INTRODUCTION
Every physician and physician in training must master the ability to use intravenous solutions for expansion of the intravascular and ECF volume. Proper understanding of solutions available (colloid vs. crystalloid), their space of distribution, their cost and potential adverse effects, as well as an assessment of the patient’s volume status are essential for their proper use. Mistakes are made when there is improper understanding of the patient’s volume and electrolyte status.
Hypovolemia is a common problem in hospitalized patients, especially those in critical care units. It can occur in a variety of clinical settings including those characterized by obvious fluid loss as with hemorrhage or diarrhea, as well as in patients without obvious fluid loss as a result of vasodilation with sepsis or anaphylaxis. In one study, inadequate volume resuscitation was viewed as the most common management error in patients that died in the hospital after admission for treatment of injuries.
UNDERSTANDING BODY FLUID COMPARTMENTS
Total-body water constitutes 60% of lean-body weight in men and 50% of lean-body weight in women. It is distributed between intracellular fluid (ICF) (66.7%) and ECF (33.3%) compartments (Figure 5.1). The ECF compartment is further subdivided into intravascular and interstitial spaces. Twenty-five percent of the ECF compartment consists of the intravascular space, with the remaining 75% constituted by the interstitial space.
FIGURE 5-1. Body fluid compartments. Total-body water consists of ICF and ECF. ICF is 40% of lean-body weight and ECF is 20% of lean-body weight. The major driving force for fluid movement between these compartments is osmosis. The ECF can be further subdivided into the intravascular and interstitial spaces that constitute 5% and 15% of total-body weight, respectively. Starling forces are the major driving force for fluid movement between these compartments.
Osmotic forces govern the distribution of water between ICF and ECF. The ECF and ICF are in osmotic equilibrium, and if an osmotic gradient is established, water will flow from a compartment of low osmolality to a compartment of high osmolality. For example, if a solute is added to the ECF such as glucose that raises its osmolality, water will flow out of the ICF until the osmotic gradient is dissipated. Water movement into and out of cells, particularly in brain, with resultant cell swelling or shrinking is responsible for the symptoms of hyponatremia and hypernatremia.
Urea distributes rapidly across cell membranes and equilibrates throughout total-body water and is, with one exception, an ineffective osmole. Equilibration of urea across the blood–brain barrier can take several hours and in this circumstance urea may function as a “transiently effective” osmole. If urea is rapidly removed from the ECF with initiation of hemodialysis in a patient with end-stage renal disease, the potential exists for the development of “dialysis disequilibrium syndrome.” Patients at increased risk are those with a blood urea nitrogen (BUN) greater than 100 mg/dL that have rapid rates of urea removal during their first or second hemodialysis session. As urea concentration falls during hemodialysis a transient osmotic gradient for water movement into brain is established. This results in headache, nausea, vomiting, and, in some cases, generalized seizures. Dialysis disequilibrium can be minimized by initiating hemodialysis with low blood flow rates and for short periods of time.
Each compartment has one major solute that acts to hold water within it: ECF—sodium salts; ICF—potassium salts; and intravascular space—plasma proteins. It is important to appreciate that the serum sodium concentration is a function of the ratio of the amounts of sodium and water present and does not correlate with ECF volume, which is a function of total-body sodium. This is illustrated by the 3 examples discussed below in which ECF volume is increased in all 3 cases but serum sodium is high, low, and normal.
If one adds NaCl to the ECF, it remains within the ECF increasing its osmolality resulting in water movement out of cells. Equilibrium is characterized by hypernatremia, an increase in ECF osmolality (NaCl addition), and ICF osmolality (water loss). As a result ECF volume increases and ICF volume decreases. Therefore, even though sodium is restricted to the ECF, its administration results in an increase in osmolality of both ECF and ICF, and a reduction in ICF volume. The osmolar effects of NaCl administration are distributed throughout total-body water even though NaCl is confined to the ECF. If one adds 1 L of water to the ECF there is an initial fall in ECF osmolality, promoting water movement into cells. Equilibrium is characterized by hyponatremia and an expansion of both ECF and ICF volumes. One-third of the water remains in the ECF and only 8% in the intravascular space.
Finally, if one adds 1 L of isotonic saline to the ECF, the saline is confined to the ECF and it will increase by 1 L. The intravascular volume will increase by 250 mL. Because there is no change in osmolality there is no shift of water between the ECF and ICF and serum sodium concentration remains unchanged.
