As surgical operative technology improves, surgeons today have the ability to visualize fine structures and detailed anatomy. There are a number of advances that have been made to optimize patient outcomes with better tissue characterization in urologic procedures. This article focuses on advances in intraoperative imaging and tissue characterization for various urologic procedures. Each modality is presented with its corresponding applications in urology. The following techniques are covered: optical coherence tomography, confocal fluorescent microscopy, near infrared fluorescence imaging, elastography, intraoperative ultrasonography, and a neurovascular bundle surgical mapping aid.
This article is not certified for AMA PRA Category 1 Credit ™ because product brand names are included in the educational content. The Accreditation Council for Continuing Medical Education requires the use of generic names and or drug/product classes as the required nomenclature for therapeutic options in continuing medical education.
For more information, please go to www.accme.org and review the Standards of Commercial Support.
As surgical operative technology improves, surgeons today have the ability to visualize fine structures and detailed anatomy. There are a number of advances that have been made to optimize patient outcomes with better tissue characterization in urologic procedures. This article focuses on advances in intraoperative imaging and tissue characterization for various urologic procedures. Each modality is presented with its corresponding applications in urology. The following techniques are covered: optical coherence tomography (OCT); confocal fluorescent microscopy (CFM); near-infrared fluorescence imaging; elastography; intraoperative ultrasonography (including Doppler, duplex flow, and high-resolution three-dimensional imaging); and the Cavermap neurovascular bundle (NVB) surgical mapping aid.
Optical coherence tomography
OCT is an imaging technique that provides real-time, high-resolution, atraumatic, cross-sectional imaging of tissues. One OCT system has been used in urologic applications (bladder cancer detection and staging and NVB mapping). The device is comprised of an 8F catheter fiberoptic probe with a computer console. The fiberoptic probe allows for it to be integrated into laparoscopic instruments or other flexible devices. OCT is similar to B-mode ultrasonography, but instead of measuring the backscattering of acoustic waves, it measures the backscattering of near-infrared light. Unlike ultrasound, however, OCT does not require direct probe contact with the tissue or a transducing medium, decreasing interference with the operative instruments in the surgical field. Image resolution as fine as 1 to 15 μm can be achieved with a maximal penetration depth of 1.6 mm, which allows for imaging of microscopic structures, such as the cavernous nerves, lymphatics, blood vessels, and fascial planes.
The superficial imaging characteristics of OCT make it ideally suited for possible assessment of depth of penetration of bladder cancer within the bladder wall. A number of groups have evaluated the use of OCT as an adjunct to conventional cystoscopy to assess better the stage and grade of bladder cancers. Specific attention has been paid to the use of OCT in assessing the depth of penetration of bladder tumors beyond the basement membrane (or lamina propria). Fig. 1 illustrates some representative images by Hermes and colleagues of OCT being used to assess bladder lesions (benign versus carcinoma in situ versus invasive carcinoma). At the present time, resection of these lesions is still performed, and so the added value of this imaging to current pathology evaluation may be questioned. The potential of using the OCT images in lieu of pathologic examination in terms of planning further treatment for these patients, however, is intriguing.
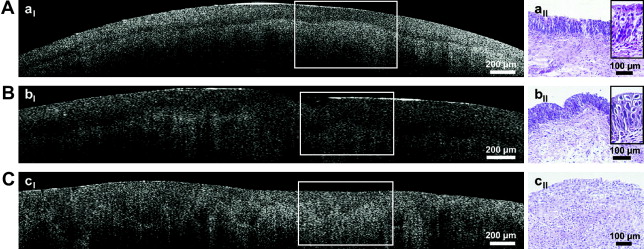
Rais-Bahrami and colleagues and Fried and colleagues demonstrated the use of OCT in imaging of the cavernous nerve and periprostatic tissue in the rat model. The rat model proved to be an ideal model for imaging because the cavernous nerve exists as a large, visible structure with minimal intervening vasculature or fat. To confirm the course of the cavernous nerve in the rat, the nerve was identified and stimulated with simultaneous intracorporeal pressure measurements and penile length and girth measurements taken. Hematoxylin-eosin–stained histologic specimens of the cavernous nerve and periprostatic tissues were then compared with the OCT images obtained from the same location on the prostate and were found to correlate well ( Fig. 2 ).
