© Springer Nature Singapore Pte Ltd. 2018
Bup Wan Kim (ed.)Clinical Regenerative Medicine in Urologyhttps://doi.org/10.1007/978-981-10-2723-9_11. Current Developments and Future Perspectives of Tissue Engineering and Regenerative Medicine
(1)
Wake Forest Institute for Regenerative Medicine, Wake Forest University School of Medicine, Winston-Salem, NC, USA
1.1 Introduction
Tissue engineering and regenerative medicine (TERM) is an interdisciplinary field encompassing many disciplines, including engineering, medicine, and science. The field has gained an enormous attention due to its potential to replace damaged human tissues and organs and restore normal function [1–3]. Although the early concept of tissue engineering was developed based on cell culture techniques, recent advances in the field combine multiple innovative technologies to accelerate the translation of clinical therapies. In fact, a number of TERM technologies have advanced to human clinical trials and commercialized [4, 5]. In this chapter, we review the current developments and recent progresses made in the field of TERM and discuss the most relevant challenges and future perspectives in the translation of TERM research.
1.2 Current Developments in TERM
Aligned with the goals of TERM, research activities have advanced in many disciplines toward developing new therapies that overcome the current limitations associated with conventional medical and surgical therapies. Over the years, numerous strategies have been applied for regenerating or replacing damaged tissues and organs, which led to the development of new therapies and products for patients [4, 6–9]. Accordingly, several urological tissue technologies have been developed for clinical use, including bladder, urethra, and ureter [9–11]. In this section, we review the current status of TERM therapies and discuss new and innovative technologies that hold promise in the future.
1.2.1 Cell Source
Cells are one of the main basic components of TERM. Cells can be injected or infused into damaged tissues alone or by combining with cell carrier materials. Cells can also be used to engineer tissue constructs by attaching on a scaffolding system fabricated with biomaterials. These cells can mature into tissues and organs by direct proliferation and differentiation into tissue-specific cells. Implanted cells can also stimulate tissue formations indirectly by releasing biological factors. In TERM therapies, allogenic and autologous cells have been used frequently. Autologous cells are preferred over allogenic cells because these cells are isolated from the host and therefore minimize rejection and eliminate side effects caused by immunosuppressive medications [4]. Several types of autologous cells, such as smooth muscle cells, urothelial cells, and muscle-derived cells, have been used in the preclinical and clinical settings with successful outcomes in the field of urology [12–14]. Autologous cells are usually isolated from diseased or damaged tissues of the host; however, obtaining sufficient amount of normal cell population may be difficult in selected cases. Additionally, fully differentiated cells may show limited proliferative capacity [15]. Therefore, alterative cell sources may be needed to obtain sufficient numbers for clinical therapies.
Stem cells are an alternative cell source that can overcome the limitations of autologous somatic cells [16–20]. Stem cells have the ability of self-renewal and can be differentiated into one or more specialized cell types. Stem cells are traditionally classified according to their origin: embryonic stem cells, fetal stem cells, or adult stem cells. Stem cells can also be classified by their plasticity: totipotent, pluripotent, or multipotent. Embryonic stem cells have a strong capacity for self-renewal with the potential to differentiate into any type of cells, so they are an effective stem cell source for regenerating tissues and organs. However, the use of these cells is very controversial at the present time. Embryonic stem cells are obtained from the inner cell mass of embryos. During the isolation process, the embryo is destroyed, and somatic nuclear transfer and cloning processes are required [21, 22]. Aside from the ethical issues, embryonic stem cells are known to form teratomas in vivo [22]. Thus, many countries prohibit the use of embryonic stem cells in research.
