Fig. 7.1
Schematic representation of the current major strategies for kidney regeneration
7.2 Mechanisms of Kidney Regeneration
7.2.1 Cells Involved in Kidney Repair
Various types of cells are considered to be able to either contribute directly to renal repair after damage or substantially improve renal injury without direct contributing to the renal epithelium. The proper regulation of these cells and successful delivery are important for the development of kidney regeneration. There are four possible main origins for cells contributing to kidney repair: (1) interstitial cell transdifferentiation to epithelium [12], (2) recruitment of stem cells from the bone marrow [13–17], (3) tubular cell dedifferentiation and proliferation in response to injury [12, 18, 19], and (4) repopulation of the renal tubules by a resident kidney stem/progenitor cells [20–22]. The first two options involve nonepithelial cell transdifferentiation into renal epithelium, whereas the other two options involve epithelial cells within the renal epithelium itself. The theory that renal repair involves cells within the renal epithelium of the nephron was supported by lineage studies showing no evidence of dilution of the cap mesenchyme-derived (Six2+) tubular epithelium with a nonepithelial cell source [23]. However, this study did not answer whether any cell within the epithelium can contribute to repair or whether repair relied on a resident stem cell population within the tubules.
7.2.1.1 Renal Progenitor Cells
Although many studies have investigated the existence, location, and contribution of renal progenitor cells to epithelial repair [21, 22, 24, 25], existence and identification of renal stem or progenitor cells in the adult kidney tissues have been somehow controversial [3]. Grobstein first demonstrated the presence of renal progenitor cells in the metanephric blastema [26, 27]. Discrete populations of these renal progenitors have been shown to persist into adulthood, and numerous studies have identified renal progenitor cells in the proximal tubule [28], tubular epithelial cells [30], Bowman’s capsule [24], glomeruli [31], renal papilla [32], and cortical stroma. These renal progenitor cells possess high cloning efficiency, self-renewal potential, and differentiate into specific renal cell lineages, suggesting a role in renal regeneration [24]. Several transcriptional regulators, such as Six2 and Foxd+1, are essential for the persistence of these progenitor cell populations beyond development, while Pax2 is required for later differentiation [33, 34].
Renal progenitor cells could be harvested and isolated from adult human kidneys as well as human urine, expanded in vitro, maintain their renal phenotypes through several passages, and reimplanted [20, 35, 36]. Bussolati et al. showed that intravenously injected renal progenitor cells migrated to the site of tubular injury and contributed to renal repair [20]. These results may support that differentiated renal epithelial cells isolated from kidney tissue have proliferation capacity through dedifferentiation of the surviving epithelia during cell culture.
In contrast to the stem cells, renal progenitor cells can only differentiate to specific cellular lineage and display limited self-renewal capacity [37]. Renal progenitor cells are identified by cell marker CD133 and CD24. CD133 is a marker of several types of adult tissue stem cells, while CD24 is a surface molecule which is expressed in human metanephric mesenchyme [38, 39]. Renal progenitor cells can differentiate into glomerular as well as tubular epithelial cells. Tubular progenitor cells represent about 2–6% of all tubular epithelial cells in healthy adult kidneys and express CD133, CD24, vimentin, cytokeratins 7 and 19, Pax2, and nestin that are not expressed by differentiated tubular epithelial cells [20]. Glomerular progenitor cells localize within the Bowman’s capsule and can differentiate toward podocyte phenotype. These glomerular progenitor cells can express CD106, whereas tubular progenitor cells cannot express this surface marker.
Renal progenitor cells have higher resistance to injury compared to other differentiated cells of the kidney. When injected in severe combined immunodeficient mice affected by rhabdomyolysis-induced acute kidney injury, these cell populations displayed the capacity to integrate into the tubules, generate new tubular epithelial cells, and improve renal function [22]. It can be an attractive strategy if renal progenitor cells can be induced via lineage-instructive reprogramming or differentiated from extrarenal stem cells, such as mesenchymal, embryonic, and induced pluripotent stem cells. These regenerated exogenous renal progenitor cells may enhance renal repair combined with a small population of endogenous renal progenitor cells. In comparison to stem cells, renal progenitor cells have several advantages, such as no need of intermediate cell culture conditions and direct transit from one phenotype to another. However, a reliable method of inducing extrarenal stem cells to differentiate into renal progenitor cells has not been appropriately established [40, 41].
7.2.1.2 Proliferation of Mature Renal Epithelial Cells
Lineage tracing studies in mice suggested that the proliferating populations after kidney injury were mature renal epithelial cells rather than a specific progenitor cell population [42], whereas analysis of cell division using unbiased DNA labeling also suggested randomness in the cells reentering cell division within the tubule [43]. Anatomical reanalysis of human tubular epithelium has showed a distinct subpopulation of cells within the tubules referred to as scattered tubular cells [44]. The first study of scattered tubular cells performed by Berger et al provided the evidence that this cell population is not a defined intratubular progenitors of fixed morphology but may arise from any tubular cell as a result of spontaneously dedifferentiation triggered by local injury [45]. Indeed, it has been known that cells within the proximal tubule can express mesenchymal cell markers (vimentin) after kidney injury, suggesting a dedifferentiation process can occur in response to injury [19].
7.2.1.3 Mesenchymal Stem Cells
For decades, many studies demonstrated that cells from the bone marrow could migrate into the sites of injury around the body, including the kidney [17]. Although bone marrow-derived cells were initially considered to have a capacity to generate new tissue even in remote injured sites, several studies have showed it may not occur in some cases [46, 47]. Mesenchymal stem cells, a population of cells within the bone marrow, are clogenic, able to undergo proliferation, and to be differentiated into various cell types, including bone, cartilage, and fat [48]. Although they were originally investigated as a support population within the bone marrow, they have also been investigated for the therapeutic potential with respect to mesenchymal tissue replacement. Recent several studies suggested that bone marrow-derived mesenchymal stem cells are able to improve deteriorated renal function in response to kidney injury and to reduce allograft rejection [49–52]. However, the mechanisms of how these mesenchymal stem cells can improve renal function were not well established. This is not regarded as a result of integration into the damaged epithelium or transdifferentiation into an epithelial cell type. These beneficial effects are rather considered to be induced by paracrine effects and immunomodulatory capacity of mesenchymal stem cells [48, 53–59].
Although mesenchymal stem cells were originally identified in the bone marrow, mesenchymal stem cell-like cells have been also identified to reside in many adult tissues, including the kidney. It may represent a perivascular cell population involved in normal tissue repair [53, 54, 57]. Recently, a population of endogenous kidney mesenchymal stem cells was characterized from the adult murine kidney [60]. It showed the characteristic gene and protein expression profile, clonogenicity, and potential to differentiate to other cell types, such as bone, cartilage, and fat. This cell population showed enrichment for kidney-specific genes, compared to bone marrow-derived mesenchymal stem cells.
7.2.2 Renotropic Factors
Soluble factors involved in kidney development have been identified by gene targeting techniques, in vitro tubulogenesis models, and organ culture systems. The regeneration process after renal injury resembles the kidney organogenesis. Most of these factors have been proved to regulate renal recovery as potential renotropic factors by using animal kidney injury models. These renotropic factors are as follows: hepatocyte growth factor (HGF) [61], epidermal growth factor (EGF) [62], heparin-binding EGF-like growth factor (HB-EGF) [63, 64], bone morphogenetic protein-7 (BMP-7) [65, 66], insulin-like growth factor-I (IGF-I) [67, 68], platelet-derived growth factor (PDGF) [69], and uterine sensitization-associated gene-1 (USAG-1) [70]. In addition, several key regulatory molecules required for renal organogenesis, such as Pax-2, leukemia inhibitory factor, and Wnt4, are reactivated in regenerating tubular cells after ischemic renal injury [71–73].
Recently, the role of these renotropic factors in kidney injury has been demonstrated. Chen et al. showed the importance of EGF receptor activation in the recovery phase after acute kidney injury through mice with a specific EGF receptor deletion in renal proximal tubules [74]. Deletion of the BMP receptor activin-like kinase 3 in the tubular epithelium enhances epithelial damage and fibrosis [75]. Conditional knockout mice lacking the HGF receptor, c-met, specifically in renal tubules demonstrated the antiapoptotic of anti-inflammatory effect of c-met signaling in renal protection after acute kidney injury [76].
A negative regulatory factor of kidney repair has also been identified. Several studies using transgenic mice expressing truncated activin type II receptor, an in vitro tubulogenesis model, the Wolffian duct culture, and isolated rat embryonic kidney culture showed that activin A is an endogenous inhibitor of renal organogenesis [77–83]. Activin A is also a potent inhibitor of renal regeneration after kidney injury [84].
Most of these renotropic factors can induce tubular cell proliferation after kidney injury when exogenously administered. However, it is still not well known whether these factors are involved in cell maturation, modulation of renal blood flow, neutrophil infiltration, and restoration of polarity. It will be interesting to examine if these renotropic factors promote renal regeneration via the activation of intrinsic renal stem cells.
7.2.3 Macrophage
Generally, macrophages have a function to clear foreign pathogens in the body that can disrupt tissue homeostasis [85] and also play an important role to mediate kidney repair. Although macrophages are well known to promote the fibrotic and inflammation reaction during renal disease, they have been also demonstrated to have a protective role in acute and chronic kidney diseases [86]. Moreover, Wyburn et al. reported that macrophages can ameliorate allograft rejection [87].
Macrophages represent a heterogeneous population with distinct functions depending on resident tissue and phase of tissue injury or disease. In some cases, macrophages display classical activation through the release of nitric oxide, reactive oxygen species, and proinflammatory cytokines, termed an M1 response or phenotype [88, 89]. In another stage of disease, macrophages can play antifibrotic and anti-inflammatory roles, termed M2 phenotype [90, 91]. These M2 macrophages have a reparative function in ischemia-reperfusion injury and obstructive nephropathy, induced by unilateral ureteric obstruction [92–95]. Many studies reported that changes in the local microenvironment are important to trigger an M1 phenotype macrophages, such as macrophage colony-stimulating factor, which can be released by tubular epithelial cells to stimulate this process [96, 97]. Based on these findings, macrophages were directed toward an M2 phenotype via genetic manipulation or cytokine alteration, such as overexpression of interleukin 4 (IL-4), IL-10, inducible nitric oxide synthase, or heme oxygenase, resulting in improved renal function [98–106]. In terms of regenerative medicine, macrophages are considered to have therapeutic potential and be one of the options for cellular or cytokine/growth factor-based therapies in kidney diseases.
7.2.4 Signaling Pathways in Mediating the Regenerative Process
Many cells, such as renal progenitor cells, endogenous renal tubular epithelial cells, bone marrow-derived stem cells, and macrophages, are involved in kidney regeneration. However, the exact mechanism of cell to cell interaction and influencing factors for cell reaction involving regenerative process after kidney injury is still not exactly known. Although several studies suggested possible pathways involved in kidney regeneration, further research to identify the factors in specific signaling pathways will be needed.
