Fig. 5.1
The classical tissue engineering approach
Recently, bioprinting has emerged as a technology within tissue engineering; it is a two-dimensional (2D) or three-dimensional (3D) fabrication method that uses living cells, biomaterials, and bioactive molecules. Using bioprinting technology, tissue- or organ-like architectures, composed of multiple cell types, can be produced. Moreover, such printing processes can be automated using computer-aided design templates. Many researchers working in regenerative medicine have tried to develop biomimetic architectures with this technology. In this chapter, current bioprinting technologies and their applications are introduced.
5.2 Bioprinting Techniques
Bioprinting techniques can be categorized into three types: jetting-, extrusion-, and laser-based bioprinting (LBB). In this section, the three techniques are described in terms of their working principles, components, advantages, disadvantages, and applicable bio-inks.
Table 5.1 summarizes achievable specifications for each bioprinting technology, in terms of resolution, printing speed, cell viability and density, materials, applicable viscosity, gelation method, and advantages and disadvantages.
Table 5.1
Summary of bioprinting techniques
Printing technique | Jetting-based bioprinting | Extrusion-based bioprinting | Laser-based printing | References | |
---|---|---|---|---|---|
Stereo-lithography | Laser-assisted bioprinting | ||||
Resolution or droplet size | <85 μm | 5 μm ~ | <1.2 μm | 8.7–140 μm | |
Printing speed | 10 μm/s–50 mm/s | 2–5 mm/s | 50 μm/s–5,000 mm/s (laser scanning rates) | 200–2,000 mm/s (laser scanning rates) | |
Cell viability | >89.8% | 70.0–93.0% | > 85.0% | 63.8–96.0% | |
Cell density | 106 cells/mL | <107 cells/mL | 106 cells/mL | 107–108 cells/mL | |
Material | Hydrogel, media, cells, proteins and drug | Hydrogel, cell aggregates (w/ or w/o media), bioceramics, proteins and drug | Photocurable hydrogel, cells, and bioceramics | Hydrogel, cell aggregates (w or w/o media), peptides, and bioceramics | |
Material viscosity | 1–18 mPa/s | 30,000–50,000 mPa/s | 2.05–5,000 mPa/s | 40–120 mPa/s | |
Gelation method | Ionic, thermal, enzymatic, and photo crosslinking | Ionic, thermal, enzymatic, photo, chemical and pH crosslinking | Photo crosslinking | Ionic and pH crosslinking | |
Advantages | Low printer cost, control droplet size, availability to many biomaterials and control cell concentration by changing drop density | Capacity to extrude cells up to high densities and wide range of viscosity | Control pore distribution, pore size and shape of a scaffold, high resolution and less effect of temperature during the printing process | Nozzle-free, no clogging and wide range of viscosity | |
Disadvantages | Frequent nozzle clogging, mechanical and thermal stress to cells, difficulty in cell encapsulation, limitations of material viscosity, requirement for crosslinkers which are cytotoxic and low cell density than other techniques | Extrusion pressure and crosslinkers decrease cell viability | Limited printing materials | Long preparation time, poor cell positioning/targeting, contamination by metallic residues and high cost |
5.2.1 Jetting-Based Bioprinting
Jetting-based bioprinting generates and delivers small droplets to a desired site to produce cell patterning; it is also called “drop-on-demand” printing (Fig. 5.2). This method is easy to develop because it needs only minor modifications from a “traditional” inkjet printer. The first modification is to replace the ink with a bio-ink; the second is that an elevator is placed under the printing head to achieve a layer-by-layer process for the construction of a 3D structure. Thus, a jetting-based bio-printer consists essentially of the printing head and elevator. The printing head produces droplets using a thermal piezoelectric actuator, or a solenoid valve. The first method involves a heater attached to the printer head. The heater generates thermal energy using electric current pulses. The energy induces vapor bubbles within the ink. Then, these bubbles push the ink out of the nozzle, so that droplets can be generated. The second method uses a piezoelectric actuator on the printer head. The actuator is triggered by applying a voltage pulse, so that the actuator deforms and squeezes ink out of the nozzle. The third method is solenoid valve-based bioprinting. The valve is controlled by a microcontroller, so that the size of droplets can be changed by the valve-open duration.


