Fig. 4.1
Spinner-flask bioreactor. (a) Schematic design [13]. (b) Human iPSC cultures in a spinner-flask bioreactor and the cultured iPSCs on the Matrigel-coated polystyrene beads and cell aggregations (c) and cell growth and viability during the culture (d) [21]. Reprinting of each image was permitted from publishers
4.2.2 Rotating Wall Vessel (RWV) Bioreactor System
Unlike the spinner flask bioreactor, the rotating wall vessel (RWV) bioreactor is designed to provide low shear stress and a homogeneous dynamic cell culture environment [22] (Fig. 4.2a). The RWV bioreactor system was originally developed by scientists at the National Aeronautics and Space Agency (NASA) for cell culture under low- or no-gravity conditions for targeted use in space. Briefly, the RWV system consists of culture medium that is horizontally rotated while connected to an oxygen and gas supply. The gravitational force experienced by single cells is minimal (approximately 10−2 g), thus reducing damage to cells and promoting cell viability and tissue maturation during in vitro culture. The efficiency of RWV bioreactors for in vitro cell culture has been evaluated through histological, biochemical, and biomechanical analysis of several cell types that have been cultured in RWV bioreactors, including tumor cells [23, 24] and stem cells [25, 26] (Fig. 4.2b–d), and engineered tissue constructs, such as cartilage tissue constructs [27, 28].
4.2.3 Hollow Fiber System
One representative application of hollow fiber bioreactors is used as a hemodialysis membrane (dialyzer) for treating patients with kidney failure [29]. A dialyzer contains a bundle of hollow fibers (≈10,000 fibers), each with an inner diameter of approximately 200 μm. As a patient’s blood is perfused through the fibers, blood waste is removed through the fiber membrane, and the filtered blood is returned to the patient. Ever since the first dialysis membrane was developed by Lipps et al. using cellulose-derived hollow fibers [30], various types of materials have been developed to improve blood dialysis efficiency [31]. Dialysis efficiency primarily depends on the biocompatibility of the hollow fibers, which is influenced by the membrane components and flow properties within the dialyzer. In addition, several fiber parameters, such as diameter, molecular weight cutoff (MWCO), wall thickness, and material type (i.e., cellulose or synthetic materials), have been modified to improve dialysis efficiency [31].
4.2.4 Hollow Fiber-Incorporated Perfusion Bioreactor
Recent progress in cell culture techniques and stem cell research has offered great promise in the field of cell-based therapies, and large-scale production of therapeutic cells is required to address massive needs for clinical translation. To this end, hollow fiber bioreactors have been used to scale up mammalian cell culture [32]. When compared with stirred flask culture, cell culture within the hollow fiber system has several advantages. Hollow fibers provide a large surface area for cell inoculation, and cells can be seeded and cultured within the hollow fibers, thus creating cultures with high cell densities. Appropriate perfusion through the hollow fibers is needed to efficiently provide oxygen and nutrients to seeded cells. During bioreactor culture, shear stress must be maintained at an appropriate level that does not harm the cells in order to preserve cellular viability during long-term cell culture. Hybridoma cell lines have been used to test the feasibility of large-scale antibody production [32], and recent studies have reported in vitro mass production of therapeutic cells [33] and stem cells [34], including embryonic stem cells (ESCs) [35] and mesenchymal stem cells (MSCs) [36, 37]. The hollow fiber system, in particular, has been used as an appropriate environment for stem cell differentiation [34]. Several types of cells, including hematopoietic stem cells (HSCs) [38], ESCs [39], and iPSCs [40], have been efficiently differentiated into blood cells [38], dopamine-producing neurons [39], and hepatocytes [40], respectively.