7.2.4.1 PI3K/AKT/mTOR Pathway
It has been demonstrated that growth factors, such as EGF, IGF-1, and HGF, can accelerate recovery of renal function after acute kidney injury [107]. These growth factors activate a lipid kinase (phosphatidylinositol-3 kinase, PI3K) that phosphorylates phosphatidylinositol 4,5-bisphosphate to yield phosphatidylinositol 3,4,5-trisphosphate. The phosphatidylinositol 3,4,5-trisphosphate phosphorylates and activates Akt. After being activated, Akt stimulates mammalian target of rapamycin (mTOR) by regulating the activity of intermediary kinases. The activation of mTOR leads to phosphorylation of downstream substrates and then induces cell regeneration. It is demonstrated that inhibition of mTOR by rapamycin delays recovery of renal function [108]. Akt can activate antiapoptotic genes and might inactivate proapoptotic factors, such as Bcl-2-associated death promoter, forkhead family transcription factors, and procaspase-9 [109]. Deletion of the EGF receptor in renal proximal tubular epithelial cells impairs phosphotidylinositol-3 kinase/Akt signaling and delays recovery from acute kidney injury [74].
7.2.4.2 Wnt/GSK3/β-Catenin Pathway
The Wnts are a family of secreted and glycosylated protein ligands. Wnt signals can inhibit glycogen synthase kinase 3 (GSK3) by phosphorylation. When GSK3 is inhibited, β-catenin is stabilized and translocates into the nucleus to act as a transcriptional coactivator of the T-cell factor/lymphoid enhancer-binding factor family or transcription factors and drive the expression of its target genes [110]. This pathway is involved in the regulation of cell fate, cell mobility, proliferation, survival, protein synthesis, and glycogen metabolism [111]. Acute kidney injury induces activation of Wnt pathway, whereas genetic inactivation of Wnt signaling can deteriorate renal function recovery and kidney regeneration [112]. Among the increased Wnt ligands after kidney injury, macrophage-derived Wnt7b can promote tubular epithelial cell regeneration and kidney repair. In the downstream of Wnt signaling, GSK3 is inhibited by Wnt ligands, IGF, EGF, and fibroblast growth factors 16, 19, and 23 [113]. In acute kidney injury, GSK3 can promote the systemic inflammatory response and participate in a number of apoptotic signaling pathways by phophorylating transcription factors that regulate apoptosis [114]. TDZD-8, a GSK3β inhibitor, can inactivate ischemia-induced activation of GSK3, caspase 3, and Bax, ameliorate tubular epithelial cell apoptosis, and protect renal function [115]. Acute kidney injury can induce the expression of β-catenin, and renal tubule-specific knockout of endogenous β-catenin aggravates kidney injury in mice [116]. It is also reported that inhibition of GSK3β can ameliorate nonsteroidal anti-inflammatory drug-induced acute kidney injury [117]. Consequently, it is considered that activation of the Wnt/GSK3/β-catenin pathway is beneficial for kidney disease and GSK3 inhibitor can be a target of therapeutic agents in the future.
7.2.4.3 JAK/STAT Pathway
When EGF binds to the EGF receptor, Janus-activated kinase (JAK) is activated and phosphorylates the intracellular domain of the receptor and allows recruitment and phosphorylation of STAT. Salahudeen et al. demonstrated that erythropoiesis-stimulating proteins suppress renal tubular cell apoptosis in vitro and enhance renal recovery in the cisplatin-induced acute kidney injury through the activation of JAK/STAT pathway [118]. In contrast, Ucero et al. reported that inhibition of the JAK/STAT pathway can decrease tubular epithelial cell apoptosis and kidney inflammation in murine acute kidney injury model [119]. (Therefore, further researches will be needed to clarify the effect of JAK/STAT pathway on renal repair.)
7.2.4.4 MAPK/ERK Pathway
Mitogen-activated protein kinases (MAPKs) are a family of kinases that have been commonly investigated on the kidney disease. There are four different MAPK pathways: extracellular signal-regulated kinase-1 and extracellular signal-regulated kinase-2 (ERK1/2), extracellular signal-regulated kinase-5 (ERK5), c-Jun N-terminal kinase (JNK), and p38MAPK [120, 121]. ERK is mainly activated by mitogenic stimuli, such as growth factor, and ERK1/2 pathway has been widely studied in kidney regeneration. It was demonstrated that activation of ERK pathway can enhance renal epithelial cell survival during oxidative injury in vitro [122]. Activation of the signal transducer and activator of transcription-3 (STAT3) during oxidative stress can attenuate EGF receptor-mediated ERK activation and renal tubular cell survival [123]. It was also reported that inhibition of ERK pathway reduces kidney regeneration in rats with myoglobinuric acute kidney injury in vivo [124].
7.3 Improving Renal Function After Kidney Injury
7.3.1 Cell-Based Approach
Early studies using stem cells for kidney regeneration usually have shown that the predominant recovery mechanism of the acute kidney injury was mediated through the transdifferentiation of the infused mesenchymal stem cells into specific renal cell types, especially podocytes, renal tubular cells, glomerular cells, and mesangial cells [13, 125–127]. More recent studies support the concept that cytokines and/or growth factors secreted from the injected mesenchymal stem cells stimulate endogenous cells to proliferate and regenerate the infused renal tissues [128–131]. Several studies suggested that the effect of mesenchymal stem cells in acute kidney injury models is associated with paracrine mechanisms, such as immune-related response and mitogenic, antiapoptotic, and vasoprotective effect [132, 133]. It was evidenced by the results of several studies that bone marrow-derived mesenchymal stem cell-cultured medium contains microvesicles and growth factors that reduce inflammation and enhance renal repair through interactions with renal progenitors [15, 125, 134, 135].
Bone marrow-derived mesenchymal stem cells are multipotent, migrate across tissues, are easily harvested, are produced throughout life, and contribute to the repair of organs [136]. The administration of bone marrow stem cells has been shown to promote neovascularization, reduce inflammation, inhibit apoptosis, and stimulate differentiation and proliferation in multiple systems [137]. Poulsom et al. first reported the effect of engrafted bone marrow stem cells into the damaged kidney, improving repair of renal tissues after acute kidney injury [17]. However, despite these benefits of bone marrow stem cells, clinical application is still in question because negative results were also reported. Bone marrow stem cells have been shown to promote interstitial fibrosis in mouse models, and direct renal injection of bone marrow stem cells induced the development of angiomyeloproliferative lesions of unknown neoplastic potential [138, 139]. Therefore, further safety studies are necessary before clinical applications to ensure safe and successful outcomes for kidney regeneration using bone marrow stem cells.
Another promising cell source of mesenchymal stem cells is adipose-derived stem cells. Several studies reported the effectiveness of adipose-derived stem cells to improve renal functions in animal models, including mouse, rat, and pig [140–143]. Administration of adipose-derived stem cells into an acute kidney injury model reduced renal fibrosis at 6 weeks [144], and intrarenal injection of adipose-derived stem cells showed improved angiogenesis and preserved the renal structure integrity, which restored renal function at 14 days [145].
Amniotic fluid-derived stem cells are easily harvested and cultured with lower risk of tumorigenicity and similar multipotency to bone marrow stem cells. Rota et al. demonstrated the therapeutic potential of human amniotic fluid stem cells [146]. In their study, engrafted amniotic fluid stem cells localized primarily to the peritubular region, limiting tubular damage, improving renal function, and prolonging survival of the animals. They also reported that amniotic fluid stem cells that were preconditioned with glial cell line-derived neurotrophic factor were observed to promote better functional recovery by contributing to renal regeneration in acute kidney injury models when compared to amniotic fluid stem cells without treatment. The authors suggested that a combination of transdifferentiation of amniotic fluid stem cells and paracrine signaling involved the regenerative effect through this study. Hauser et al. reported that intravenous infusion of amniotic fluid stem cells induced more rapid recovery of renal function in an animal model of acute kidney injury, when compared to that of bone marrow stem cells. In this study, bone marrow stem cells showed higher potential for proliferation, whereas amniotic fluid stem cells showed better capacity for antiapoptosis and persistence within the peritubular capillaries. In addition, they isolated different cytokines and growth factors from bone marrow and amniotic fluid stem cells, suggesting different modes of action between both cell sources.
Induced pluripotent stem cells (iPSCs) have gained increasing interest in the field of regenerative medicine, as they possess pluripotent capability and potentially provide an inexhaustible source of patient- and tissue-specific stem cells. Recently, iPSCs have been successfully generated from human renal sources, such as kidney mesangial cells, proximal tubular cells, podocytes, and epithelial cells derived from urine [147–149]. IPSCs have several advantages compared with embryonic stem cell, such as absence of ethical issues related to cell sourcing, fewer immune rejection, and less potential for abnormal tissue formation. Moreover, they also have more capabilities to facilitate targeted, organ-specific differentiation due to retaining the epigenetic pattern of the parent cell [150]. Recent studies reported the improvement of renal functions in an acute kidney injury rodent model after administration of iPSCs [151, 152]. However, iPSCs have been shown to express abnormal genes and induce T-cell-dependent immune reaction in syngeneic mice [153]. This unexpected immune response is a big hurdle of iPSCs for clinical application.
Aforementioned renal progenitor cells are also promising cell sources for the treatment of acute kidney injury. It has been demonstrated that CD24+/CD133+/CD106- renal progenitor cells are resistant to apoptosis and promote recovery of tubular injury in the rhabdomyolysis-induced acute kidney injury rat model [22]. Neural cell adhesion molecule (NCAM)-positive cells that were identified from human kidney tissue showed clonogenic and renal progenitor properties [154]. After engraftment into the chick embryo, NCAM+ human nephron progenitor cells formed a renal-like structure. Administration of human nephron progenitor cells into the kidneys of acute kidney injury rat model induced inhibition of disease progression and improvement of renal function. Taken together, these results support the concept that renal progenitor cells have the capacity to migrate and proliferate to improve structural and functional damage after acute kidney injury.
7.3.2 Developmental Biology
Although advancement of stem cell technology has showed promising results in the field of kidney regeneration, stem cells themselves have limitations to maintain the structure of the kidney. Thus, many researchers tried to develop the cellular approach by incorporating principles of developmental biology. This investigative field sought to create, implant, and maintain a renal structure that mimics the physical and physiological characteristics of the native kidney.
