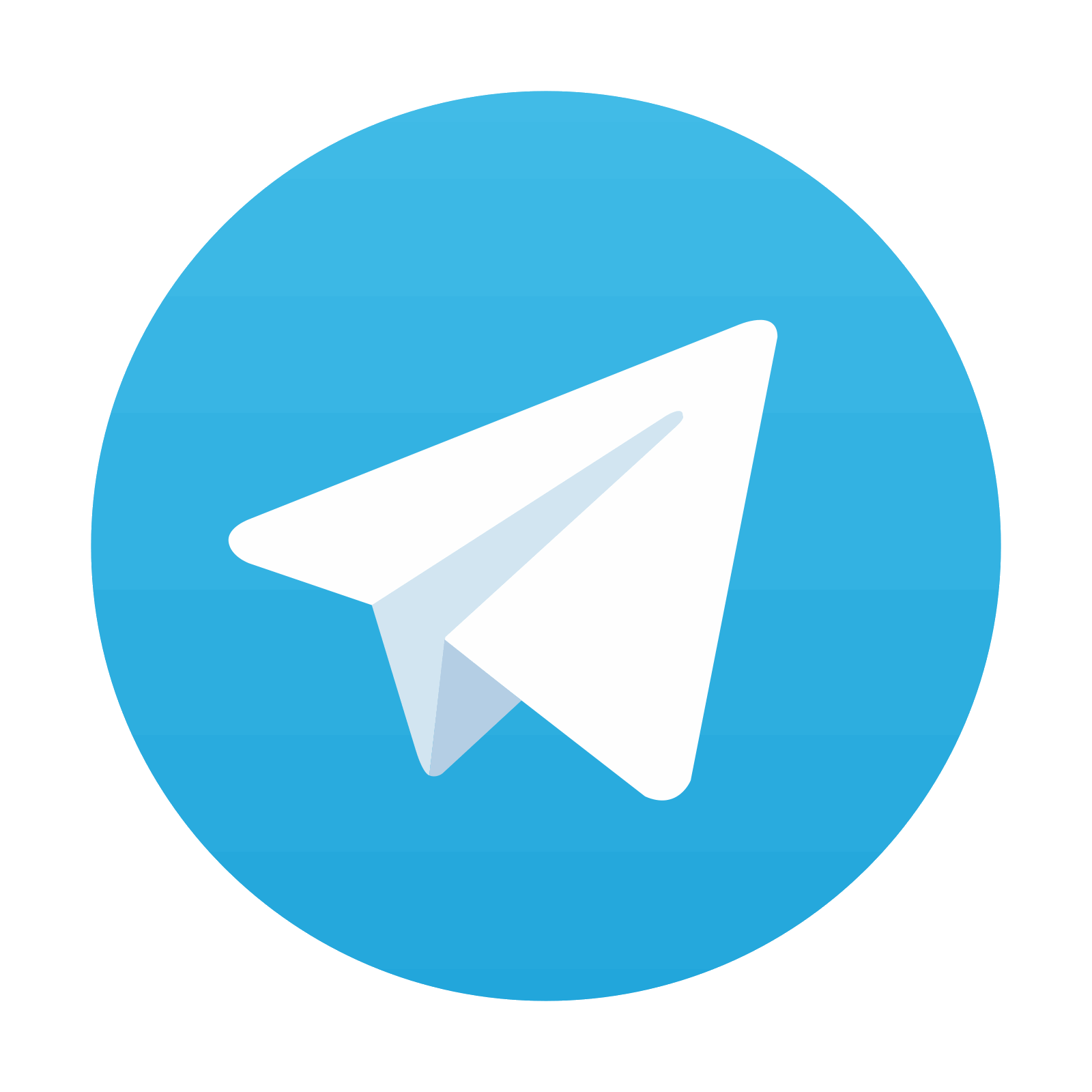
Stay updated, free articles. Join our Telegram channel
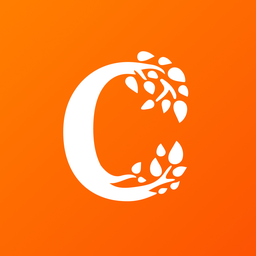
Full access? Get Clinical Tree
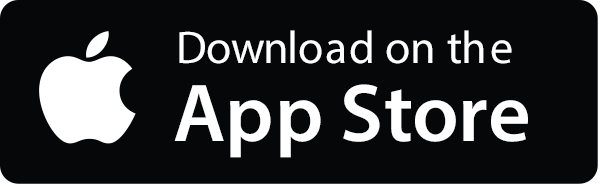
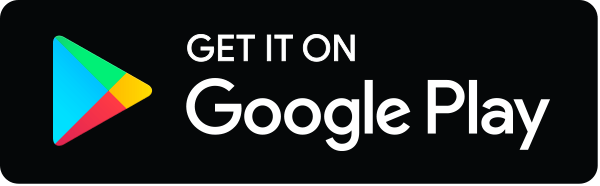
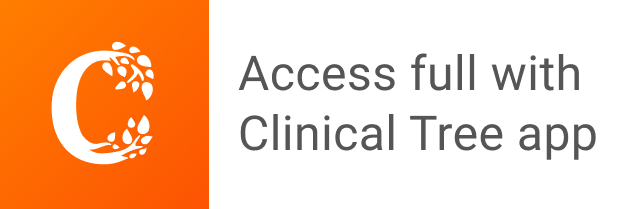