Adult stem cells can be obtained in most tissues and organs in the body, including the bone marrow, fat tissue, blood, heart, nerve tissue, muscle, skin, and urine [18, 23]. Although adult stem cells have lower self-renewal and differentiation capacity than embryonic stem cells, they are relatively free from ethical and safety issues and are easily translated to patients. Adult mesenchymal stem cells, which are derived from bone marrow or adipose tissues, have been used in numerous studies [24]. Bone marrow-derived stem/stromal cells can differentiate into various cell types, including osteocytes, chondrocytes, and myocytes, and can develop into many tissue types including the bone, cartilage, muscle, vessels, and liver. Since Zuk and colleagues have first reported on how to obtain multipotent stem cells from human adipose tissues in 2001 [25], the use of adipose-derived stem cells has been enormously increased. Adipose-derived stem cells are multipotent cells, like bone marrow-derived stem cells. However, these cells are easier to obtain with high yields than bone marrow-derived stem cells [26, 27]. With these favorable characteristics, adult mesenchymal stem cells have been frequently used in bladder and urethral reconstructive applications [9, 28].
Another attractive source of stem cells in TERM is amniotic fluid and placental stem cells. These cells are a mixture of multipotent stem cells that express embryonic and adult stem cell markers [29]. They can differentiate into a variety of cell types, including chondrogenic, osteogenic, hepatic, myogenic, neuronal, and hepatic lineages. They have over 250 population doublings and do not form tumors in vivo [29–31]. With these advantages, amniotic fluid stem cells have shown therapeutic effects in various tissue regeneration studies. For instance, the injection of a combination of early differentiated amniotic fluid stem cells into damaged urethral sphincter in mice showed new muscle fiber formation and improved urodynamic function [32]. Thus, amniotic fluid and placental stem cells are considered a promising cell source for cell-based TERM research. Their applications are expected to increase in preclinical and clinical settings [31].
Recently, induced pluripotent stem cells (iPSC) have gained an enormous attention as novel cell sources for TERM applications. These cells are generated by dedifferentiation of adult somatic cells through genetic reprogramming [33]. iPSC were first discovered by Takahashi and Yamanaka in 2006 through the reprogramming of adult mouse fibroblasts by overexpressing four transcription factors, Oct-4, Sox-2, c-Myc, and Klf4 [34]. Pluripotent stem cells can be obtained without the use of embryos. Recently, a few studies have shown the possibility of reprogramming human cells, as they show similar morphology, gene expression, and surface markers as human embryonic stem cells [35–37]. These results have opened a new era in TERM, as they can be utilized as personalized cell sources, not only for regenerating damaged tissues or organs of the patients but also for establishing patient-specific platforms in drug discovery. In addition, a recent study reported that human iPSC-derived smooth muscle precursor cells improve urethral sphincter function in a rat model. As such these cell sources have received much attention in urology [38]. Issues related to incomplete reprograming and teratoma formation still remain to be solved, but research of iPSC has seen a rapid and exponential growth [39–42].
Stem cells have been also found in urine [28]. Urine-derived stem cells have high self-renewal and expansion capacity and low telomerase activity and secrete paracrine factors. These cells have multipotent differentiation potential, including osteogenic, chondrogenic, myogenic, adipogenic, neurogenic, and endothelial differentiation. Urine-derived stem cells have become a promising cell source in urology, because the origin of these cells is from the urinary tract system, and they can be differentiated into bladder cells [23, 28, 43].
1.2.2 Biomaterials
Biomaterials are an essential element in TERM research. Biomaterials can be used alone as scaffolds or used with cells as tissue constructs. Biomaterials serve as a bridge to fill defect sites and promote new tissue formations by inducing the body’s ability to regenerate. Biomaterials can also deliver cells into the body to facilitate rapid regeneration. Biomaterials provide environment for cell attachment, growth, and differentiation. Therefore, an ideal biomaterial should be biocompatible and support tissue developments by appropriate control of cell attachment, proliferation, migration, and differentiation [44]. When biomaterials are implanted in the body, they should readily integrate with host tissues without inducing unfavorable inflammation. Biomaterial scaffolds possess porous structure to facilitate cell migration and induce vascularization, as well as provide structural support. Biodegradable biomaterials specifically should provide mechanical support in early tissue development and should degrade at a controlled rate over time, thereby promoting new tissue formation.