Fig. 5.2
Jetting-based bioprinting (using piezoelectric pressure (left), thermally induced air-pressure (middle), and solenoid valve (right))
Jetting-based bioprinting has several advantages. First, this method is relatively inexpensive in comparison with extrusion- based bioprinting and laser-based bioprinting. Second, the printed concentration of cells can be adjusted readily by controlling for the density of droplets. Finally, this methodology can use a variety of biomaterials as bio-ink [4]. For example, Horváth et al. [5] developed an in vitro air–blood tissue barrier using hy926 endothelial cells and A549 epithelial cells with Matrigel. Scoutaris et al. [7] reported printing results with cetirizine HCl (CTZ), diphenhydramine HCl (DPD), and ibuprofen (IBU).
However, jetting-based bioprinting has an intrinsic limitation in terms of the biomaterials; these should have a low viscosity (1–18 mPa/s) because the technique requires the generation of small droplets [8]. The viscosity also affects the speed of the process and the cell viability. To create a rigid scaffold using low-viscosity materials, a time-consuming process for crosslinking is typically required after printing. When droplets are created from the printer head, cell viability can be influenced by thermal or mechanical stress. Moreover, the available cell density is limited, because nozzle clogging is an issue with this technology [4].
Recently, several new methods have been introduced to overcome these difficulties. Kador et al. [9] suggested a design for a retinal model produced by thermal inkjet printing. They overcame the limitations of the electrospinning approach, in terms of cell positioning, by combined use of jetting-based bioprinting. Xu et al. [10] prepared functional cartilage tissue with electrospinning. The electrospinning technique was used to address the disadvantages of jetting-based bioprinting in terms of mechanical properties. Moreover, Binder [11] developed an in situ skin bioprinter for enhancing the healing of skin wounds. They directly printed skin cells, including fibroblasts and keratinocytes, on a skin wound.
5.2.2 Extrusion-Based Bioprinting
Extrusion-based bioprinting is a technique to create 3D cellular constructs by dispensing continuous filaments with a cell-laden hydrogel. The printer consists of a cartridge, deposition stage, and printer head, composed of a syringe and nozzle. According to the dispensing system, extrusion-based bioprinting is categorized into two types: pneumatic and mechanical extrusion systems (Fig. 5.3). Pneumatic extrusion-based bioprinting prints bio-ink out of the nozzle by applying air pressure. The amount of bio-ink is adjusted by controlling the air pressure. This method can use relatively high-viscosity materials, in comparison with jetting-based methods. Mechanical extrusion-based bioprinting uses a piston or screw for printing. Piston-based bioprinting controls the volume of ink extruded directly. Screw-based dispensing can produce much higher forces, so the system can handle materials of much higher viscosity.


Fig. 5.3
Extrusion-based bioprinting (using pneumatic (left) and mechanical (piston (middle), and screw (right)) pressure)
As mentioned above, extrusion-based bioprinting can handle high-viscosity materials, of about 30,000–50,000 mPa/s [12]. Thus, high concentrations of cells can be used in such a system. A cell concentration of ~1 × 107 cells/mL has been reported [13]. Moreover, a wide range of biomaterials has been used in extrusion-based systems. Luo et al. [14] created a scaffold with alginate, Pluronic F127, and a bioceramic (Ca7Si2P2O16) powder for bone tissue engineering. Latza et al. [15] studied a device for wearable electronics using sucker ring teeth (SRT; from squid) protein, water, and glycerol. Yang et al. [13] produced osteochondral biphasic grafts using alginate, cartilage-derived ECM, PLGA, hydroxyapatite (HA), and cells.