Another application of the hollow fiber-incorporated perfusion system includes the development of bioartificial organs as a promising strategy for treating organ failures. One example is the use of bioartificial livers to temporarily support liver function. In end-stage liver failure, hemodialysis and liver-assist systems are limited in their ability to remove toxins from the blood and cannot replicate several other functions of the liver. However, liver-assist systems are developing in order to incorporate viable cells and thereby support metabolic function. One early study, performed by Sussman et al., used hollow fiber systems to temporarily support patients with liver failure [ 41 ]. The bioartificial liver developed in this study, known as the extracorporeal liver-assist device (ELAD ® ), was designed to seed immortalized liver cells into the hollow fibers of the system, maintain cell viability, and provide metabolic function to blood perfused throughout the system. After ELAD ® showed promises in numerous preclinical trials [ 42 ], the safety and efficiency of ELAD ® in supporting liver function were confirmed in clinical trials [ 41 ], and the device is currently available for liver failure patients [43] (Fig. 4.3b).
Likewise, kidney-assist devices using viable kidney cells have been developed to address the limitations of blood dialysis in the conventional hemodialysis system [44]. To support renal biological functions that cannot be replicated by conventional blood dialysis, Humes et al. [45] developed a bioartificial kidney device by seeding pig-derived proximal renal cells into a hollow fiber kidney-assist device and implanting the device into dogs with renal failure (Fig. 4.3a). The bioartificial kidney system was able to facilitate improved filtration capability, plasma parameters, and metabolism compared to a hemodialysis system that did not incorporate cells. These results demonstrate that it is possible to develop an artificial kidney by incorporating viable renal cells into the conventional kidney dialysis system and that such a cell-based artificial kidney may contribute to the improvement of renal function in kidney failure patients.
4.3 Application of Bioreactors for the Engineering of Urogenital Constructs
As described previously, bioreactor systems have been applied to the reconstruction of 3D engineered tissues, and numerous types of urogenital constructs, including bladder, urethra, and kidney constructs, have been fabricated in vitro for implantation in vivo. While most early studies did not use bioreactor systems for 3D culture, the need to develop functional large-scale tissue constructs that mimic in vivo environments has necessitated the creation of well-designed bioreactor systems.
4.3.1 Bladder
End-stage bladder disease is frequently treated using grafts of native gastrointestinal segments; however, the incorporation of the graft tissue into the host urinary tract often causes several complications, including metabolic disturbances, increase of mucosa production, and malignant diseases. Alternatively, natural and synthetic biomaterials have been used to address these issues, but several disadvantages, such as foreign body reactions, perforation of adjacent urogenital tissues, severe fibrosis, and poor host tissue integration, still remain unresolved [46]. Cell-based approaches utilizing viable bladder cells have allowed researchers to recreate bladderlike structures in vitro, thus enabling functional bladder reconstruction by in vivo implantation. Numerous studies have reported the creation of bioengineered bladderlike constructs using different types of scaffolds, such as those made from biological [47–51] and synthetic materials [7, 52], and a majority of early studies were conducted in 3D culture systems without in vitro bioreactor systems. In an early study, we developed an engineered bladder tissue construct using dog-derived bladder-derived cells [51]. We used canine-derived allogeneic submucosa as a scaffold and then seeded urogenital and smooth muscle cells into the scaffold for in vitro culture. The implantation of this autologous cell-seeded bladder construct resulted in an approximately 99% increase in bladder urodynamic capacity, while the implantation of cell-free scaffolds increased bladder size by only 30%. Functional and histological analysis demonstrated that the implantation of the cell-seeded construct facilitated normal bladder compliance, and the seeded cells showed normal cellular organization and phenotypic properties along the harvested host tissue. These results demonstrate that a small biopsy (≈1 cm2) is sufficient to obtain enough cells for engineering bladder tissue and that the implantation of cell-seeded tissue constructs produces more bladder augmentation than the implantation of cell-free scaffolds [51].