The concept of early investigations in this field endeavored to infuse new nephrons to developing kidneys by implantation of embryonic metanephric tissue into the renal cortex of neonatal mice [155]. Metanephros is the embryological precursor of the adult kidney, characterized by mesenchyme and ureteric buds (Fig. 7.2) [156]. Transplantation of primitive metanephric tissue over fully developed kidney may have some advantages, such as reduced immunogenicity due to the absence of native vasculature and antigen-presenting cells [156, 157]. Thus, metanephros can be maintained in vivo without host immunosuppression. Although transplanted metanephric tissue was successfully differentiated and developed into functional nephrons in the host kidney of neonate, it was not initially reproduced in host kidneys of adult due to lack of differentiation and acute graft rejection [158]. However, another study reported successful implantation and differentiation of metanephroi into adult hosts, with demonstrated glomerular filtration and plasma clearance after modifying the protocol [156]. Encouraged by these results, further studies to investigate the possibility of xenotransplantation have been performed. More information related to xenotransplantation will be discussed in another section.


Fig. 7.2
Isolated metanephros from embryo of E15 transgenic mouse in which a green fluorescent protein is expressed in the ureteric bud under the control of the Hoxb7 promoter. (a) Only the ureter can be distinguished from metanephric mesenchyme under the vision of light microscope. (b) Ureter and branches of ureteric bud can be seen with green color under the vision of immunofluoro-microscope
7.4 De Novo Organ Regeneration
The basic concept for regeneration of solid organ is to collect stem or progenitor cells from appropriate cell sources and expand these cells in culture with or without differentiation. Subsequently, cells are seeded into a scaffold and placed into a bioreactor to promote cell acclimation, attachment, and maturation. Then, finally this cell-seeded scaffold can be implanted in the host [159, 160].
7.4.1 Artificial Scaffold
Extracellular matrix is the naturally occurring scaffold material secreted and manufactured by the resident cells of each organ, and it plays a critical role in development and repair of organ [161, 162]. The extracellular matrix is a complex three-dimensional framework of structural and functional proteins in a state of dynamic equilibrium within the surrounding tissues and provides the means by which cells communicate with each other and the external environment [163]. The extracellular matrix contains growth factors and bioinductive cytokines, which facilitate the reparative process, cell attachment, and tissue integration [164, 165]. Consequently, the roles of extracellular matrix for organogenesis and regeneration can be categorized by providing a three-dimensional scaffold for the spatial organization of cells, secreting and storing growth factors and cytokines, and regulating signal transduction [166].
7.4.1.1 Bioengineered Scaffold
Development of biomaterial engineering has produced bioengineered scaffolds that facilitate improved differentiation of transplanted cells. The first histocompatible functional kidney using bioengineered scaffold was generated by Lanza et al. in 2002 [167]. They used a nuclear transplantation technique in which dermal fibroblasts isolated from an adult cow were transferred into enucleated bovine oocytes and then transferred into progestin-synchronized recipients. Metanephroi taken from embryos were dissociated to single-cell suspension using collagenase, and the cells were expanded in vitro. Then, the cells were seeded onto a specialized polymer tube as the artificial scaffold. The cell-seeded scaffold was implanted into the same cow from which the cells had been cloned and produced urine-like liquid. Histologic analysis showed that it had well-differentiated kidney-like structure, including organized glomerulus-like, tubular-like, and vascular components. This investigation established the possibility of kidney regeneration using bioengineered scaffolds.
Synthetic biodegradable and biocompatible polyesters, such as polyglycolic acid (PGA), polylactic acid-polyglycolic acid (PLGA), and polycaprolactone (PCL), also can be used as a scaffold for kidney regeneration. Although these synthetic polymers are a US Food and Drug Administration (FDA)-approved biocompatible material, they have critical drawbacks, such as producing acidic products during degradation and inducing an inflammatory response in the host, which restricts the clinical applications [168–172]. To overcome these adverse effects of synthetic biomaterial, several investigations are in progress.
7.4.1.2 Decellularized Cadaveric Scaffold
Artificial scaffold also can be generated from organs of animal or human’s cadaver through decellularization [173–176]. The decellularization process can remove DNA, cellular material, and cell surface antigens from the extracellular matrix scaffold while preserving the structural and functional characteristics of vascular channels. The process of decellularization includes the repeated irrigation of cadaveric organs with acids or detergents through the innate vasculature, although organs with higher fat component, such as pancreas, often require the addition of lipid solvents [177]. To generate cadaveric scaffold, complete decellularization must be achieved because residual cellular material may contain antigenic epitopes that trigger inflammatory reactions and compromise subsequent recellularization [178, 179]. Ethylene oxide or paracetic acid can effectively sterilized the extracellular matrix without denaturing the extracellular matrix proteins or growth factors after decellularization [180, 181].
Using this technique, a functional artificial rat heart using a cadaveric heart as a scaffold was successfully generated [182]. For this, a whole-heart scaffold with intact three-dimensional geometry and vasculature was created via coronary perfusion with detergents into the cadaveric heart, followed by repopulation with neonatal cardiac cells or rat aortic endothelial cells and cultured under physiological conditions to stimulate organ maturation. In this study, the injected neonatal cardiac cells formed a contractile myocardium, which performed the stroke function. Decellularized cadaveric scaffolds have also been used to generate other organs, such as the liver, respiratory tract, and urinary bladder [183–185].
Recent advancement of decellularization-recellularization technique enabled to generate more complex organs, such as the kidney. Ross et al. first reported successful regeneration of an entire kidney using the decellularized rat kidney as a scaffold [186]. After fabricating intact scaffold, murine embryonic stem cells were injected through the innate vasculature. In this study, embryonic stem cells were used for seeding population because of their high doubling capacity, pluripotency, and potential to differentiate and integrate into primordial kidney cultures. The kidney scaffold successfully supported the growth and migration of the seeded embryonic stem cells within glomerular, vascular, and tubular structures while inducing differentiation down renal cell lines. Immunohistochemical analysis indicated that the seeded embryonic stem cells had lost their embryonic appearance and had shown gross morphological changes consistent with mature kidney cells, as well as expression of immunohistochemical markers of renal differentiation, such as Pax-2, Ksp-cadherin, and pan-cytokeratin. Through this study, the authors suggested several points to advance this research field that pretreatment of the embryonic stem cell with prodifferentiation agents, such as retinoic acid, activin-A, and BMP-7, may promote implantation and proliferation by providing a more kidney-specific lineage. Moreover, while injected cells through innate vascular channels can be evenly distributed in the renal cortex, the cells may not localize in the collecting system. Thus, retrograde seeding via ureter was attempted to resolve this problem, but this method caused uneven cell dispersion. Furthermore, murine scaffolds are not feasible for adult human kidney regeneration in terms of size and structure. Therefore, organs of larger animals, such as pig, or primates had to be considered to be investigated using this technique to meet human renal requirements.
However, application of this decellularization and recellularization technique to larger and complex organs is a great challenge, because larger organs with greater parenchymal mass require stronger detergents, higher perfusion pressures, and prolonged detergent exposure time that may damage the native vasculature and extracellular matrix proteins [187]. The first trial to decellularize primate kidney performed by Nakayama et al. confirmed effective decellularization with preservation of native extracellular matrix architecture and protein complement [188]. After recellularization, immunohistochemical analysis showed effective local migration and attachment of renal cells to the border of scaffold, demonstrating the feasibility of primate kidneys for decellularization and recellularization technique.
More recently, intact, whole-organ extracellular matrix scaffolds were generated from porcine kidneys [187, 189]. These studies reported successful decellularization using detergent perfusion through the innate vasculature and confirmed total cell clearance with preserving the architecture of scaffold, including glomeruli, tubules, and vasculatures. Despite these advances of generating decellularized scaffold technique, regeneration of a whole functional kidney using this method is still not achieved. Although several attempts have been performed, effective seeding population of cells was not established. Moreover, it is unclear whether larger kidney scaffold can support the retrograde ureteric perfusion to recellularize the collecting system. It is also not certain whether extracellular matrix of the decellularized kidney scaffold can support the proliferation and differentiation of stem cells and whether recellularization by perfusion through the innate vasculature does not cause thrombogenic complications after reimplantation. Furthermore, even after reliable protocols of recellularization are established, various functions of regenerated kidney, such as homeostasis, resorption, endocrine, metabolism, and immunomodulation, should be confirmed.
7.4.2 Isolation of Cells
The kidney has approximately 20 different specific cell types. For efficient kidney regeneration, all cells must be characterized for repopulation. Although many attempts have been performed to establish a reliable cell source and optimal cell growth conditions to provide adequate enrichment for achieving stable renal cell expansion systems, it is still challenging. It is essential to isolate feasible stem or progenitor cells for cell expansion and differentiation in vitro, and renal stem/progenitor cells are one of the good candidates. There are five different methods which have been used to isolate renal stem/progenitor cells from the adult kidney.
7.4.2.1 Label-Retaining Cells
One of the characteristics that distinguish stem cells from differentiated cells is their infrequent cell division. To conserve the proliferation capacity for a lifetime and to prevent genetic injuries during mitosis, the cycle of stem cells is very slow. Renal stem/progenitor cells were isolated using this property. When these cells are labeled with markers such as 5-bromo-2-deoxyuridine (BrdU) and histone 2B-GFP (H2B-GFP), they can retain the label for a long time. Then, these slow-cycling label-retaining cells (LRCs) can be detected following a chase period. Oliver et al. administered BrdU to rat and mouse neonates and found BrdU-retaining cells localized in the papilla, after chasing them for more than 2 months [32]. After the induction of ischemia, LRCs disappeared from papilla, whereas most of them expressed Ki67, which is a proliferation marker. Through this study, the authors demonstrated that the renal papilla is a niche for adult kidney stem cells and that LRCs proliferate and migrate following ischemic kidney injury. They also showed similar results in another study using H2B-GFP transgenic mice [190]. H2B-GFP is more stable and easily detected than BrdU, and it can label the cells in a cell cycle regardless of the stage, whereas BrdU can only label the cells in the S phase [191]. In this study, H2B-GFP-positive cells proliferated and migrated to renal tubules after kidney injury.
Maeshima et al. identified LRCs using BrdU predominantly in proximal tubules of the adult rat kidney [30]. After ischemic injury, most of the LRCs were found in tubules and were positive for PCNA, indicating that LRCs proliferate after injury. The LRCs were successfully isolated and cultured, and they initially expressed vimentin, a mesenchymal stem cell marker, and eventually became positive for E-cadherin, an epithelial cell marker, after multiple cell divisions. In another study, they demonstrated that LRCs became positive for proximal tubule and ureteric bud markers when transplanted into the metanephric kidney of embryonic rat, indicating that LRCs were integrated into epithelial components of the nephrons in the process of kidney development [192].
Although labeling with BrdU is an effective technique to detect stem cells, considerable problems were also suggested. The BrdU label may be released from dying cells and taken up by adjacent dividing cells [193]. Moreover, the possibility that BrdU-retaining cells are not stem/progenitor cells but fully differentiated cells was proposed [194]. Vogetseder et al. reported that LRCs expressed differentiated tubule markers, such as Na-K-ATPase, NaPi-IIa, PMP70, and megalin. Similarly, H2B-GFP labeling has also drawbacks because the labeling is diluted after massive cell proliferation. To obtain a better understanding of LRCs, a more accurate purification and characterization technique are required.