Numerous biomaterials with specific properties have been developed and applied in TERM research. Selection of biomaterials is extremely important for the successful outcome of research studies. Biomaterials are traditionally classified according to their origin: synthetic and natural polymer [44, 45]. Biodegradable synthetic polymers such as poly-lactic acid (PLA), poly-glycolic acid (PGA), poly-lactic-co-glycolic acid (PLGA), and poly-caprolactone (PCL) have been widely used in TERM studies due to several advantages. These polymers are easy to obtain and handle as compared to natural polymers, although synthetic polymers are generally not as biocompatible as natural polymers. Biodegradable synthetic polymers can be manufactured in a large scale with reproducible physical and chemical properties, and their degradation rates are controllable. Natural polymers that are frequently used in TERM include collagen, gelatin, alginate, fibrin, silk, chitosan, and hyaluronic acids. Although naturally derived materials are difficult to control their degradation rates, they have advantages of possessing biologic recognition and biocompatibility.
Recently, injectable biodegradable hydrogels, such as collagen, gelatin, alginate, fibrin, hyaluronic acid, and polyethylene glycol (PEG), have been extensively used in TERM research. Hydrogels can cross-link, absorb water up to 1000 times their dry weight, and provide microenvironments similar to that of native tissues [46]. They can be injected in combination with cells and bioactive molecules, such as growth factors or genes. Despite these advantages in TERM research, the use of hydrogels is limited due to the weak mechanical properties and rapid degradation [47]. As such, various types of synthetic hydrogels and hybrid/composite biomaterials have been investigated in order to improve these properties [46, 48].
Current approaches in biomaterials research have focused on developing smart biomaterials that provide bioactive functions. Smart biomaterials have been investigated with the purpose of accelerating or enhancing tissue regeneration by controlling biological activities of cells and releasing regulatory factors [49, 50]. Consequently, a strategy of in situ tissue regeneration has gained an increasing interest in the field. This strategy uses the body’s own regenerative capacity by activating and recruiting host stem/progenitor cells into the scaffold implant. For facilitating migration, recruitment, homing, growth, and differentiation of host cells, functional scaffolding systems containing bioactive molecules, including growth factors, peptides, and drugs, have been used. By controlling spatiotemporal release of the bioactive molecules contained in the scaffolding system, tissues can be regenerated by using only the host cells [51–53]. This strategy eliminates ex vivo cell manipulations, which are labor intensive and time-consuming and require enormous resources.
Decellularized tissues and organs have been used as scaffold biomaterials. Decellularization is the process that removes cellular components from donor tissues and organs, while retaining extracellular matrices. Decellularized scaffolds preserve the architectural structures and shape of organs and tissues. Due to lack of cellular components, immunorejection can be minimized. Therefore, use of decellularized scaffolds has become an alternative strategy to allogeneic organ transplantation [54]. In urology, decellularized urinary bladder matrix and small intestinal submucosa have been investigated extensively for tissue reconstruction. These approaches have been successfully translated into the clinic for bladder augmentations [8, 12, 55–57]. Moreover, recellularization of decellularized lung, kidney, and heart scaffolds has been tried preclinically to achieve functional tissues for whole-organ engineering [58–60].
1.2.3 Vascularization
Vascularization is a key factor in the successful outcomes of tissue regeneration. Tissue survival primarily depends on oxygen and nutrient supply from host blood vessels in vivo. Diffusion distance of oxygen and nutrients is generally 200 μm, so it is difficult for implanted cells that are more than 200 μm away from host vessels to survive [61–63]. Therefore, vascularization of volumetric, three-dimensional (3D) tissue constructs is necessary to the cells for a long-term survival. As such, several vascularization strategies have been investigated in engineered tissues such as the urethra [64], ureter [9], bone [65], cardiac tissue [66], skeletal muscle [67], skin [68], and lung [69], to overcome the current challenges associated with diffusion limitation.