Recently, various functional bio-inks have been developed for extrusion-based bioprinting. Rees et al. [17] suggested a nanocellulose (carboxymethylation and periodate oxidation)-based bio-ink that could prevent bacterial growth. The material was used to print a wound dressing. Highley et al. [16] developed a supramolecular self-healing hydrogel, which was cured by applying a physical stimulus. The hydrogel was restored when the physical stimulus was removed. The material could be used to construct complex structures with high resolution. Cho’s group used a decellularization process to develop tissue-specific bio-inks with adipose, cartilage, and heart tissue. These bio-inks provided enhanced biocompatibility for tissue growth in a 3D structure [18].
Extrusion-based scaffold-free bioprinting has also been described, in which cell aggregates having spherical or rod shapes are printed directly with no additional biomaterials [20, 21]. Using the bio-printed cell aggregates, organ-specific biological features could be mimicked. Moreover, tissue-like cell densities can be achieved. For example, Itoh et al. [20] introduced a tubular structure with multicellular spheroids. However, the structure did not easily stand by itself without supporting materials, so a fine architecture could not be formed. To overcome this, Kucukgul et al. [21] introduced a new process. They defined a designed structure and empty spaces. In the empty spaces, a supporting structure was printed with agarose gel. Then, computer-designed structures were plotted with the rod-shaped cell aggregates.
5.2.3 Laser-Based Bioprinting (LBB)
The LBB technique involves constructing a structure by solidifying or depositing bio-ink with photon energy. This technique can be divided into stereolithography (SLA) (Fig. 5.4) and laser-assisted bioprinting (LAB) (Fig. 5.5).



Fig. 5.4
Laser-based bioprinting (stereolithography (SLA))

Fig. 5.5
Laser-based bioprinting (laser-assisted bioprinting (LAB))
5.2.3.1 Stereolithography (SLA)
SLA produces 3D structures with a scanning laser beam on a photo-curable material. Thus, the system consists primarily of a laser and optics, a photopolymer container, and an elevator. The laser beam irradiates the photopolymer. Galvanometric mirrors and lenses are used to control the position of the beam and its focusing. Transferred photon energy initiates the photo-curing process and a 2D pattern is generated by scanning the beam. Then, a 3D structure can be produced, by stacking the patterns layer-by-layer.
SLA technology has good spatial resolution, of <1.2 μm [22]. Recently, higher resolutions have been obtained using two-photon polymerization (TPP). TPP uses a femtosecond laser to obtain a high-intensity near-infrared beam that induces localized excitation by two-photon absorption. This then induces nano-scale photo-polymerization. Haske et al. [23] achieved a resolution of 65 nm. Thus, SLA can control the shape of a scaffold, its pore distribution, and overall size very precisely. Other advantages of SLA technology include the speed and temperature of the fabrication process. The speed is high because the laser scanning rate can be up to 5000 mm/s [24]. The temperature during the fabrication is lower than with other techniques, so that cells are less affected [25]. SLA can use several types of biomaterials. Lee et al. [26] introduced a customized poly(propylene fumarate)/diethyl fumarate photopolymer and built 3D scaffolds for bone tissue engineering. Jansen et al. [27] created porous structures with a well-defined gyroid architecture for tissue engineering using pentaerythritol tetra-acrylate resin, ceramic suspensions, and PDLLA3-FAME/NVP resins. However, the number of applicable biomaterials for SLA is still small in comparison with the other techniques, because SLA requires a photocurable polymer. Recently, Shanjani et al. [28] reported a hybrid printing system combining SLA and extrusion-based bioprinting to allow use of “softer” materials as the bio-ink.
Recently, researchers have studied building 3D cellular scaffolds using SLA [29–31]. Soman et al. [29] created an in vitro model with PEG material and cancer cells to mimic a metastatic process. SLA has also been used to construct microfluidic chips, because the method is cost-efficient and the process can be automated. Shallan et al. [31] developed a complex monolithic device using a transparent resin. Au et al. [32] evaluated a commercially available SLA system to produce computer-designed microfluidic chips. It was validated by culturing and imaging with Chinese hamster ovary (CHO) cell on the chip.