7.4.2.2 Side Population Cells
Since stem cells extrude dyes, such as Rhodamine 123 and Hoechst 33342 via ATP-binding cassette transporters, they are located in a unique position in fluorescent-activated cell sorting (FACS) analysis and are called side population (SP) cells [195]. SP cells are characterized as Hoechst low cells and are isolated from several tissues, including the heart, liver, and skeletal muscle. Several groups isolated and characterized SP cells from the kidney. Iwatani et al. isolated SP cells from adult rat kidney, but these SP cells were not involved in kidney repair following gentamicin-induced nephropathy [196]. Hishikawa et al. isolated SP cells from adult mouse kidney [197]. These cells expressed Musculin/MyoR, a transcription factor found in skeletal muscle precursors. Musculin/MyoR-positive cells reside in the renal interstitium and are decreased following cisplatin-induced acute kidney injury. Systemic injection of kidney SP cells into mice improved renal function in cisplatin-induced acute tubular injury model. In addition, SP cells expressed a high level of leukemia inhibitory factor (LIF) and renoprotective factors, such as HGF, vascular endothelial growth factor (VEGF), and BMP-7 in a cisplatin-induced acute kidney injury model. Challen et al. also isolated SP cells from adult mouse kidney, and these cells expressed genes involved in Notch signaling. These SP cells are located predominantly in the proximal tubules and integrated into the mesenchyme- and ureteric bud-derived structures when injected into the embryonic kidney, suggesting that they have multilineage differentiation potential. Administration of these cells improved renal function in adriamycin-induced kidney injury model, but these cells were barely incorporated into the renal tissues after infusion. Taken together, SP cells are considered to release renoprotective factors in paracrine fashion, but the exact function or composition of SP cells is still unclear.
7.4.2.3 Cell Surface Markers
Another approach for isolating putative stem cells is utilizing stem cell markers, such as CD133, CD24, and stem cell antigen-1 (Sca-1). Although CD133 is not a specific marker for kidney stem cells, it is a universal marker for stem cells in other tissues, such as hematopoietic stem cells, cancer stem cells, and vascular endothelial progenitor cells [198, 199]. Bussolati et al. isolated CD133+ cells from normal adult human kidney [20]. These cells expressed Pax-2 (embryonic kidney marker), but not CD34, CD45 (hematopoietic lineage marker), CD 90, or c-Kit (stem cell markers), indicating that they were originated from renal tissues. They were capable of self-expansion and limited self-renewal and could be induced to differentiate into epithelial and endothelial cells in vitro and in vivo. When CD133+ cells were injected intravenously in a glycerol-induced acute kidney injury model of severe combined immunodeficiency mice, they migrated to the injured kidney and were incorporated predominantly into the proximal and distal tubules. Sagrinati et al. isolated CD24+ and CD133+ cells from parietal epithelial cells in the adult human kidney after culturing glomeruli [24]. These cells also expressed the stem cell-specific transcription factors, such as Oct-4 and BmI-1. These cells had a high self-renewal potential, and when cultured in appropriate conditions, these cells could be differentiated into tubular epithelial cells, osteocytes, adipocytes, and neuronal cells in vitro. These cells were incorporated into regenerating tubules, and renal function was improved when they were administered intravenously into severe combined immunodeficiency mice with glycerol-induced acute kidney injury. Dekel et al. isolated Sca-1-positive cells from adult mouse kidney [200]. The Sca-1+ cells were located mainly in the papilla and could differentiate into myogenic, osteogenic, adipogenic, and neural lineages in vitro. When injected into the renal parenchyma of ischemia-induced acute kidney injury model, some of these cells were integrated into renal tubules.
7.4.2.4 Cell Culture
Selective culture conditions are useful to isolate stem cells [201]. A unique cell population can be isolated during the culture of dispersed cells derived from the adult kidney. Gupta et al. isolated progenitor-like cells from adult rat kidneys by using culture conditions similar to those used for bone marrow-derived cells [202]. These cells were characterized by long-term self-renewal without senescence and the expression of vimentin, CD90, Pax-2, and Oct-4 without any markers of major histocompatibility complex (MHC) class or other differentiated cell markers. These cells can also be induced to multiple lineages in vitro, including hepatocytes, neurons, and endothelial cells. The cells could differentiate into renal tubular cells when injected under the capsule of the kidney or intra-arterially in uninjured kidney or ischemia-reperfusion injury of the kidney. It is proposed that these progenitor cells isolated by using selective culture condition participate in the regenerative response of the kidney to acute injury. However, possibility of contamination of differentiated kidney cells and blood cells is a limitation of this method.
7.4.2.5 Parietal Epithelial Cells
Mature podocytes are highly differentiated nondividing cells, and it is not clear whether damage of podocyte in adulthood can be repaired. Glomerular epithelial stem cells may reside in the kidney and may be capable of regenerating podocytes, and it was suggested that glomerular epithelial stem cells exist in the parietal epithelial cells [203]. It was reported that some of the parietal epithelial cells localized in the Bowman’s capsule of adult human kidney have the characteristics of stem cells [24]. In this study, parietal epithelial cells were divided into three subpopulations according to the locations and characters. First, cells localized at the urinary pole expressing CD24 and CD133, but not podocalyxin (PDX), a differentiated podocyte marker (CD24+, CD133+, PDX- cells), could regenerate both tubular cells and podocytes. Second, cells localized between the urinary pole and the vascular pole expressing both progenitor and podocytes markers (CD24+, CD133+, PDX+ cells) could only regenerate to podocytes. Finally, cells localized at the vascular pole did not express progenitor markers but displayed phenotypic features of differentiated podocytes (CD24-, CD133-, PDX+ cells). Moreover, the in vivo properties of the cells were also evaluated by Ronconi et al [204]. Administration of CD24+, CD133+, and PDX- cells, but not CD24+, CD133+, PDX+ or CD24-, CD133-, and PDX+ cells, into mice with adriamycin-induced kidney injury reduced proteinuria and improved glomerular damage, suggesting that CD24+, CD133+, and PDX- cells are potential therapeutic targets for glomerular disorders characterized by podocyte injury. Appel et al. reported that the CD133+ parietal epithelial cells have the capacity to proliferate, migrate along the glomerular tuft, and differentiate to mature podocytes after podocyte injuries by using immunostaining, label retention, and transgenic mice approaches [205]. The cells at the base of the vascular pole adjacent to the podocytes were stained with both the parietal epithelial cell marker (claudin-1) and podocyte-specific markers (nestin, dipeptidyl peptidase IV, aminopeptidase A). The idea that parietal epithelial cells migrate to become podocytes was also supported by BrdU staining of rat parietal epithelial cells and genetic tagging of parietal epithelial cells utilizing parietal epithelial cell-specific promoter (hPODXL1). The researchers demonstrated that the cells at the urinary pole of the Bowman’s capsule migrate down to the proximal tubules and are involved in the turnover of tubular epithelium. Finally, they concluded that the cells localized at the border of different compartments have features of progenitor cells.
7.4.3 Potential Cell Sources for Renal Regeneration
Recent advances in stem cell biology and cell culture techniques have facilitated the development of cell therapy for clinical translation [206, 207]. Compared with other approaches for kidney regeneration, this method using cellular approach can be more practically applied to kidney tissue regeneration due to the relatively simple cell manipulation process, easy access to the target site in a less invasive manner, and effective integration of administered cells with the host tissues. Consequently, many studies have been performed using this cellular approach to treat renal failure [3, 41, 206]. In this section, cellular approach for kidney tissue regeneration, including various cell sources used as well recent advances made in preclinical and clinical studies, will be discussed.
7.4.3.1 Kidney Tissue-Derived Cells
Although multipotent stem cells have been known to play important roles for various tissues and organs of humans, no definite evidence to date establishes the existence of a pluripotent, self-renewing cell population in the adult kidney. During kidney development, condensed mesenchyme around the tips of the ureteric bud possesses self-renewing cells capable of generating all other elements of the nephrons, interstitium, and vasculature via a mesenchyme-epithelial transition [208]. The cells of condensed mesenchyme are regarded as the renal stem cell population. These stem cells of condensed mesenchyme cease asymmetric division and self-renewal and then exhibit spontaneous commitment to mesenchyme-epithelial transition. However, these cells can be exhausted before the perinatal stage [209]. This phenomenon indicates that complete regeneration involving a complete replacement of the lost nephron does not occur in the mammalian kidney. Nevertheless, researchers have discovered the stem cell-like pluripotent cells in adult kidney, which are called renal progenitor cells, and there are two major theories to explain the origin of proliferating tubular epithelia after acute kidney injury. The first one is that stem or progenitor cells in renal tubule can generate new epithelia, and the other one is that fully differentiated epithelia may dedifferentiate, reenter cell cycle, and generate new epithelial cells through self-duplication.
Primary Kidney Cells
Kidney tissue consists of more than 20 specialized cell types that are structurally organized into functionally and anatomically distinct compartments. Primary renal cells can be harvested from both normal and diseased kidney tissues and expanded in vitro while maintaining the phenotype and function which they are derived. Among these primary renal cells, proximal tubular cells play important roles in kidney functions [210, 211]. The main roles of proximal tubular cells are reabsorption of proteins and electrolytes, production of erythropoietin, and hydrolase activity. Proximal tubular cells occupy the highest percentage of renal cell population in normal kidney; thus, isolation and expansion of functional primary proximal tubular cells from renal tissues can be considered as an attractive renal cell source for cell-based kidney regeneration [212]. Primary proximal tubular cell cultures have several advantages and are more representative of normal physiology of proximal tubular cells than immortalized cell lines, but primary renal cells, including proximal tubular cells, can lose expression of specific genes during in vitro culture and are limited to only two to five passages [213]. The optimal combination of high purity, well differentiation, and consistent proliferation is observed at passage 2–3 [214].
Culture method of primary kidney cells from human kidney tissues for cell expansion has been established [35]. In histological analyses, the majority of the cultured cells retained a proximal tubular phenotype, while distal tubular cells and podocytes were present in a lower percentage of the entire cell population. In addition, when the expanded cells were cultured under a three-dimensional environment, tubule-like structures with functional properties were formed from the cultured cells. These results indicated that the established cell harvesting and culture method may potentially be developed as an effective cell-based approach for kidney regeneration.
Several protocols for other kidney cell types, except proximal tubular cells, also have been established. Presnell et al. established a culture method for primary cell cultures isolated from all major compartments of the kidney, especially the tubular cell-enriched subpopulation and the erythropoietin-producing subpopulation that were reproducibly developed from both normal and diseased kidneys [215]. Recent advances of immunomagnetic cell isolation technique enabled to isolate additional subtypes of kidney-derived epithelial cells. Renal epithelial cells were successfully separated from the ascending limb and the distal tubule of the human kidney using glycoprotein-coated magnetic beads [216]. This result demonstrated that ascending limb of Henle’s loop and distal tubule can be a promising cell source for cell-based kidney regeneration and establishment of in vitro cell culture system for various cell types of the kidney can be realized.