The most critical challenge in the implantation of cell-seeded constructs is to accelerate host vascularization that is able to provide seeded cells with sufficient nutrients and oxygen for better cell survival within the implant. Schoeller et al. tested whether pre-vascularization of the scaffold matrix would enhance bladder reconstruction [49]. To achieve pre-vascularization, the authors utilized native host regenerative capabilities to fabricate a capsule pouch with host vascularization. Pre-vascularized scaffolds were created by implanting silicone blocks into the groins of rats for 1 week. Autologous urothelial cells were then seeded into these vascularized capsules, and the cell-seeded constructs were transposed into bladder wall defect sites. The group treated with the pre-vascularized cell-seeded scaffolds experienced a higher survival rate than the scaffold-only (80% mortality) and saline groups (100% mortality). Histological analysis of the cell-seeded constructs with prefabricated capsule structures showed multilayered urothelial cell lining along the transplantation site. These results suggest that it is possible to pre-vascularize cell-seeded constructs using the host’s regenerative capacities, which may also contribute to accelerating urogenital tissue regeneration in vivo [49]. Another study utilizing a pre-vascularization strategy for the engineering of bladder constructs was conducted by Schultheiss et al. [47], who seeded autologous urothelial and smooth muscle cells onto scaffolds made of decellularized bowel segments. To provide the constructs with pre-vascularization capabilities, endothelial progenitor cells were also incorporated into the engineered bladder constructs, which underwent 3D in vitro culture before implantation. The implantation study revealed that bladder constructs seeded with endothelial cells efficiently prevented blood thrombosis when compared to a control without pre-vascularization capabilities, indicating that pre-vascularization of an engineered bladderlike construct can be used for the reconstruction of a defective bladder.
More recently, our group successfully developed an approach to create engineered bladder constructs containing autologous bladder cell sources for treating patients with end-stage bladder disease [7]. Autologous urothelial and smooth muscle cells were cultured, seeded, and matured within collagen-based scaffolds for several weeks. The engineered bladder constructs were then implanted into myelomeningocele patients using an omental wrap. In follow-up studies, we found that bladder functions, such as leak point pressures and compliance, were improved in patients who were implanted with our engineered bladder constructs. These results suggest that our bioengineered bladderlike construct can be used to treat patients who need cystoplasty.
Since the bladder tissue repeatedly undergoes dynamic circumstances (such as fill-void cycles) daily, reconstruction of functional bladder tissue in vitro is desirable in order to retain structural stability against mechanical stimulation [53]. To this end, mechanical preconditioning can be applied to the bladder construct to create a functional bladder that has mechanical properties similar to those of bladders in in vivo environments [15, 53] (Fig. 4.4a). Several approaches have been used in order to improve the mechanical properties of engineered bladder constructs. One early study attempted to test the feasibility of simulating normal bladder physiology using a bioreactor system [54]. Wallis et al. developed a bioreactor system that simulated mechanical conditions occurring in normal bladder tissue, thereby allowing cell-seeded scaffolds to undergo cyclic mechanical stimulation. The scaffolds, which were seeded with bladder cells, were based on acellular porcine matrix or commercially available small intestinal submucosa (SIS). Histological analysis demonstrated that the seeded urothelial and smooth muscle cells were evident during bioreactor culture and that cellular structure aligned along the direction of applied pressure. The authors suggest that the bioreactor developed in this study was successful in promoting the formation of mechanically sound constructs and could be used to test the role of mechanical stimulation on bladderlike tissue [54].
In another study, Davis et al. [55] tested the effects of physiological bladder dynamics on cell viability using a bioreactor system (Fig. 4.4b). To engineer a bladderlike structure, they seeded human urothelial cells into a porcine bladder matrix and compared cell viability and proliferative capability in the resulting construct with that of constructs grown in conventional static culture. Cell viability analysis demonstrated that dynamic culture in the bioreactor significantly improved cell viability and proliferation after 4 days of culture. The authors suggest that a bioreactor system that provides mechanical stimulation is beneficial in recreating functional bladder tissue in vitro. Similar studies using different bioreactors have consistently confirmed these results [25, 56].
Several studies have also utilized the microenvironments of native tissues or organs as “in vivo bioreactors” that retain their original regenerative capabilities for cell proliferation. While a few studies have already used the in vivo environment for pre-vascularization of scaffolding systems [49], Campbell et al. [57] used the peritoneal cavity as an “in vivo bioreactor” to bioengineer visceral organs, such as the bladder and uterus. To produce tissue grafts, they implanted appropriate templates for different organs into rats or rabbits for 2–3 weeks. The produced tissue, which was found to contain a myofibroblast-rich cell population, was then harvested and reimplanted into the bladders (of what animals?). After 14 months, the implanted bladders developed morphologically normal bladder structure, suggesting that the in vivo environment may be used as a functioning bioreactor system to reconstruct bladder tissue for treating bladder augmentation cystoplasty.