A common approach for vascularization in TERM is to stimulate vascular ingrowth into the tissue constructs from adjacent host tissues. Angiogenic/vasculogenic growth factors, including vascular endothelial growth factor (VEGF), basic fibroblast growth factor (bFGF), and platelet-derived growth factor (PDGF), have been used to promote angiogenesis [63, 70]. VEGF has been widely used in tissue engineering applications in urology; for instance, VEGF-binding biomaterials were used to promote angiogenesis that resulted in tissue regeneration such as urethral and bladder tissues [71, 72]. Stem/progenitor cells have been implanted for inducing vascularization in the target tissues to be regenerated [73, 74]. These cells can release angiogenic factors or partly differentiate into vascular endothelial cells following implantation. Stem cells, vascular cells, and growth factors can be combined in various ways to achieve synergistic effects [70, 75, 76].
These strategies, however, would not be sufficient to form volumetric vascularized tissues of clinically relevant size. 3D scaffolds implanted in vivo take time to be fully vascularized, despite the use of angiogenic factors. This is due to the transient activity of factors that induce angiogenesis, and the rate of neovascularization is only ~5 μm/h [77]; thus, the angiogenic activity cannot be maintained long-term [63]. Consequently, the cells residing in the core region of the implanted scaffolds are unable to receive necessary oxygen and nutrients in a timely manner. To overcome the limitations associated with delayed vascularization within the 3D scaffolds, a strategy of pre-vascularization has been extensively investigated. This strategy involves in vitro as well as in situ formation of vascular structures within the scaffolds prior to implantation [78]. The rationale for this strategy is that preformed vascular structure would be readily integrated with the host vascular system following implantation, resulting in a rapid perfusion of blood into the implanted scaffolds. Recent advances in scaffold fabrication techniques, such as microfabrication and 3D printing, have enabled the development of 3D scaffolds that possess complex and perfusable vascular structures [79–81].
Another vascularization strategy is to facilitate vascularization by controlling the properties of microstructure of biomaterials. Highly controlled porous scaffolds facilitate the formation of vascular networks as well as enhancing supply of oxygen and nutrients [82]. The use of decellularized tissue scaffolds presents with an advantage due to the preservation of inherent vascular structures [69, 83]. While establishing adequate vascularization of 3D volumetric scaffolds still remains a challenge, continued development of innovative strategies using advanced techniques may lead to a solution that could accelerate the translation of various TERM therapies to patients.
1.2.4 Bioreactors
Bioreactors have been used in TERM to improve structural and functional maturation of engineered tissues in vitro. Bioreactors provide biomechanical forces such as compression, shear stress, and pulsatile flow under real-time monitored, highly controlled conditions [84, 85]. Bioreactors have been applied in a variety of tissues, including cardiovascular, musculoskeletal, skin, and bladder tissues [85–89]. In urology, bioreactors have been used to culture engineered tissues under the condition mimicking the filling pressures of bladder. For instance, human urothelial cells seeded on extracellular matrix scaffolds were exposed to cyclical filling pressures in the urinary bladder bioreactor system. This resulted in a significant increase of urothelial cell growth as compared with the static culture conditions [90]. In another example, 3D engineered muscle constructs were subjected to uniaxial cyclic bioreactors, which resulted in improved organization of unidirectional myotubes [91]. Bioreactors also have been used in cardiovascular tissue engineering to perfuse sufficient oxygen and nutrients to engineered tissue constructs [92]. In addition, preconditioning of cellular vascular grafts by a pulsatile bioreactor could maintain cellular viability and improve mechanical properties [93]. Recent advances in computer engineering, mechanical engineering, and materials science facilitated in designing of more sophisticated bioreactors that simulate physiological environments of tissues or organs [94]. With the advances in bioreactor technology, it is expected that its utility will be expanded to large, complex engineered tissue applications such as the kidney, liver, and heart.