Renal Stem/Progenitor Cells
Stem/progenitor cells isolated from many adult organs demonstrate clonogenic, self-renewing ability and can give rise to terminally differentiated cells of the original tissue. Renal stem/progenitor population disappears in the adult kidney, possibly due to the loss of its niche [209, 217]. However, renal stem/progenitor cells still exist in the adult kidney and are located in specific locations, such as renal papilla, tubular epithelial cells, Bowman’s capsule, and the S3 segment of the proximal tubules [24, 28–30, 32].
A recent study showed that renal stem/progenitor cells derived from the S3 segment of adult rat kidney nephrons were able to reconstitute a three-dimensional kidney-like structure in vitro [218]. In this study, kidney-like structures were formed when a cluster of renal stem/progenitor cells was suspended in an extracellular matrix gel and cultured in the presence of several growth factors, such as glial cell-derived neurotrophic factor (GDNF), basic fibroblast growth factor (bFGF), HGF, EGF, and BMP-7. The clusters from dissociated S3 segment-derived cells were induced by the hanging drop method in three-dimensional culture, while two-dimensional culture conditions were not able to reconstruct kidney-like structures. The reconstructed kidney-like structures included most of renal substructures, such as glomeruli, proximal and distal tubules, loop of Henle, and collecting ducts, but not the vasculature. This study suggested that a cluster of tissue stem/progenitor cells may have the ability to reconstitute the minimum unit of its original organ by differentiating into specialized cells in the correct niche. Renal stem/progenitor cells derived from the S3 segment of adult rat kidney expressed stem cell markers, such as Sca-1, c-kit, Musashi-1, and nestin, as well as renal lineage markers, such as WT-1 and PAX-2. Although it was assumed that these cells were similar to metanephric mesenchymal cells, based on marker protein expression, the cluster could differentiate into collecting duct-like cells or mesangial-like cells, which are not considered to be derived from metanephric mesenchyme.
Resident mesenchymal stem cells have been described in many organs as a multipotent population adjacent to endothelial cells in the microvasculature and characterized by expression of PDGF, neuron glial antigen-2, CD146, and coexpression of other mesenchymal markers [53]. Isolation of resident mesenchymal stem cells from the murine kidney was also reported [54]. Subsequently, mesenchymal stem cells were isolated from human glomeruli and showed self-renewal capability, clonogenicity, and multipotency [219]. Furthermore, Wang et al. reported that renal mesenchymal stem cells isolated from adult murine kidney could differentiate into renin-producing cells [220]. The comparative study of gene expression in mesenchymal stem cells between kidney and bone marrow origin identified selected patterns of genes in renal mesenchymal stem cells possibly related to a memory of tissue origin [60]. This result suggests that renal mesenchymal stem cells might display organ-specific regenerative capacities that could overcome those of mesenchymal stem cells from other organs, such as the bone marrow or adipose tissue, and that could be exploited for therapeutic applications.
Although adult renal stem/progenitor cells still remain poorly understood, they have been reported to be nontumorigenic when injected into a mouse model, whereas pluripotent stem cells, such as embryonic stem cells or iPSCs, have a risk of potential tumorigenicity as a clinical source [221, 222]. In this regard, the results of aforementioned studies support the possibility that adult renal stem/progenitor cells may represent a safer clinical source than pluripotent stem cells. Therefore, renal stem/progenitor cells may be a promising cell source for kidney regeneration, if it is possible to establish renal stem/progenitor cells with multipotency for kidney reconstruction.
7.4.3.2 Mesenchymal Stem Cells
Mesenchymal stem cells are stromal cells that can be found and isolated from various tissues. They also have the capacity to differentiate into a mesenchymal tissue types, including bone, cartilage, muscle, tendon, fat, and other connective tissues. Unlike pluripotent stem cells, such as embryonic stem cells and iPSCs, mesenchymal stem cells can be practically applied for cell-based therapies with fewer safety concerns and ethical issues. These mesenchymal stem cells have been studied in recent decades as a therapeutic agent for various tissues and organs due to its relatively easy approach to harvest, multilineage differentiation potential, ability to migrate to the site of injury, and no ethical concerns.
Bone Marrow-Derived Stem Cells
Since 2000, bone marrow-derived stem cells have been used in experimental kidney disease models. Several studies have shown that treatment with bone marrow-derived stem cells can ameliorate some of injured renal tissues, such as tubular epithelial cells, podocytes, mesangial cells, and endothelial cells [15–17, 125, 127, 223–226]. Treatment using bone marrow-derived stem cells can contribute to the attenuation of renal fibrosis during progression of chronic kidney disease [138]. Although early studies suggested that bone marrow-derived stem cells could be differentiated into renal epithelial cells and mesangial cells [227–229], recent researches have supported that migration of bone marrow-derived stem cells into the kidney is very rare and their ability to direct differentiation is limited [47]. Thus, the beneficial effects of bone marrow-derived stem cells are considered to be mainly mediated by paracrine action of injected cells that have antiapoptotic, immunomodulatory, and proangiogenic effects [50, 51, 230]. Taking ischemic renal injury, for example, bone marrow-derived stem cells can initially ameliorate the injury either by direct inhibition of cell apoptosis or prevention of inflammatory cell influx. During the repair phase, bone marrow-derived stem cells secrete several factors that promote tubular epithelial cell dedifferentiation and proliferation [231]. The transdifferentiation of bone marrow-derived stem cells observed in early studies may reflect cell fusion. If bone marrow-derived stem cells fuse with resident cells in the kidney, they acquire a phenotype of resident cells. Therefore, it could appear as if bone marrow-derived stem cells were differentiated into resident cells. Indeed, it was reported that bone marrow-derived stem cells could fuse with other cell types [232, 233].
Togel et al. performed the first phase I clinical study to evaluate the efficacy and safety of allogeneic bone marrow-derived stem cell administration for the prevention of acute kidney injury after open heart surgery [234]. Sixteen patients who underwent cardiac surgery and who were at a high risk of postoperative acute renal failure due to underlying chronic kidney disease, advanced age, congestive heart failure, and diabetes mellitus were enrolled in this study. Allogeneic bone marrow-derived stem cells were injected into the suprarenal aorta after surgery. Preliminary data showed that renal function was well preserved after surgery for up to 16 months and none of the patients who received stem cell injection required hemodialysis, while 20% of case controls developed acute renal failure. The length of hospital stay and readmission rates of stem cell group reduced by 40% compared to control group, and no adverse events related to this therapy occurred.
Recently, two subsequent clinical trials were performed to evaluate the effect of bone marrow-derived stem cells in patients who underwent kidney transplantation [235, 236]. These clinical trials have shown that bone marrow-derived stem cells were safe and offered an early recovery of graft function. However, early posttransplant stem cell infusion was found to be possibly associated with acute graft dysfunction due to engraftment syndrome [235]. This adverse effect, which was accompanied by neutrophil recruitment and complement C3 deposition, was possibly attributable to the inflammatory condition occurring after kidney transplantation, leading to altered functions of mesenchymal stem cells [237].
More recently, a phase I clinical study using autologous bone marrow-derived mesenchymal stem cells for the treatment of allograft rejection after kidney transplantation from living donors was performed [238]. In this trial, bone marrow-derived stem cells were administered intravenously at intervals of 7 days when a protocol of renal biopsy at 1 or 6 months showed signs of rejection and/or an increase in interstitial fibrosis or tubular atrophy. Although only six patients were enrolled in this study, two renal biopsies after treatment with bone marrow-derived stem cells showed resolution of cell infiltrates, suggesting a regenerative and anti-inflammatory effect of the cell therapy on the renal tissue. The engraftment syndrome, one of the negative effects of mesenchymal stem cell administration, was not observed in this study possibly due to a late timing of stem cell administration, beyond 6 months, after transplantation.
Despite these encouraging progresses of these clinical trials, questions related to the establishment of the best way to apply mesenchymal stem cells to kidney disease still remain unsolved. In addition, therapeutic mechanism is also still poorly understood. Mesenchymal stem cells do not engraft in the kidney when injected intra-arterially or intravenously; rather, they can form emboli in the lung [239]. Thus, these studies are not cell transplantation but cell infusion that exerts effects through paracrine mechanisms. This implies that if we can identify the bioactive substances secreted by bone marrow-derived stem cells, infusion of these substances without cells would be enough. Indeed, conditioned media from mesenchymal stem cells protected kidneys from acute injury as well as injection of the cells did in preclinical study [49]. Incomplete understanding of the mechanism of mesenchymal stem cells for cell therapy is not a reason not to pursue these clinical trials, but more basic research to investigate the biology of mesenchymal stem cells for kidney protection and renal regeneration as well as further clinical trials devoted to testing efficacy of this therapy is required.
Adipose Tissue-Derived Stem Cells
Adipose tissue-derived stem cells are another type of mesenchymal stem cells residing in subcutaneous adipose tissues. They are an attractive source of cells with regenerative properties that are similar to those of bone marrow-derived stem cells. Moreover, adipose tissue-derived stem cells are promising stem cells for clinical use, because the subcutaneous adipose tissues are abundant in humans and can be easily harvested using liposuction. In addition, these cells have no ethical issues regarding the cell source and less concern about safety of allo- and xenografting. Furthermore, adipose tissue-derived stem cells have more potential of anti-inflammatory and immunomodulatory functions than bone marrow-derived stem cells. Adipose tissue-derived stem cells have the capacity to differentiate into other cell types, such as adipocytes, osteoblasts, neurons, and myocytes [145]. Several studies have demonstrated that cell therapy using adipose tissue-derived stem cells minimized kidney damage or improved renal dysfunction after renal damages, such as ischemic injury, progressive renal fibrosis in a mouse model, cisplatin- or folic acid-induced acute kidney injury, atherosclerotic renal artery stenosis in swine models, and antiglomerular basement membrane disease in a rat model [140–143, 145, 240–242]. Adipose tissue-derived stem cells also seem to improve renal function mainly via paracrine effects, such as suppressing oxidative stress and inflammatory response.
Umbilical Cord Blood-Derived Stem Cells
The umbilical cord blood has been known to contain mesenchymal stem cells, and several studies evaluated the efficacy of administration of umbilical cord blood-derived stem cells in the restoration of renal function in animal acute kidney injury models [243, 244]. Injection of human umbilical cord blood-derived mesenchymal stem cells to immunodeficient mice with cisplatin-induced acute kidney injury was performed [243]. This study showed that umbilical cord blood-derived stem cells ameliorated tubular injury, resulting in the recovery of renal function. Coculturing of umbilical cord blood-derived stem cells with cisplatin-treated proximal tubular cells showed that the expression of HGF was particularly induced and that of interleukin 1-β and tumor necrosis factor-α was significantly decreased in the coculture system. These results indicated that the process of renal function improvement by umbilical cord blood-derived stem cells was also correlated to the modulation of paracrine factors in the kidney. Both umbilical cord blood- and bone marrow-derived stem cells expressed similar set of genes in the comparative study of gene expression profile between these two kinds of stem cells, but bone marrow-derived stem cells predominantly expressed a set of genes related to antimicrobial activity and osteogenesis, whereas umbilical cord blood-derived stem cells predominantly expressed genes related to matrix remodeling and angiogenesis [245]. This result suggested that umbilical cord blood- and bone marrow-derived stem cells may have distinct activities in vivo.