In another study, Kajbafzadeh et al. used native bladder tissue as an in vivo bioreactor to determine appropriate scaffolding systems for bladder reconstruction [58]. They implanted several scaffolds (such as pericardium, biofilm, and polyglycolic acid (PGA) scaffolds or a combination of each type of scaffold) between bladder mucosa layers for several weeks and examined the immunological response and vascularization of the implants. Histological analysis revealed that PGA-coated pericardium was the most effective scaffold in terms of reducing inflammation and promoting efficient vascularization. This result suggests that a combination of biodegradable materials with acellular matrices will produce appropriate scaffolding systems that optimize cell attachment in vitro and bladder tissue reconstruction in vivo [58].
4.3.2 Urethra and Ureter
Urethral defect is a common disease that occurs secondary to urinary injuries, and treatment of these defects is limited by a shortage of implantable tissue [59]. Recent advances in tissue engineering and regenerative medicine have offered alternative solutions by producing functional urethral constructs in vitro. Although the cell-free scaffolding system has potential for use in treating urethral defects [60], several reports suggest that the use of cell-based urethral constructs may provide better results without any graft failure and stricture formation. In an early study, De Filippo et al. developed a 3D culture method to engineer urethral constructs. Autologous rabbit bladder cells were expanded and seeded into bladder submucosa, and the engineered urethral constructs were implanted into urethral defects in rabbits. To examine the effects of the seeded cells on urethral recovery, some animals were implanted with the scaffold alone. Histological and molecular analysis demonstrated that the seeded cells formed normal urethral structures 1 month after implantation, and the neoformations retained epithelial and smooth muscle cell phenotypes, as determined by Western blotting. Organ bath analysis confirmed contractility of the harvested urethral tissue, indicating efficient neural integration. However, implantation of the scaffold alone caused graft failure, characterized by strictures and poor tissue formation. These results suggest that a tubularized tissue construct with bladder cells can be fabricated and that such a construct may contribute to the reconstruction of defective urethral tissues. Different cell sources have also been used to engineer urethral tissue constructs. Since the epidermis seems to play an important role in the reconstruction of urethral tissue, Li et al. [61, 62] established a cell culture method for the expansion of oral keratinocytes. They isolated keratinocytes from the epidermis of rabbits, incorporated the keratinocytes into constructs seeded with various other cell types for engineering urethral tissues, and implanted the engineered constructs into urethral mucosa defect sites. Histological analysis showed that the constructs with keratinocytes facilitated better integration with host epithelium than those without keratinocytes. In fact, animals that were given the implants without keratinocytes developed inflammation, resulting in graft failure. These results suggest that the incorporation of epidermal layers into urethral constructs is helpful for promoting efficient mucosal integration with the host [62].
In addition to the formation of epidermal layers within engineered urethral constructs, mechanical stimulation has been applied to create mature, functional, urethral constructs. Fu et al. developed a robust bioreactor system that permits cyclic mechanical stimulation (Fig. 4.5a) [59]. To create their constructs, they incorporated several cell types, such as adipose-derived stem cells (ADSC) and oral epithelial cells, for muscular and mucosal layers, respectively. The epithelial cells were purified to obtain cells with higher proliferative capacity. Both types of cells were seeded onto fibrous PGA mesh using a layer seeding technique. The seeded construct was then placed into the bioreactor, where cyclic mechanical stimulation was applied to produce extended constructs. Histological analysis showed higher cell densities within these extended constructs compared to those not given mechanical stimulation (Fig. 4.5b). An in vivo study using dogs also revealed that implantation of the extended constructs resulted in improved urethral functional outcomes and normal structural morphology similar to that of autologous urethral tissue (Fig. 4.5c–d ). These results suggest that mechanical stimulation in combination with the use of appropriate cell types is necessary for engineering functional urethral constructs and that mechanically stimulated implants better facilitate urethral reconstruction following implantation.