5.2.3.2 Laser-Assisted Bioprinting (LAB)
LAB involves primarily a laser, bio-ink-coated ribbon, and the collector substrate. Lasers can be divided into pulsed and continuous devices. The ribbon consists of a glass or quartz plate, a metal layer, typically of gold, silver, or titanium and an energy absorbing layer (interface layer) made of a sacrificial hydrogel. The bio-ink is then coated on the energy absorbing layer. The collector substrate is coated with biopolymer and cell culture medium to enhance adhesion of the cells.
The laser pulse is focused and irradiated on the bio-ink-coated ribbon using the optics. Then, the energy absorbing layer is expanded thermally and generates vapor bubbles to form and jet droplets of bio-ink. The droplets fall on the receiving substrates so that the designed 2D cell pattern is created. The two most common methods of LAB are absorbing film-assisted laser-induced forward transfer (AFA-LIFT) and matrix-assisted pulsed laser evaporation direct writing (MAPLE-DW). AFA-LIFT is modified from LIFT for bioprinting. AFA-LIFT uses a sacrificial metal, such as gold or titanium, for the energy absorbing layer. It uses a high-power laser system and the metal layer can induce metallization of the ejected ink. This can affect the cytotoxicity of the process. To overcome this, MAPLE-DW uses a lower-power laser and a sacrificial hydrogel, such as Matrigel, for the energy absorbing layer [33].
LAB uses a laser pulse, so that relatively high resolution can be achieved, from 8.7 μm to 140 μm [31, 34]. Laser scanning rates up to 2000 mm/s have been reported; this high printing speed can be advantageous [35]. LAB uses a ribbon, so a nozzle is not necessary. Thus, nozzle clogging is not a concern, so materials of various viscosities can be used for printing. Additionally, the cell density can be increased, up to 108 cells/mL [36]. However, the ribbon is used over a relatively long time and high-precision cell patterning cannot be guaranteed. LAB is expensive because of the laser and it has limitations in terms of scaling up the structure [37].
Several bio-inks have been introduced for use with LAB technology. Pagès et al. [35] created 3D constructs for complex tissue substitutes using collagen and cells in medium. Dinca et al. [38] introduced a controllable self-assembly technique for peptides for use in biosensors and tissue engineering. Cheng et al. [39] developed a unique process to coat HA powders on titanium substrates.
Recently, several advanced processes have been reported for LAB. Catros et al. [40] optimized experimental conditions using inorganic nano-hydroxyapatite (n-HA) and human osteoprogenitor cells. They showed that cell viability, proliferation and phenotype were maintained without changes in the physicochemical properties of n-HA. Xiong et al. [41] printed straight and Y-shaped tubes using a ribbon composed of alginate and cells in medium. Their preliminary results suggest the technique is valuable for 3D tubular constructs and organ printing.
5.3 Applications
Recently, 3D bioprinting has been used widely in applications such as tissue engineering, body-on-a-chip, and drug delivery systems. Table 5.2 shows a summary of bioprinting applications sorted by printing methodology, cell source/protein, and biomaterials.
Table 5.2
Bioprinting applications for blood vessel
Printing method | Cell source/ protein | Material | References |
---|---|---|---|
Extrusion-based | HUVECs, SMCs, and NHDFs | ECM, SMCM, FM, and gelatin | [20] |
Extrusion-based | HCASMCs | Alginate, MWCNT | [64] |
Extrusion-based | L929 mouse fibroblasts | Alginate | [65] |
Extrusion-based | HUVECs, NHLFs | Collagen, gelatin, fibrin | [66] |
Laser-based | Breast cancer cell (MDA-MB-231 and MCF-7) and fibroblasts (BJ5ta) | Gelatin | [59] |
Laser-based | HUVSMCs | Alginate | [41] |
5.3.1 Tissue Engineering
As mentioned above, the “traditional” scaffold-based approach has difficulties in terms of vascularization and developing a complex tissue or organ. In tissue engineering, 3D bioprinting is considered a breakthrough technology. Bioprinting processes can use multiple cell types and precisely control scaffold geometry in developing complex tissues. This subsection introduces artificial vessels, bones, cartilage, and organs produced with 3D bioprinting technology.