1.2.5 New and Innovative Technologies for TERM Applications
Recent research works in the field of TERM have shown rapid technological evolutions that target clinical applications via multidisciplinary research. These technological evolutions reboost research areas that had previously been stagnated by innovative solutions and convergence technologies. Some of these include scaffold fabrication methods, monitoring and evaluating tools, modeling, and preservation. Utilization of these technologies will further enable the field to develop TERM therapies for complex tissue and organ systems.
3D printing, an additive manufacturing technology, is one of the most explored scaffold fabrication technologies currently in TERM [95]. It has become a powerful tool to fabricate clinically relevant 3D scaffolds and tissue constructs in a precise manner with high reproducibility. With this technology, precise fabrication is possible for complex tissue and organs of the human body as well as scaffolding tailored for the geometry of patients’ lesions. This technology also has been used to fabricate surgical models such as kidney, liver, bone, and cardiovascular tissues [96, 97]. In urology, 3D printing has been used to fabricate bladder scaffolds that are later covered with the patient’s own cells [98, 99]. Recently, 3D printing technology has rapidly improved so that various types of cells as well as biomaterials can be printed. 3D bioprinting technology has allowed for the fabrication of living tissues and organs having complex structures [99, 100]. Various 3D bioprinting methods, such as inkjet printing, microextrusion printing, and laser printing, have been explored [99]. These bioprinting modalities have been modified and combined in various ways to produce tissue constructs effectively. With the remarkable improvement of 3D bioprinting technology, 3D human-scale living tissues have been fabricated [101]. While 3D bioprinting technology holds promise as a tool to generate complex tissue constructs, several issues remain to be solved, including the development of clinically usable software and development of diverse bioinks as well as improvement of bioprinters that could be reliably used in the clinic. In the future, one can envision treating a patient with a tissue deformation. Medical data such as CT or MRI can be converted into a 3D CAD model to generate a patient-specific tissue construct using a bioprinter for immediate surgical reconstruction in the operating room.
Another research area that is gaining increasing attention is developing tools for monitoring and evaluation of TERM products. Histological analysis using sample tissues is the most common method to assess tissue implants. Animals are often sacrificed for tissue collections, and no further follow-up will be possible. To overcome this limitation, noninvasive high-resolution image acquisition techniques have been developed at the cell, molecular or tissue level [102]. These techniques allow researchers to collect accurate morphological and functional information without compromising an ongoing study. To this end, various cell tacking technologies and related tissue monitoring methods have been introduced to track implanted or injected cells [102]. Noninvasive magnetic resonance imaging (MRI) has been used to assess tissue functions associated with the movement of the body, hemodynamics, and metabolism [102, 103]. For instance, to monitor and evaluate stem cell homing and tissue regeneration effects in vivo, magnetic nanoparticle-absorbed mesenchymal stem cells were injected, and the cells were tracked with MRI [104]. Optical imaging techniques are also used in noninvasive real-time measurements of the tissue. With those tools, a series of tissue formation processes such as gene expression, differentiation, apoptosis, and vascularization from implantation to remodeling can be investigated [102, 103]. These monitoring methods progress in conjunction with the advancement of relevant nanoengineering, molecular biology, and computer science technologies. In the future, noninvasive image acquisition methods are expected to contribute to the analysis of tissue function in smaller scale, making it more reproducible, less invasive, faster, more precise, and economical.
Modeling technology is becoming increasingly important in TERM. 3D computer simulations of cell behaviors predict not only physical structures but also dynamic interactions between cells and environments. “BioSPICE” is open-source software to predict spatiotemporal behaviors of cells, and “cellular automata” simulate cellular dynamics, including migration and differentiation in two-dimensional (2D) space [105, 106]. These technologies might be essential in the design of improved tissue engineering products, as tissue development depends on cellular phenotypes and behavior patterns. In the near future, multi-scale modeling from the submolecular to the tissue level could be applied to provide a framework for bioreactor design and bioinspired tissue engineering constructs for TERM.