Amniotic Fluid Stem Cells
As multipotent stem cells at the fetal stage, amniotic fluid-derived stem cells have been considered an attractive cell source for regenerative medicine. Amniotic fluid-derived stem cells exhibit characteristics of both embryonic and adult stem cells, are easily harvested, and retain high self-renewal potential and multiple differentiation capacity without development of teratoma formation compared to embryonic stem cells or iPSCs [246]. Perin et al. isolated amniotic fluid-derived stem cells from human amniotic fluid obtained at 12–18 weeks gestation and showed that these cells expanded in vitro could survive, proliferated, and integrated into the embryonic kidney and underwent organ development, demonstrating a potential cell source for kidney regeneration [247]. Therapeutic effects of human amniotic fluid-derived stem cells have been demonstrated in kidney injury animal models, such as glycerol- or cisplatin-induced acute kidney injury, a mouse model of unilateral ureteral obstruction, and Alport syndrome [146, 248–251]. Initial studies into the properties of amniotic fluid-derived stem cells in kidney injury demonstrated that the injection of such cells into animal models of kidney disease delayed progression of fibrosis [250]. More recent studies have investigated whether amniotic fluid-derived stem cells can generate renal cell types in vitro, with evidence for a capacity to differentiate into podocyte-like cells [252]. In addition, it was proposed that these cells could be propagated in a nephron progenitor state for many passages in order to provide an expandable source of cells to give rise to this specific renal cell type. Comparison of the characteristics between amniotic fluid- and bone marrow-derived stem cells was performed [248]. In this study, amniotic fluid-derived stem cells had a more potent antiapoptotic activity against renal tubular cells but lesser stimulatory activity for the proliferation of renal tubular cells compared with bone marrow-derived stem cells. It was also demonstrated that amniotic fluid- and bone marrow-derived stem cells expressed different sets of paracrine factors, indicating that both cell populations may have distinct activities in vivo. In another study, mammalian target of rapamycin was reported as an important factor in renal differentiation of amniotic fluid-derived stem cells [253].
Endothelial Progenitor Cells
Endothelial progenitor cells participate in the repair of tissues, including the kidney, under specific physiological and pathological conditions. Renal ischemia mobilized endothelial progenitor cells, and transplantation of endothelial progenitor cell-enriched cells from the medullopapillary parenchyma provided partial renoprotection after ischemic injury of the kidney [254]. Acute elevation of uric acid acts as an endogenous mediator of mobilization and renoprotection of endothelial progenitor cells [255]. Endothelial progenitor cells can be isolated from human peripheral blood using CD34 as a marker for positive selection [256]. The CD34+ mononuclear blood cells obtained the characteristics of vascular endothelial cells when cultured on fibronectin-coated dishes. Endothelial progenitor cells were incorporated in ischemic tissues in vivo and expressed vascular endothelial cell markers, such as CD31, when introduced into the circulation using a hindlimb ischemia model. The efficacy of administration of endothelial progenitor cells for the improvement of renal function was also reported in animal models of acute kidney injury and renal artery stenosis [254, 257, 258]. In a chronic renal artery stenosis model, a single intrarenal injection of autologous endothelial progenitor cells preserved microvascular architecture and decreased microvascular remodeling by preserving hemodynamics [257]. However, it was reported that the function of endothelial progenitor cells was deteriorated in chronic kidney disease patients, suggesting that the transplantation of autologous endothelial progenitor cells may not be feasible for the treatment of chronic kidney disease [259].
7.4.3.3 Pluripotent Stem Cell
Pluripotent stem cells have the potential to differentiate into any cell type in the body and self-assemble into heterogeneous tissues or organs, and they have indeed been shown to generate mature cells in vitro [260–262]. Pluripotent stem cells include both embryonic stem cells, which are derived from embryos and grown in primary culture, and iPSCs, which are produced from terminally differentiated cells using transfection factors, such as c-myc, Oct4, Klf4, and Sox-2. Pluripotent stem cells have been successfully differentiated into various types of cells and tissues, including hematologic, neural, cardiac, hepatic, pancreatic, and intestinal lineages [263–271]. Recent progress toward generating renal cell types from embryonic stem cells or iPSCs has generated human nephron progenitor cells, including intermediate mesoderm and metanephric mesenchyme cells [272–277]. However, the progress of kidney regeneration using pluripotent stem cells is notably slow compared to other fields, and there have been few efficient protocols in the kidney, whereas full protocols for the derivation of neurons, hepatocytes, and cardiac myocytes were already established [278–280]. Although different studies have used different growth factor protocols in human pluripotent stem cells, the Wnt agonist CHIR99021 was commonly used to promote mesoderm differentiation [273].
Recently, an efficient method has been developed to induce the differentiation of intermediate mesodermal cells from pluripotent stem cells using a combination of activin A and CHIR99021, followed by combined treatment with BMP-7 and CHIR99021 [272, 274]. Odd-skipped related 1 (OSR1)+ intermediate mesodermal cells were differentiated from human iPSCs using this protocol with an efficiency greater than 90% [274]. Several studies also have endeavored to generate metanephric nephron progenitor cells by direct differentiation from pluripotent stem cells. Takasato et al. induced a primitive streak from human embryonic stem cells using activin A (or CHIR99021) and BMP-4 [275]. The differentiated human embryonic stem cells formed renal vesicles combined with dissociated embryonic mouse kidney cells, involving the integration of human cells into mouse renal structures. In contrast, Taguchi et al. demonstrated more reasonable protocols to induce renal structures such as nephrons and proximal tubules [277]. They proposed the concept of “posteriorization” to induce nephron progenitor cells from pluripotent stem cells in a mouse and human model, indicating that nephron progenitor cells of the metanephric mesenchyme could be derived from posteriorly located intermediate mesoderm.
The ureteric bud lineage cells were also attempted to be differentiated from pluripotent stem cells by stepwise treatment of iPSCs or human embryonic stem cells with a combination of fibroblast growth factor 2 and BMP-4 for 2 days, followed by combined treatment with activin A and BMP-2 for another 2 days [276]. After the 4 days of treatment, intermediate mesoderm-like cells expressing PAX2, OSR1, WT1, and LHX1 were produced, and the ureteric bud markers, such as HOXB7, RET, and GFRA1, were upregulated in these cells after additional 2 days of differentiation, indicating that ureteric bud progenitor-like cells could be differentiated from pluripotent cells.
Embryonic Stem Cell
Embryonic stem cells are pluripotent stem cells and were initially derived from the inner cell mass of the blastocyst of mouse embryos [281]. These cells have the ability to differentiate into various cell types of the mesodermal, endodermal, and ectodermal lineages; thus, they have been considered to be used as an effective tool for kidney regeneration. Although research on human embryonic stem cells is limited due to ethical concerns, extensive research was conducted using murine embryonic stem cells to establish the methods to efficiently induce differentiation into renal cells [282]. Thomson et al. first established human embryonic stem cell line in 1998 [283], and subsequently human embryonic stem cell lines have been found to be capable of differentiating in vitro into extraembryonic and somatic cell lineages [284]. When human embryonic stem cells were cultured with a mixture of growth factors, including bFGF, transforming growth factor (TGF) β1, activin-A, BMP-4, HGF, EGF, β-nerve growth factor, and retinoic acid, they differentiated into cells expressing WT-1 and renin [285]. Moreover, it was shown that mouse embryonic stem cells stably transfected with Wnt4 differentiated into tubular-like structures that express aquaporin-2 when cultured with combination of HGF and activin-A [286]. The mixture of LY294002, CCG1423, and Janus-associated tyrosine kinase inhibitor 1 was also shown to enhance the differentiation of mouse embryonic stem cells into intermediate mesoderm and renal progenitor cells [287]. In another study investigating an ex vivo culture system, embryonic stem cells were microinjected into the developing metanephros, and this was cultured to analyze the differentiation capacity of embryonic stem cells into renal cells [288]. Tubule-like renal epithelial structures were identified with an efficiency of about 50%, and individual embryonic stem cells were occasionally observed in structures resembling glomerular tufts. Furthermore, when embryonic stem cells treated with retinoic acid, activin A, and BMP-7 were injected into a developing metanephros, they contributed to the tubular epithelia with almost 100% efficiency [289]. Despite these promising results, there is a concern about this technique that embryonic stem cells can develop teratoma at 2 to 4 weeks after transplantation into mouse [290]. In addition, the risk of uncontrolled growth as well as legal and ethical problems associated with the use of embryonic tissue is limitation of the embryonic stem cells. Although embryonic stem cells are a valuable cell source for investigating the mechanism of kidney regeneration, there are still several hurdles for clinical applications of these cells.
Induced Pluripotent Stem Cell
Another cell source that possesses pluripotency is the iPSC, which was first generated by Takahashi et al. through reprogramming human fibroblasts in 2006 [261]. These novel discoveries have completely changed view of the development and cellular specialization. After then, iPSCs have been successfully established from several mammalian species, including rat, rabbit, pig, monkey, and humans [262, 291–296]. Nevertheless, not all adult cells can be similarly reprogrammed, indicating that critical factors for reprogramming are cell dependent. For example, reprogramming of mature B cells from adult spleen to iPSCs required an additional factor, C/EBPα, as well as Oct4, Sox2, Klf4, and c-Myc [20]. Recently, generation of iPSCs has been reported from human mesangial cells, tubular cells, and epithelial cells derived from urine [147, 149, 297]. The iPSCs derived from renal tissues may have advantages for renal cell therapy or kidney regeneration, because a persistent genome-wide epigenetic memory of the somatic cell of origin can be retained in iPSCs [298]. Indeed, when iPSCs derived from human mesangial cells that were differentiated in podocyte were engrafted in a metanephric kidney, they were integrated in the developing glomeruli [147, 148]. In addition, there are several advantages of iPSCs for kidney regeneration, such as no ethical issues and no immune rejection especially compared to embryonic stem cells. However, increased risk of uncontrolled growth is one of the great concerns, because these cells are reprogrammed using Klf4 and c-Myc, which are oncogenic factors [261, 299]. Hopefully, iPSCs can be established from human renal proximal tubular cells without transfection of Klf4 and c-Myc, suggesting that the oncogenic risk associated with iPSC generation can be decreased by expressing only Oct4 and Sox2 [299]. Therefore, it is possible to prepare patient-specific pluripotent cells without manipulating germ cells because iPSCs are pluripotent and can be generated from adult somatic cells.