5.3.1.1 Blood Vessels
Blood vessels are the part of the cardiovascular system that transfers blood in circulation, transporting nutrients, oxygen, carbon dioxide, hormones, and blood cells to and from tissues. To prepare scalable organs, the artificial formation of blood vessels is one of the most important issues in tissue engineering. The vessel is tube-shaped, having a lumen, endothelium, and basement membrane. Printing a blood vessel is a challenge because of its complexity.
Many studies have been reported on the construction of artificial vessels. Itoh et al. [20] produced vascular prostheses with human umbilical vein endothelial cells (HUVECs), human aortic smooth muscle cells (HASMCs), and human normal dermal fibroblasts (HNDFBs) using a scaffold-free bioprinting technique (Fig. 5.6). The tubular tissues were implanted in the aortas of male F344-rnu/rnu athymic nude rats. They observed endothelial cells on the inner surface of the tubular tissues and normal flow rates in the aortas using percutaneous ultrasonography. Also, thrombosis did not occur in any rat.


Fig. 5.6
Scaffold-free vascular graft with MSCs. Adapted from Itoh et al. PLoS One 2015;10(9):1–15 [20]
Miller et al. [62] used a printable, cyto-compatible, and sacrificial material to produce micro-channels incorporating a hydrogel structure. After printing a 3D channel network, cell-laden hydrogel was infiltrated into the sacrificial mold and cross-linked (Fig. 5.7). Then, the mold was dissolved to produce hollow channels. An artificial vascular structure was produced by seeding HUVECs in the channel and culturing them. Then, the construct was implanted in the circulatory system of a rat. The results showed that the rat femoral artery could sustain physiological blood pressure, from 80 to 100 mmHg and a normal level of flow was observed [63].


Fig. 5.7
A schematic overview of the casting of perfusable vascular architectures by using bioprinted sugar lattice. Reprinted from NATURE MATERIALS, Miller JS, Stevens KR, Yang MT, Baker BM, Nguyen D-HT and Cohen DM, Rapid casting of patterned vascular networks for perfusable engineered three-dimensional tissues, 2012;11(7):768–74, Copyright © 2012, Rights Managed by Nature Publishing Group [62]
Dolati et al. [64] used a coaxial bioprinting process and printed vascular conduits directly. They prepared alginate reinforced with carbon nanotubes and then encapsulated human coronary artery smooth muscle cells (HCASMCs) in the material for printing. Their method had flexibility in terms of scale, from micro- to sub-millimeter. Similarly, Gao et al. [65] made a built-in micro channel structure by using layer-by-layer printing. They used a coaxial nozzle and printed hollow calcium alginate filaments, encapsulating L929 mouse fibroblasts.
Thus, arteries have been printed successfully. However, a capillary network at the single-cell scale is much harder to print. To address this, several researchers have studied perfusable capillary networks to induce angiogenesis. Lee et al. [66] used HUVECs and biological matrices and formed larger fluidic vascular channels. The capillary network was constructed by angiogenesis, induced from the printed channels.
5.3.1.2 Bone and Cartilage
Many studies have been reported on the regeneration of bone and cartilage. Tissues can be printed using patient data, acquired with imaging techniques. This has been shown to be an effective method for reconstructing structures damaged by osteoporosis and bone fractures, for example.