Cells and tissues’ preservation technology is essential in clinical applications and commercialization of TERM products. The most common technology used in TERM is cryopreservation. Successful cryopreservation protocols have been developed for different cell types with parameters that control cryopreservation speed and the type of cryoprotectant chemicals [107, 108]. While the goal of cryopreservation is to preserve cells for tissue fabrication of engineered tissue products, it is becoming increasingly popular for individuals to preserve reproductive tissues for potential infertility or chemotherapy. To this end, appropriate preservation facilities are required to preserve cells and tissues without loss of their fertility over a long period of time [109]. More recently, a testicular tissue preservation system has been established for utilizing spermatogonial stem cells, which follows good tissue practice (GTP) protocol of US Food Drug Administration [110]. Establishing preservation protocols for various cell types is crucial for the fabrication and commercialization of cellular composite tissue constructs.
1.3 Challenges and Future Perspectives
Over the past decades, a considerable research progress has been made to bring TERM technologies toward clinical translation. Consequently, several TERM products have been successfully translated to human clinical trials, some of which achieved favorable outcomes involving functional improvement. For instance, tissue-engineered tubularized urethras using autologous smooth muscle and epithelial cells were implanted into urethral defect sites, and that led to functional tissue restoration for up to 6 years in 5 patients [111]. In another example, various types of cells have been implanted in patients for corneal regeneration [112] and for treating myocardial infarction [59, 113, 114]. With the successful clinical translation of initial TERM therapies, it is expected that TERM research will continue to focus on developing engineered tissue constructs and therapies that can be translated to patients. However, several challenges still remain, and these must be addressed in order to bring more complex tissue therapies to the clinic [3, 4, 115].
One practical challenge that hampers clinical translation is building a clinically relevant-sized composite tissue constructs [98, 116]. In the clinical situation, composite tissue damage is more common rather than single tissue damage. For instance, a patient with severe facial trauma frequently has composite tissue injuries involving the skin, muscle, nerve, and bone, which should all be reconstructed for functional restoration. Considering the clinical relevance, current research should focus on developing implantable composite tissue constructs for patients. Accordingly, the composite tissue constructs should be able to combine at least two different tissue types in a single contiguous construct and mimic the structural and mechanical characteristics of each tissue type. However, fabricating composite tissue constructs remains a technological challenge. As previously noted, 3D bioprinting and decellularization/recellularization technologies have shown the potential of fabricating complex, composite, human-scale tissues [60, 101, 117]. In addition to these technologies, other fabrication methods that can produce composite tissue constructs need to be developed.