Fig. 4.5
(a) An in vitro bioreactor system for engineering urethral constructs [59]. (b) Gross and histological images of the engineered urethral tissue construct in vitro. (c) Urethrography (left) and the harvested tissue images (right). (d) Histological evaluation of the harvested tissue. Reprinting of each image was permitted from publisher
Various approaches for reconstructing ureteral tissues have been developed in order to treat ureteral defects and lesions due to surgical dissection and traumatic injury. While a few studies have utilized cell-free scaffolding systems, such as biologic scaffolds [63, 64], more recent trials have focused on cell-based strategies to engineer ureteral tissue constructs for replacement of ureteral defects [65, 66]. In several trials, functional ureteral tissues preconditioned with mechanical stimulation have been fabricated using bioreactor systems. Vardar et al. developed a flow bioreactor system that mimics normal flow in the human ureter [67]. Cyclic mechanical stimuli were applied to collagen-based scaffolds seeded with human urothelial and smooth muscle cells, and phenotypic changes were examined by histological and molecular analysis. Dynamic culture under mechanical stimulation was found to significantly improve tissue formation and facilitate normal muscle tissue-specific phenotypes. These results also emphasize the importance of mechanical cues for the reconstruction of functional ureteral tissue, where a flow bioreactor system is necessary to provide cyclic mechanical stimulation. In another study using a bioreactor system, an automated bioreactor system able to control physiological conditions and cell culture parameters, such as pH and temperature, was established and used to produce tubular 3D tissue constructs [68].
4.3.3 Kidney
Kidney transplantation is the only definitive treatment for end-stage renal disease, but the lack of transplantable kidneys has resulted in an increase in the number of patients waiting for treatment. Recent advances in the field of regenerative medicine and tissue engineering have enabled scientists to recreate kidney tissue constructs in vitro, and preclinical trials have been performed to test the feasibility of restoring renal function using these functional constructs. Important components of this treatment strategy include (1) the development of scaffolding systems, (2) the establishment of kidney cell culture systems and 3D culture conditions, and (3) the designing of in vitro bioreactor systems. Early efforts have focused on the engineering of kidney tissue constructs using renal cells and scaffolding systems without bioreactor systems. Collagen-based biomaterials have often been used as scaffolds due to their biocompatibility and mechanical properties when seeded with renal epithelial and mesangial cells [69] and neonatal renal cells [70]. Our group has also developed a collagen gel-based culture system to create 3D renal constructs using primary renal cells [71]. To improve the mechanical properties of the engineered constructs, we used materials such as hyaluronic acid [72], synthetic polycarbonate material [73], and PGA scaffolds [74]. When the engineered renal constructs were tested in preclinical studies, the implants maintained renal-specific structures [73, 74] and, interestingly, produced urine-like fluids [73]. More recently, the development of induced pluripotent stem cells (iPSCs) has enabled the recreation of kidney tissues in vivo [75–78]. By combining an appropriate scaffolding system with iPSCs, researchers can produce large-scale renal constructs in vitro that can be used to treat renal failure in clinical trials. Although the use of implantable segments is a promising treatment for kidney failure, engineered segments may not able to replicate functions performed by a whole-sized kidney. To this end, an interesting approach to producing organ-sized kidney constructs has been developed for whole-kidney transplantation.