Roh et al. [67] produced scaffolds using oxygen plasma treatment and a PLGA/n-HAp/β-TCP composite. Based on the scaffold, they enhanced the initial adhesion, proliferation, and differentiation of preosteoblasts. Van Rie et al. [68] printed scaffolds using cryogel-PCL composite and seeded MC3T-E1 osteoblast precursor cells. They observed colonized cells attached to the porous cryogel network.
Luo et al. [14] described a hollow struts-packed (HSP) bioceramic scaffold using a coaxial 3D printing strategy. The HSP bioceramic scaffold enhanced the proliferation and attachment of cells. When this structure was implanted in the damaged femur of a rabbit, recovery of a large bone defect was observed because of the implant’s osteo-conductivity (Table 5.3).
Table 5.3
Bioprinting applications for bone
Printing method | Cell source/protein | Material | References |
---|---|---|---|
Extrusion-based | Osteoblast precursor cells (MC3T3-E1) | PLGA and PLGA/n-HAp/β-TCP | [67] |
Extrusion-based | Osteoblast precursor cells (MC3T3-E1) | PCL, cryogel, and gelatin | [68] |
Extrusion-based | N/A | Bioceramics, Pluronic F-127, and alginate | [14] |
Extrusion-based | ihMSCs | PLGA-PEG-PLGA triblock copolymer hydrogel | [84] |
Extrusion-based | Human AFSCs | Gelatin, fibrinogen, HA, glycerol, and PCL | [70] |
Laser-based | MG-63 cells | n-HA and Matrigel | [40] |
Izadifar et al. [69] performed a layer-by-layer process with two bio-inks and a PCL. The first bio-ink was composed of alginate and primary chondrocytes extracted from embryonic chick cartilage. The second contained ATDC5 mouse cell with alginate. The structure showed outstanding performance in terms of proliferation, cell viability, and secretion of cartilage ECM. Moreover, the real thickness of human articular cartilage could be achieved.