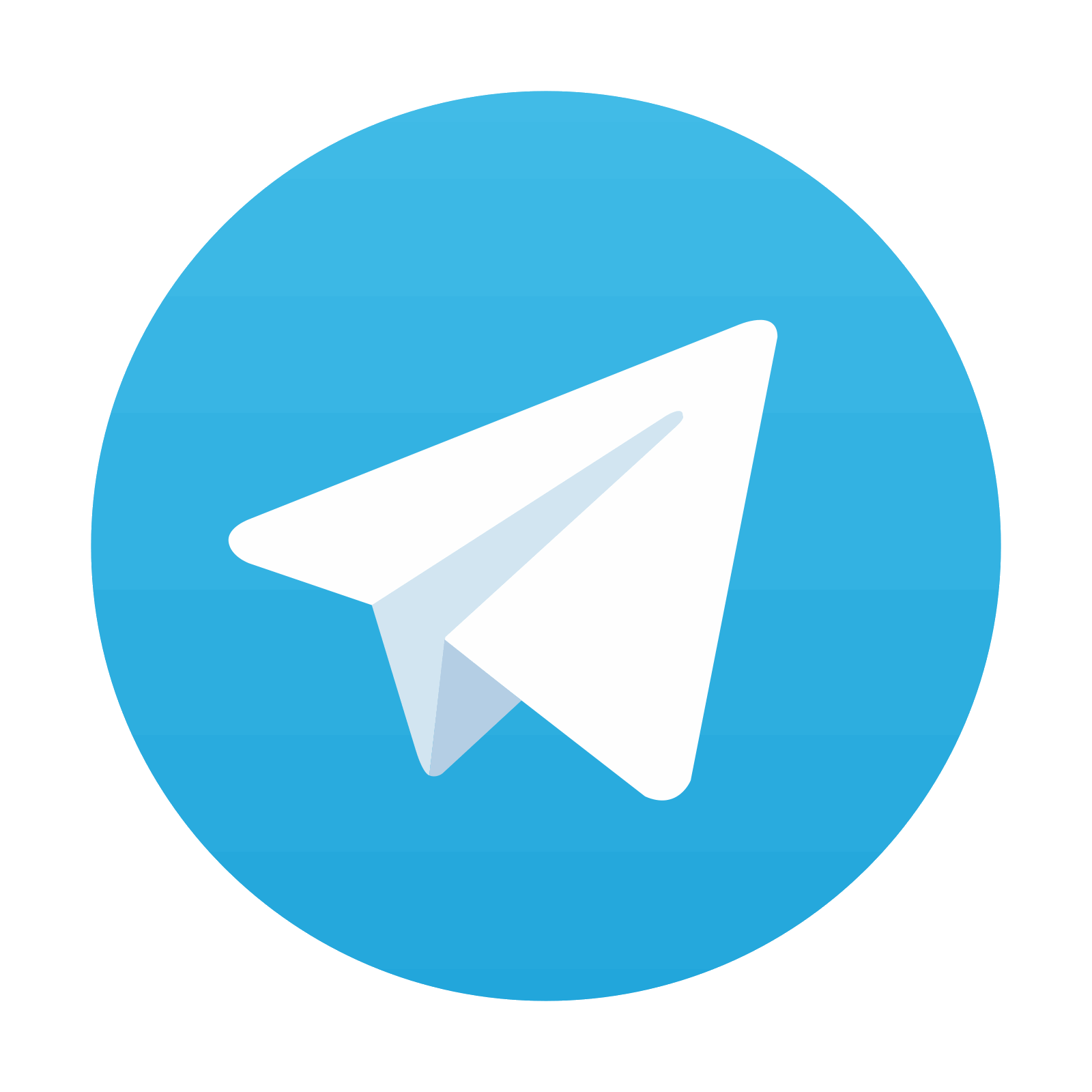
Stay updated, free articles. Join our Telegram channel
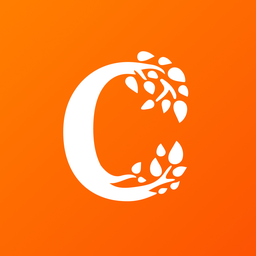
Full access? Get Clinical Tree
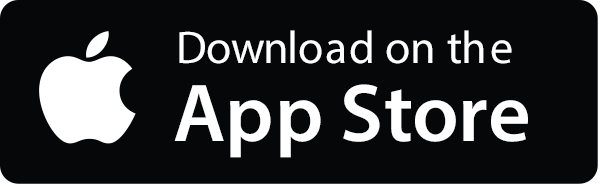
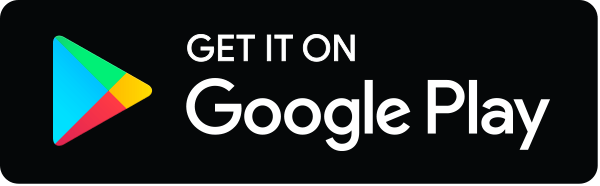