The concept of engineering whole-kidney constructs originated from utilizing native kidney architecture as a cell-seeding template [79–81]. The engineering process is composed of two techniques. First, cellular components are removed from the native kidney tissue in order to eliminate potential immune responses when the kidney construct is implanted into a normal, non-immunocompromised host [82]. This process, called “decellularization,” is usually completed by dynamically perfusing detergents through the entire kidney tissue. The optimal decellularization process completely removes cell components, maintains an intact extracellular matrix, and preserves vascular integrity. Preservation of the intact vascular network is particularly critical because it allows for efficient blood perfusion following implantation [79, 82]. The decellularized kidney scaffold is then recellularized in order to produce a functional renal scaffold. In the recellularization process, cells with kidney phenotypes are repopulated within the acellular collagen-based kidney scaffold. When undifferentiated stem cells are used to recellularize the kidney scaffolds, the seeded cells need to undergo the differentiation process for renal lineages. The use of a bioreactor is essential in the recellularization process for the creation of functional whole-kidney constructs in vitro. Unlike the previously mentioned trials that produced small scaffold segments with low cell-seeding densities, whole-kidney scaffolds are seeded with a high number of cells and therefore require efficient perfusion to maintain cellular viability throughout the entire scaffold. Several types of bioreactors have thus been developed to produce recellularized whole-kidney constructs [79–81, 83–85].
An early study confirmed the importance of bioreactor culture for repopulation of a whole-kidney construct. Ross et al. developed a bioreactor system to recellularize acellular rat whole-kidney constructs using ESCs [81]. The use of ESCs for this purpose is advantageous due to the high proliferative capacity and multi-differentiative ability of ESCs; however, several ethical and safety issues need to be addressed prior to using ESCs in clinical trials [86]. To examine cellular viability and tissue formation, the authors first cultured ESCs seeded within whole-kidney scaffolds under static conditions. However, most of the cells underwent apoptosis within a few days of culture, so the authors then established a perfusion culture system based on a bioreactor system. The perfusion culture system was equipped with a peristaltic pump, a tubing, a pressure monitoring system, and a cyclic beating system that maintained constant physiologic pressure (120/80 mmHg) with periodic beating (270–300 beats/min), constant CO2, and constant temperature. Over 10 days of perfusion culture, the whole-kidney constructs, which were seeded with ESCs through the renal artery or ureter, maintained viable cells and facilitated proliferation of seeded ESCs within tubular, vascular, and glomerular structures. A majority of the ESCs lost their pluripotency during bioreactor culture, implying that they may have undergone renal differentiation. In a subsequent study, the authors also demonstrated that the seeded ESCs were able to differentiate into the endothelial cell lineage [83]. Interestingly, the attachment of mouse ESCs induced remodeling of the basement membrane in the rat-derived kidney matrix, suggesting a possible strategy for clinical xenotransplantation. Overall, these results suggest that acellular whole-kidney scaffolds can be repopulated with pluripotent cells using a bioreactor system with simulated physiological conditions in order to form renal tissue.
Other studies focus on monitoring physical and biochemical function during perfusion bioreactor culture. The examination of viable and functional kidney constructs for implantation is preferably done in a noninvasive manner. Uzarski et al. developed a bioreactor system that allowed them to examine renal function in terms of cell viability and renal-specific behaviors [84] (ref). Using the bioreactor system, the authors were able to measure decellularization efficiency in a noninvasive manner, monitor (arterial?) pressure changes due to the level of recellularization, and evaluate recellularization efficiency by measuring cell viability (resazurin) and renal function (alumin, kidney injury molecule-1) through sampling the culture medium. The results demonstrate that this bioreactor system may be successfully used to scale up and broaden the applications of whole-kidney technology.