Recent study reported the therapeutic effect of iPSCs on renal ischemia [151]. In this study, iPSCs were generated without transfection of c-Myc and administered via intrarenal arterial route into kidneys of ischemia/reperfusion-induced acute kidney injury rat models. The administration of iPSCs reduced the expression of oxidative substances, proinflammatory cytokines, and apoptotic factors and eventually improved survivals of rats.
Nevertheless, there are still several hurdles of iPSCs-based therapy, such as no established differentiation protocols for moving from pluripotent state to functional renal cells, undefined optimal final culture conditions for target cell, and multiple steps each requiring different factors to induce a stepwise differentiation [41]. In addition, it was reported that some cells differentiated from iPSCs expressed abnormal gene and induced T-cell-dependent immune response even in syngeneic recipients [300]. Therefore, it is necessary to evaluate the immunogenicity of specific cells derived from iPSCs before they can be used in a clinical application.
7.4.4 In Vitro Kidney Regeneration Without Scaffolds
There have been many efforts to generate mature cells using pluripotent stem cells in vitro. Several studies reported successful generation of mature cells, which have characteristics of liver, pancreas, and intestine, from embryonic stem cells or iPSCs using stepwise protocols mimicking the mechanism of embryonic development [263, 265, 267, 270, 271]. Furthermore, autonomous formation of three-dimensional adenohypophysis, polarized cortical tissues, optic cap, and retina structures using three-dimensional culture systems of embryonic stem cells suggested the possibility of organogenesis for therapeutic regeneration using this approach [301–304].
It was demonstrated that a single multipotent progenitor cell from embryonic mouse kidney can express Sall-1 and differentiate into several types of renal cells, such as podocytes and tubular epithelial cells, and eventually reconstruct a three-dimensional kidney structure [305]. Another study also reported that single-cell suspensions from embryonic kidney reaggregated and formed organotypic renal structures [306]. Because intermediate mesoderm cells differentiated into renal progenitor cells and then into maturing renal cells, if pluripotent stem cells can be differentiated into intermediate mesoderm, all kinds of renal cells may be generated from embryonic stem cells or iPSCs. The protocols to differentiate human iPSCs into intermediate mesoderm cells were established using bacterial artificial chromosome-based vectors and single-nucleotide polymorphism array-based detection [274]. These cells expressed intermediated mesoderm marker genes and could mature into various types of cells including those found in organs, such as the kidney, adrenal gland, and gonad. These results suggested that three-dimensional kidney structure could be constructed if pluripotent stem cells can be made to generate renal progenitor cells.
7.4.5 Blastocyst Complementation
Blastocyst is an early developmental stage of the embryo that occurs 5 days after fertilization. Injection of pluripotent stem cells into blastocysts synchronizes the development of two line cells, and the combined blastocyst can generate a chimeric body. The chimera can also be produced by injecting pluripotent stem cells into xenoblastocyst, which lacks potential to form any particular cell lineage. The chimera is then transplanted into a foster uterus, and the deficient cells are exclusively derived from injected pluripotent stem cells. It was first reported that injection of normal embryonic stem cells into the blastocysts of recombination-activating gene 2-deficient mice, which have no mature B or T lymphocytes, generated somatic chimeras with embryonic stem cell-derived mature B and T cells [307]. Recent several studies demonstrated that several types of tissues and organs, such as the thymic epithelium, yolk sac, germ cells, hepatocytes, heart, pancreas, and kidney, could be reconstructed using this blastocyst complementation system [308–315]. Kobayashi et al. successfully generated a functional pancreas using this approach [310]. In this study, iPSCs of rat were injected into Pdx-/- (pancreatogenesis-disabled) blastocysts of mouse that produced newborn rat/mouse chimeras with a pancreas which originated almost entirely from the injected iPSCs of rat. The mouse and rat iPSC-derived pancreas produced insulin, and the transplantation of this iPSC-derived pancreas islets improved hyperglycemia in a diabetic rodent model [217]. This study suggested that iPSC-derived cellular progeny could aggregate and differentiate into the deficient organ in a vacant developmental niche. Moreover, these results also demonstrated that interspecific blastocyst complementation could be used to generate organs derived from donor pluripotent stem cells in vivo using a xenogeneic environment. This study group generated a Pdx-/- pig and succeeded in generating bigger pancreas using this technique, suggesting that human-scale organs could theoretically be generated [311]. Likewise, chimeric mice carrying a liver derived from injected iPSCs of mouse were also generated from blastocysts with fumarylacetoacetate hydrolase deficiency [308]. Hepatocytes derived from the injected iPSCs also had a proliferative characteristic of normal hepatocytes.
Kidney reconstruction using this blastocyst complementation system has been attempted. Usui et al. injected normal mouse iPSCs into blastocysts from kidney-deficient mice lacking the SAL-like 1 protein, which is essential for kidney development; eventually, the neonatal mice had kidneys derived from injected iPSCs [315]. However, the vascular and nervous systems were not constructed from iPSC-originated cells; thus, the kidney was not completely complemented. Immunohistochemical analysis of the regenerated kidney showed that the vascular system, including renal segmental, lobar, interlobar, arcuate, and interlobular arterioles, was a chimeric structure originating from both donor iPSCs and host cells. Whether urine was produced by this regenerated kidney was not certain due to the lack of precise urine analysis. In addition, generation of rat kidneys in mice failed after injection of rat iPSCs into kidney-deficient mouse blastocysts. This result indicates that the key molecules in mice involved in interactions between the metanephric mesenchyme and ureteric bud do not cross-react with those in rats. Therefore, the generation of xenogeneic organs using interspecific blastocysts requires a host animal strain lacking all renal lineages.
Although this strategy using blastocyst complementation has showed promising results for kidney regeneration, there are several limitations for clinical applications. It is difficult to generate interspecific chimeras in livestock. In addition, tissue rejection also should be overcome. Moreover, ethical issue is one of the most serious problems. There has been always a concern about the possibility of generating interspecific chimeras containing brain derived from injected pluripotent stem cells.
7.4.6 Embryonic Organ Transplantation
The embryonic metanephros (Fig. 7.3), which constitutes the primordial mammalian kidney, has been used for the investigation of kidney regeneration and showed promising results as a potential source for the generation of a functional whole kidney [156, 316–324]. If a metanephros is transplanted into the renal cortex of a host mouse, it can continue to grow [155]. The transplanted metanephros contains vascularized glomeruli and mature proximal tubules endowed with a capacity for glomerular filtration. Collecting duct-like structures also appeared and extended from the transplant toward the papilla of the host. Although there is no direct evidence that these collecting duct-like structures connect with the collecting system of the host or that the transplanted metanephros functions in a manner similar to that of native kidney, these results offer a rationale for the existence of renal stem cells in the metanephros from embryos, which can be a potential source of transplantable regenerated kidney. However, there are still several unsolved problems related to this technique, including questions as to the suitability of the renal capsule of patients with dialysis as a transplant site, given the significant disruptions to this area, such as the vasculature, and the fact that space limitations beneath the renal capsule may inhibit the growth of transplanted metanephros. To overcome these concerns, the metanephroi were transplanted into a host omentum in another study [156]. The transplanted metanephroi into the omentum were not confined by a tight organ capsule or disturbed by dialysis. The metanephroi transplanted into a host omentum also could differentiate into mature vascularized glomeruli and proximal tubules and produce urine. Moreover, after an intact ureteroureterostomy with the ureter of the native kidney that was removed, anephric host animals started to void and showed prolonged survival. These metanephroi developed in host animals exhibit metabolic renal function and produce erythropoietin and renin leading to detectable levels of these proteins in serum [319, 323, 324]. Furthermore, metanephroi from porcine embryos were transplanted into either the omentum of mice in which costimulation was blocked or area under the kidney capsules of immunodeficient mice and developed to generate fully functional nephrons [316, 320]. The average levels of creatinine and urea nitrogen were higher in the cystic fluid that arose from the transplanted tissue than in the sera of the transplanted host mice. These successful xenotransplantation of metanephroi between different species was based on previous studies showing minimal immunogenicity in tissues, including the metanephros harvested at earlier gestational ages [325]. In cases of allotransplantation, such as rat metanephros to rat omentum, transplants assumed a kidney-like shape in situ that was approximately one-third the diameter of the native kidney, and the transplants contained well-differentiated kidney structure in histological analysis. In addition, the transplantation can be performed without immunosuppression. However, in xenotransplantation, although transplanted metanephros grew and differentiated into renal tissue in the host omentum, immunosuppressants were required. Without these agents, the transplants disappeared soon after transplantation.


Fig. 7.3
Metanephroi of E13 mouse embryo. (a) Metanephroi, which are located just above aortic bifurcation, can be observed in E13 mouse embryo after removing lower half of the spinal cord and dorsal muscular layer of the embryo (red arrow, both metanephroi). (b) Intact metanephros can be isolated using microforceps in the vision of microscopy
Transplantation of metanephros was also shown to maintain blood pressure in anephric rats with induced acute hypotension and reduce vascular calcification in rats with chronic renal failure, indicating that transplanted metanephroi have multiple renal functions as well as urine production [323, 324]. Although the omentum has been preferred because a tight renal capsule does not confine it and the host vessels can be preferentially integrated into the transplanted metanephros, the low hydrostatic pressure in the omentum may influence the acquisition of some renal function in the developed metanephros. Indeed, it was reported that the para-aortic area is better for the establishment of renin-producing tissue [319].
Recently, it was reported that xenotransplanted metanephros could supply endogenous mesenchymal stem cells with a niche for differentiation into erythropoietin-producing renal tissues [318]. Polymerase chain reaction (PCR) using species-specific primers and sequence analysis showed that xenotransplanted metanephroi expressed host animal-originated erythropoietin. This result implied that the erythropoietin-producing cells were originated from the host animals and developed to produce erythropoietin in the transplanted metanephros. It was also shown that the erythropoietin-producing cells were not originated from integrating vessels but from circulating host mesenchymal stem cells mobilized from the bone marrow.
Conventional transplantation of metanephros requires strong and persistent immunosuppression to avoid humoral rejection associated with the xenogeneic barrier, which can cause several adverse effects, such as carcinogenicity and severe rejection. Thus, for safety, when the xenotransplant is not needed anymore, it should be discarded by introducing a cell fate-regulating system in which a suicide gene is expressed on demand. To avoid the xenogeneic barrier, metanephroi isolated from transgenic ER-E2F1 mice that are expressing E2F1 under the control of tamoxifen can be used. E2F1 is a transcription factor which regulates cell proliferation and ectopic expression of which induces apoptosis. The administration of tamoxifen in host rats wherein metanephroi of ER-E2Fa mice were transplanted activated the suicide gene, and the xenocompartments of transplanted metanephroi were ablated by apoptosis, leaving the autologous erythropoietin-producing tissues. In addition, it was also confirmed that the metanephros established by this method no longer needed immunosuppressants while maintaining erythropoietin production [318]. These results indicate that xenometanephroi per se could acquire some renal functions in the host omentum as well as supply a niche for host stem cells to regenerate renal tissues and then be rebuilt to constitute only host cell components. This technique can be helpful to reduce the adverse effects of long-term administration of immunosuppressants and to moderate the ethical issues surrounding xenotransplantation.