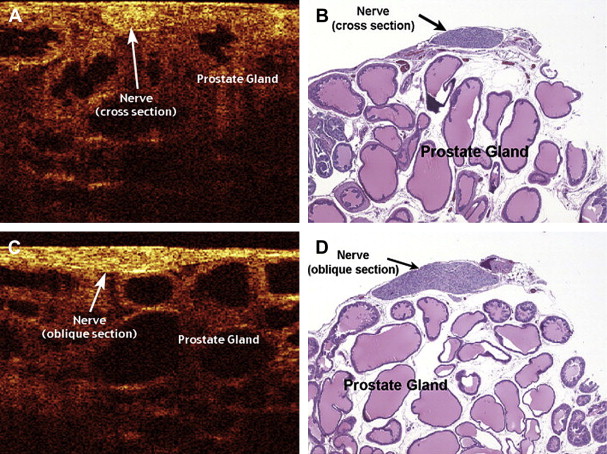
Rais-Bahrami and colleagues and Aron and colleagues both reported on the use of OCT to image human ex vivo prostatectomy specimens immediately after removal. Rais-Bahrami and colleagues found the OCT images of human cavernous nerve and prostatic tissue to be similar to that of the rat in that there was good histologic correlation with the OCT images. Identification of the NVB proved to be more difficult, however, compared with the rat prostate because of the presence of more periprostatic blood vessels and fat resulting in a degradation of the OCT resolution. Aron and colleagues also found that identification of the NVBs required an experienced operator to distinguish them from adipose tissue, small vessels, and lymphatics.
Although OCT has been used to aid in the intraoperative identification and preservation of the NVB, success has yet to be validated by potency results. Future improvements in the technology resulting in greater depth of penetration and resolution may make OCT more feasible for intraoperative identification of the cavernous nerve during nerve-sparing prostatectomies or for localization of cavernous nerve endings to allow for a precise nerve graft anastomosis.
There is also preliminary work on the use of OCT for testicular seminiferous tubule imaging. In men who have nonobstructive azoospermia, testicular sperm extraction is used to retrieve sperm to achieve pregnancies with assisted reproductive techniques. Unfortunately, sperm retrieval is only successful in about 50% to 60% of these cases. This has driven the development of “imaging-assisted” testicular sperm extraction to improve retrieval rates, using such techniques as microscopic assisted testicular sperm extraction and power Doppler ultrasound assisted guidance. OCT with its high-resolution images may improve sperm retrieval rates by better identifying isolated foci of spermatogenesis in these men with nonobstructive azoospermia.
Confocal fluorescent microscopy
CFM is a new technology that has been described for use in gastroenterology, dermatology, pulmonology, and urology and provides such incredibly detailed resolution that it allows for in vivo differentiation of cancerous and normal tissue. CFM can also provide enough resolution to allow distinction between some differentiated and undifferentiated cancers.
Koenig and colleagues have demonstrated the use of CFM for endoscopic microscopy of the bladder and opened the possibility of using this technology for real-time in vivo assessment of bladder lesions. CFM allowed imaging of cellular details of the entire urothelium. The superficial umbrella cells, intermediary and basal cells, capillary vessel network, and layers of the lamina propria could all be clearly identified similar to histologic sections of the tissue. Currently, the CFM system provides imaging only on horizontal tissue planes. With three-dimensional software reconstruction, however, the production of real-time three-dimensional noninvasive histologic imaging with flexible fibers that could be passed through the working channel of the cystoscope is likely to become available in the near future.
CFM has also been used to provide real-time in vivo images of peripheral nerves and deep-brain structures in rats genetically engineered to express a fluorescent protein in all sensory and motor neurons. CFM allows for enough spatial resolution to produce images down to the axonal level. A commercially available unit uses CFM technology, and is composed of a laser scanning unit, a small fiberoptic probe, and an image processing unit. Real-time images can be produced up to 12 frames per second, and the probe allows for a lateral resolution of 3.5 μm with a depth of penetration of 15 μm. Compared with such technologies as ultrasonography or OCT, CFM requires the use of a fluorescent dye applied and diffused within the tissue being studied to help resolve, differentiate, and augment tissue characteristics.
Boyette and colleagues have used CFM technology along with injection of a fluorescent retrograde nerve tracer to provide in vivo real-time images of the cavernous nerve in rats. A recombinant β subunit of cholera toxin conjugated to a fluorescent compound served as the fluorescent nerve tracer and was injected into the corpus cavernosum of male rats to allow for retrograde transport along the cavernous nerves. They found that optimal imaging of the cavernous nerve was obtained after allowing for 9 days of retrograde transport along the nerve. The cavernous nerves were then exposed and imaged using CFM, which produced exceptional images, even allowing for visualization of the branching of the cavernous nerve ( Fig. 3 ). Confirmation that the nerves being imaged were actually the cavernous nerve was obtained by electrical stimulation of the fluorescent nerve with simultaneous intracavernosal pressure monitoring.