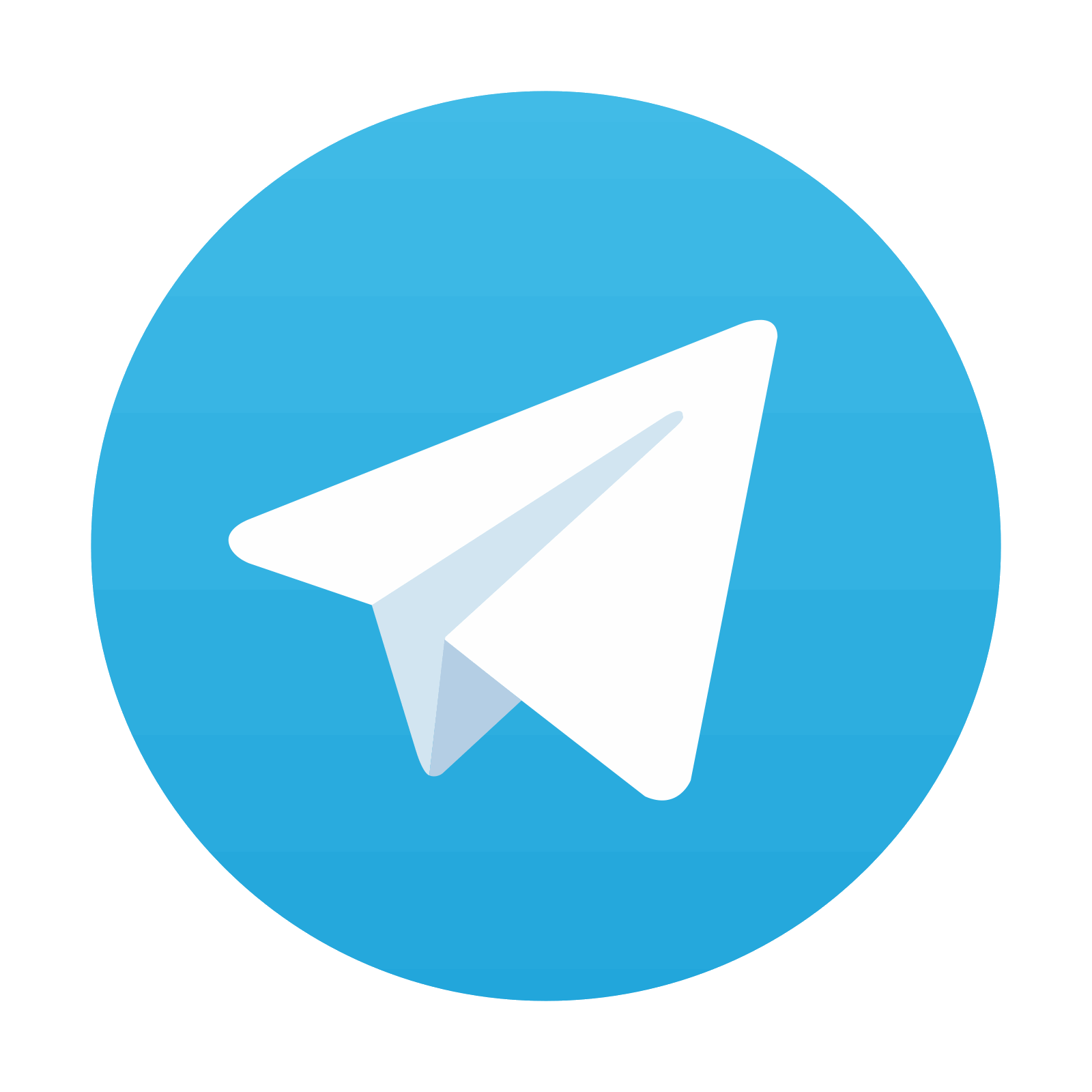
Stay updated, free articles. Join our Telegram channel
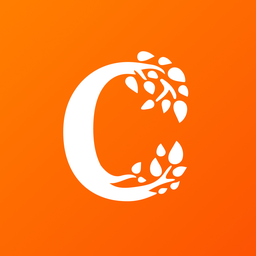
Full access? Get Clinical Tree
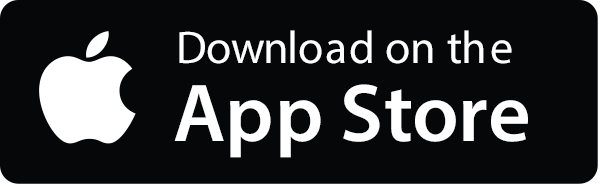
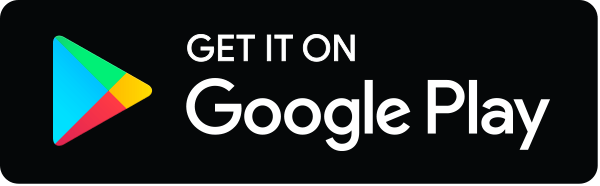