7.4.7 Renal Tissue Regeneration Using Metanephric Progenitor Cells
One of the most promising methods for kidney engineering is the generation of fetal or mature renal tissues using intrinsic proficiency of cells to organize themselves into fully self-derived sophisticated structures [301, 303]. Several early studies using this strategy demonstrated that renal epithelial cell lines cultured in extracellular matrix gels could be induced to create branching tubules either by the whole embryonic kidney or by the metanephric mesenchyme [326]. Advancement of this methodology showed that cells isolated from ureteric bud (Fig. 7.4) of mice at embryonic day 11.5 (E11.5) and propagated in vitro could undergo branching tubulogenesis when stimulated by a conditioned medium from a metanephric mesenchyme-derived cell line [327]. Similarly, a tubular epithelial cell line or mesenchymal stem cells generated glomerular and tubular structures in subcutaneous spaces when stimulated by a media from vascular endothelial and tubular cells [328]. These results suggested that simple cultures of cells derived from undifferentiated metanephric mesenchyme and unbranched ureteric bud could be used to create branching tubular structures similar to those that form in normal metanephros.


Fig. 7.4
Mechanically isolated ureteric bud from the metanephros of E11.5 mouse embryo
Generation of three-dimensional renal epithelia which contain glomerular-like structures in vitro was attempted using suspensions of murine metanephric mesenchymal E11.5 progenitor cells selected for a high expression of Sall-1, which is essential during kidney development [305]. This cell preparation required coculture with an exogenous spinal cord cell layer, as has been performed in traditional developmental investigations [329]. Although the functional capacity of these structures has not been investigated in vivo, this approach suggested that dispersed cell suspensions can be used as starting material to obtain rudimental three-dimensional kidney tissues. Further development of this method enabled the generation of fetal kidney tissue from suspensions of E11.5 kidney cells without using any exogenous tissue [306]. While this method successfully reproduced the structures and differentiation states of nephrons and stroma, collecting ducts developed as a multitude of very small collecting duct trees rather than a continuous one as would happen in a normal kidney. Combination of reaggregated single-cell suspensions of ureteric bud and metanephric mesenchyme allowed the formation of nephrons arranged around a single collecting duct tree [330]. However, this endeavor did not achieve development of glomeruli to any meaningful extent due to avascular environment in vitro. This limitation inhibits further progress of research related to maturation potential of tissue obtained from a simple culture or suspension of precursor cells. Therefore, the capacity of these cells to generate nephros with functional properties still remained unclear.
Using simple suspensions of singe kidney cells, “organoids” that could carry out renal functions when implanted into a living animal, such as glomerular filtration, tubular reabsorption of macromolecules, and production of erythropoietin, were finally constructed [322]. These organoids were generated from single-cell suspensions derived from E11.5 mouse kidneys and then implanted under the renal capsule of an athymic rat. The main procedure to obtain complete glomerulogenesis consisted of soaking the organoids in a solution containing VEGF, and then injection of VEGF into the recipient animals after the organoids had been implanted in the kidney. This method restituted the efficacy of the podocyte VEGF axis that is necessary for glomerular capillary endothelial induction and resulted in the formation of vascularized glomeruli with fully differentiated glomerular capillary walls, including endothelial fenestrae, slit diaphragms, and foot processes. These organoids could develop glomeruli with normal-appearing slits in vivo, wherein the proportion of diaphragms were comparable to that found in the adult glomerulus. When the ultrafiltering function of the intragraft nephrons was tested by injecting fluorescent dextrans of increasingly high molecular weight into the host blood system, the proximal tubular cells concentrated dextrans only of lower molecular weights from the lumen, indicating efficient ultrafiltration.
Approaches starting from the metanephros and dissociating down to the single-cell level offer a valuable source for performing pharmacologic or genetic modifications in individual cells prior to the in vitro aggregation step [322, 331]. The single-cell stage allows the use of genetic engineering approaches to humanize cells, by introducing immunomodulatory genes or by switching off others, to reduce the possibility of rejection and facilitate the xenotransplantation of animal renal tissues [331]. These self-forming organoids can be exploited to generate human renal tissue by constructing chimeric kidneys that combine animal progenitor cells and human stem cells, followed by the selective elimination of animal cells after transplantation. Previous studies have shown that the organoids could incorporate cells of another source, such as human amniotic fluid stem cells or iPSC-derived OSR1-positive cells, to build chimeric tissue in vitro [253, 274]. Although several technical problems remain to be overcome, these systems are useful to examine the mechanisms of renal progenitor differentiation and suggest the possibility of developing a whole kidney from a single stem cell.
7.4.8 Kidney Regeneration Using Xenoembryos
The idea of generating human organs in animals was previously suggested by several studies [332, 333]. Regeneration of a whole functional kidney that produced urine and renal hormones using a developing heterozoic embryo as an organ factory has been endeavored [334–336]. This strategy is based on the concept of “borrowing” the developing program of a growing xenoembryo by applying stem cells at the niche of organogenesis. During development of the metanephros, the metanephric mesenchyme initially forms from the caudal portion of the nephrogenic cord and secretes GDNF, which induces the nearby Wolffian duct to produce a ureteric bud [337]. Then, the metanephric mesenchyme consequently forms the glomerulus, proximal and distal tubule, loop of Henle, and the interstitium, as a result of reciprocal epithelial-mesenchymal induction between the ureteric bud and metanephric mesenchyme [338]. For this epithelial-mesenchymal induction to occur, GDNF must interact with its receptor, c-ret, which is expressed in the Wolffian duct. Thus, it can be hypothesized that GDNF-expressing stem cells can differentiate into kidney structures if they are placed at the budding site and stimulated by several factors spatially and temporally identical to those found in the developmental milieu.
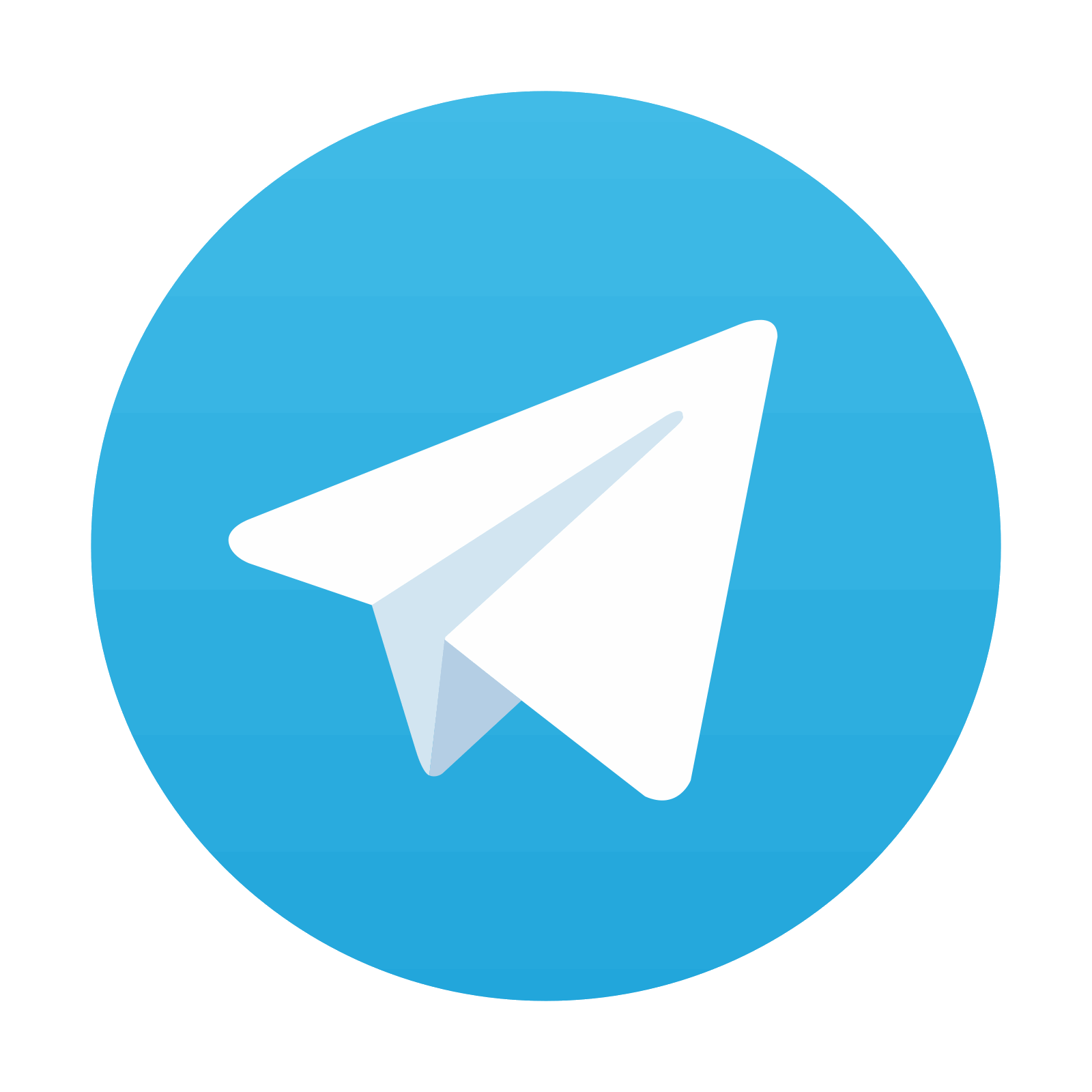
Stay updated, free articles. Join our Telegram channel
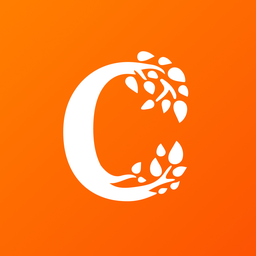
Full access? Get Clinical Tree
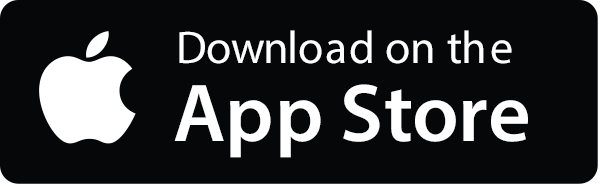
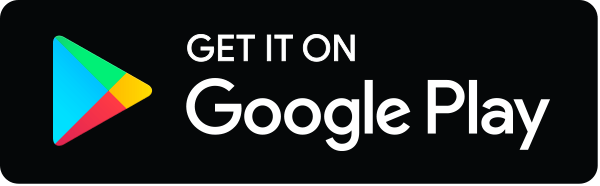