Although CFM technology produces striking images in the rat model, there are limitations to the technology that need to be overcome before successful application in humans. The depth of penetration of the probe is only 15 μm, which works well for the rat model. As was encountered in translating OCT technology from the rat to human model, however, the greater amount of periprostatic fat, vessels, and lymphatics in the human model may hinder the view of structures, such as the cavernous nerve. Another major limitation to the technology is the need for injection of a fluorescent nerve tracer and waiting for retrograde transport to the cavernous nerve. Although transport in the rat model took 9 days, it has been estimated that it may take up to 45 days for optimal imaging to be obtained in the human because of the four to five times greater distance from the penis to the cavernous nerve the tracer has to travel. Finally, although short-term safety of the injected tracer composed of the β subunit of cholera toxin was demonstrated in the rat model, long-term studies need to be performed in humans before the technology can become mainstream.
CFM has also been used to detect DNA abnormalities within spermatozoa in a noninvasive manner. This again brings about the possibility of using such imaging technology in men with nonobstructive azoospermia to identify areas within the testicle that may harbor pockets of spermatogenesis. The depth of penetration for imaging may be a limiting factor in truly performing a noninvasive scanning of the entire testicle, but as the technology improves, there are likely to be further applications for this modality.
Confocal fluorescent microscopy
CFM is a new technology that has been described for use in gastroenterology, dermatology, pulmonology, and urology and provides such incredibly detailed resolution that it allows for in vivo differentiation of cancerous and normal tissue. CFM can also provide enough resolution to allow distinction between some differentiated and undifferentiated cancers.
Koenig and colleagues have demonstrated the use of CFM for endoscopic microscopy of the bladder and opened the possibility of using this technology for real-time in vivo assessment of bladder lesions. CFM allowed imaging of cellular details of the entire urothelium. The superficial umbrella cells, intermediary and basal cells, capillary vessel network, and layers of the lamina propria could all be clearly identified similar to histologic sections of the tissue. Currently, the CFM system provides imaging only on horizontal tissue planes. With three-dimensional software reconstruction, however, the production of real-time three-dimensional noninvasive histologic imaging with flexible fibers that could be passed through the working channel of the cystoscope is likely to become available in the near future.
CFM has also been used to provide real-time in vivo images of peripheral nerves and deep-brain structures in rats genetically engineered to express a fluorescent protein in all sensory and motor neurons. CFM allows for enough spatial resolution to produce images down to the axonal level. A commercially available unit uses CFM technology, and is composed of a laser scanning unit, a small fiberoptic probe, and an image processing unit. Real-time images can be produced up to 12 frames per second, and the probe allows for a lateral resolution of 3.5 μm with a depth of penetration of 15 μm. Compared with such technologies as ultrasonography or OCT, CFM requires the use of a fluorescent dye applied and diffused within the tissue being studied to help resolve, differentiate, and augment tissue characteristics.
Boyette and colleagues have used CFM technology along with injection of a fluorescent retrograde nerve tracer to provide in vivo real-time images of the cavernous nerve in rats. A recombinant β subunit of cholera toxin conjugated to a fluorescent compound served as the fluorescent nerve tracer and was injected into the corpus cavernosum of male rats to allow for retrograde transport along the cavernous nerves. They found that optimal imaging of the cavernous nerve was obtained after allowing for 9 days of retrograde transport along the nerve. The cavernous nerves were then exposed and imaged using CFM, which produced exceptional images, even allowing for visualization of the branching of the cavernous nerve ( Fig. 3 ). Confirmation that the nerves being imaged were actually the cavernous nerve was obtained by electrical stimulation of the fluorescent nerve with simultaneous intracavernosal pressure monitoring.
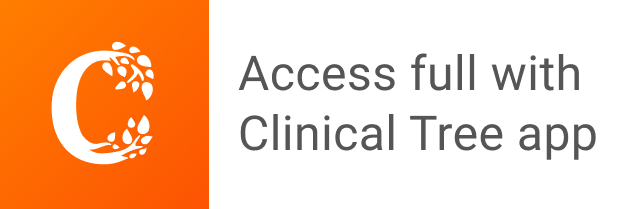