Kang et al. [70] developed an integrated tissue-organ printer (ITOP) and applied it to the regeneration of bone, cartilage, and muscle tissue. They printed a cell-laden hydrogel and a biodegradable polymer (PCL), anchored in sacrificial Pluronic F127, into a single structure. Their method increased the mechanical stability of the scaffold and overcame limitations in terms of structural integrity, size, and shape. To obtain a more precise structure, tissues were constructed using medical image data (Table 5.4). They implanted the structure in nude rats and observed the functionality of the structure (Fig. 5.8).
Table 5.4
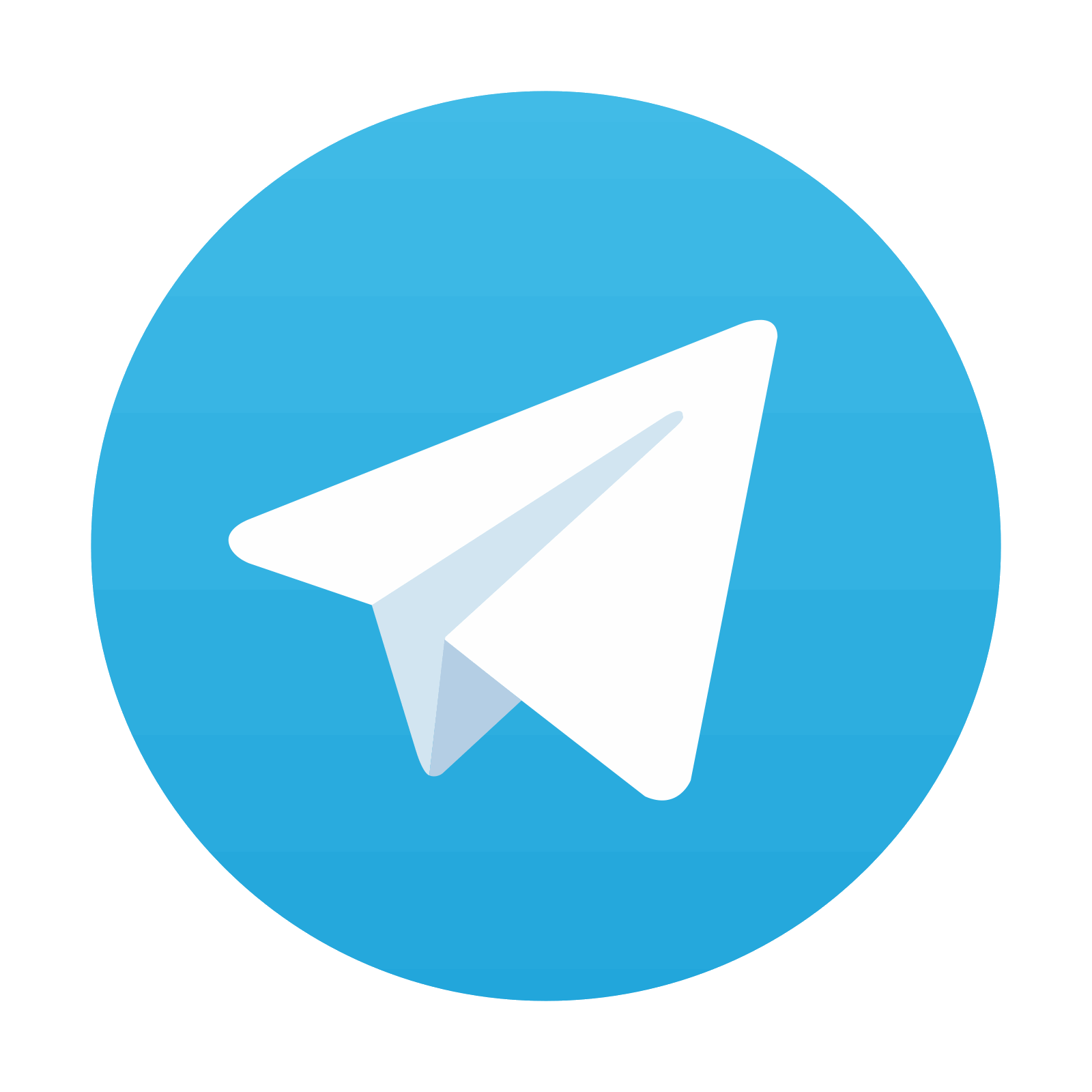
Bioprinting applications for cartilage
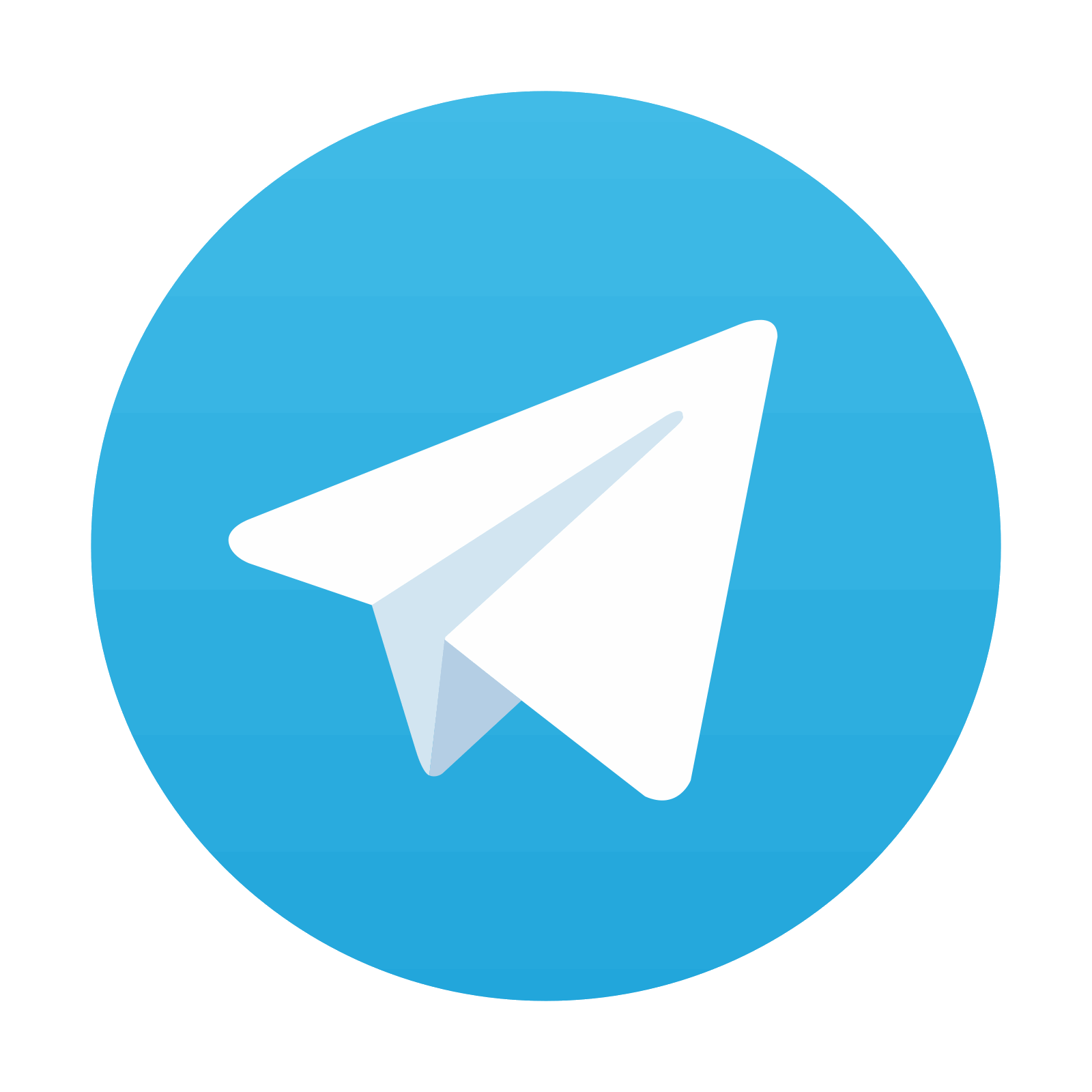
Stay updated, free articles. Join our Telegram channel
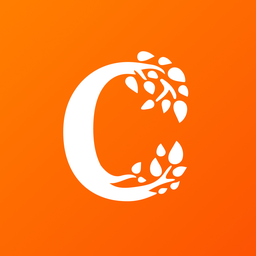
Full access? Get Clinical Tree
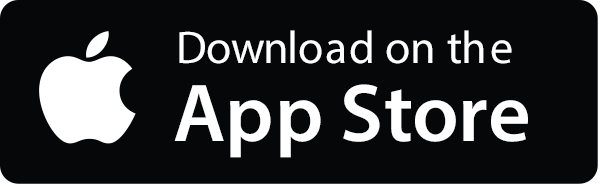
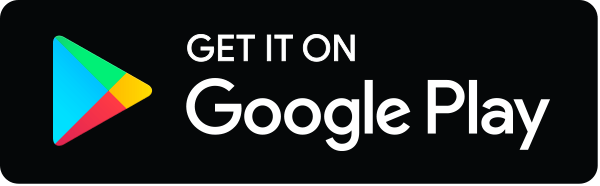
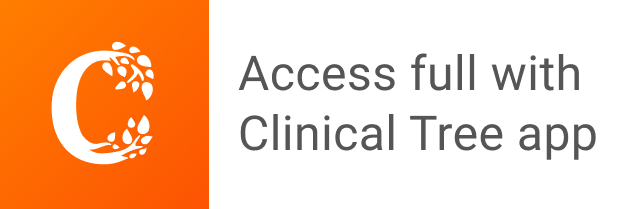