Starling forces govern movement of water between intravascular and interstitial spaces (Figure 5.2). Expansion of the interstitial space results in the clinical finding of edema. Edema fluid resembles plasma in electrolyte content, although its protein content may vary. The interstitial space must be expanded by 3 to 5 L before edema in dependent areas is detected. Edema may be localized as a result of vascular or lymphatic injury, or it may be generalized as in congestive heart failure (CHF). Forces governing edema formation are summarized by the equation below in which Kc reflects the surface area and permeability of the capillary. LR is the lymphatic return. Pc and Pt are the hydrostatic pressures in the capillary and tissue, respectively, whereas πc and πt are the oncotic pressure in the capillary and tissue, respectively.
FIGURE 5-2. Starling forces across the capillary bed. Starling forces that move fluid out of the capillary are intravascular hydrostatic pressure (most important) and interstitial oncotic pressure. Forces acting to move fluid into the capillary are the intravascular oncotic pressure (most important) and interstitial hydrostatic pressure. Fluid in the interstitial space drains back to the venous system via lymphatics.
Net accumulation = Kc × [(Pc – πc) – (Pt – πt)] – LR
The most common abnormalities leading to edema formation are an increase in capillary hydrostatic pressure or a decrease in capillary oncotic pressure. In CHF, for example, the Pc increases. In cirrhosis, the Pc increases (secondary to portal hypertension) and the πc declines. Table 5.1 lists the specific causes of edema, classified according to the major mechanism(s) responsible. The final common pathway maintaining generalized edema is renal retention of excess sodium and water.
TABLE 5-1. Mechanism of Edema Formation
KEY POINTS
REPLACEMENT OPTIONS: COLLOID VERSUS CRYSTALLOID
Despite the fact that adequate volume replacement is essential in the management of critically ill patients, the optimal replacement fluid remains a focus of considerable debate. Clinicians can choose between a wide array of crystalloids and colloids. Crystalloid solutions consist of water and dextrose and may or may not contain other electrolytes. Their composition varies depending on the type of solution. Some of the more commonly used crystalloid solutions and their components are shown in Table 5.2, and include 5% dextrose in water (D5W), normal saline (0.9%), one-half normal saline (0.45%), and Ringer’s lactate. Ringer’s lactate is used more commonly in surgical services and normal saline in medical services.
TABLE 5-2. Commonly Used Crystalloid Solutions
Colloid solutions consist of high-molecular-weight molecules such as proteins, carbohydrates, or gelatin. Colloids increase osmotic pressure and remain in the intravascular space longer compared to crystalloids. Osmotic pressure is proportional to the number of particles in solution. Colloids do not readily cross normal capillary walls and result in fluid translocation from interstitial space to intravascular space.
Colloids are referred to as monodisperse, like albumin, if the molecular weight is uniform, or polydisperse, if there is a range of different molecular weights, as with starches. This is important because molecular weight determines the duration of colloidal effect in the intravascular space. Lower-molecular-weight colloids have a larger initial oncotic effect but are rapidly renally excreted and, therefore, have a shorter duration of action. Hydroxyethyl starch (HES), dextran, and albumin are the most commonly used colloids. Gelatins are not commercially available in the United States.
HES is a glucose polymer derived from amylopectin. Hydroxyethyl groups are substituted for hydroxyl groups on glucose. The substitution results in slower degradation and increased water solubility. Naturally occurring starches are degraded by circulating amylases and are insoluble at neutral pH. HES has a wide molecular weight range. Duration of action is dependent on rates of elimination and degradation. Lower-molecular-weight species are eliminated rapidly by the kidney. The rate of degradation is determined by the degree of substitution (the percentage of glucose molecules having a hydroxyethyl group substituted for a hydroxyl group). Substitution occurs at positions C2, C3, and C6 of glucose, and the location of the hydroxyethyl group also affects the degradation rate. Characteristics associated with a longer duration of action include higher molecular weight, a high degree of substitution, and a high C2:C6 ratio.
Hetastarch is a HES with a high molecular weight (480 kDa), slow elimination kinetics, and is associated with an increase in bleeding complications after cardiac and neurosurgery. The higher the molecular weight and the slower the elimination rate, the more likely that HES will cause clinically significant bleeding. Newer HES preparations with lower molecular weights and more rapid elimination kinetics may be associated with fewer complications. Hetastarch use is also associated with an increased risk of acute kidney injury (AKI) in septic patients and in brain-dead kidney donors. Several randomized controlled trials and a recent systematic review showed that HES increases the risk of AKI in septic patients. In one study, the median time to AKI was 16 days. The incidence of AKI is increased with increases in the cumulative dose. Given these findings, hetastarch cannot be recommended in patients with impaired kidney function. The threshold level of glomerular filtration rate below which hetastarch should be avoided is unknown. Table 5.3 compares albumin to hetastarch. Hetastarch is available as a 6% solution in normal saline. One liter of hetastarch will initially expand the intravascular space by 700 to 1000 mL. Other commercially available HES preparations include Voluven, Hextend, and Hespan.
TABLE 5-3. Albumin Versus Hetastarch
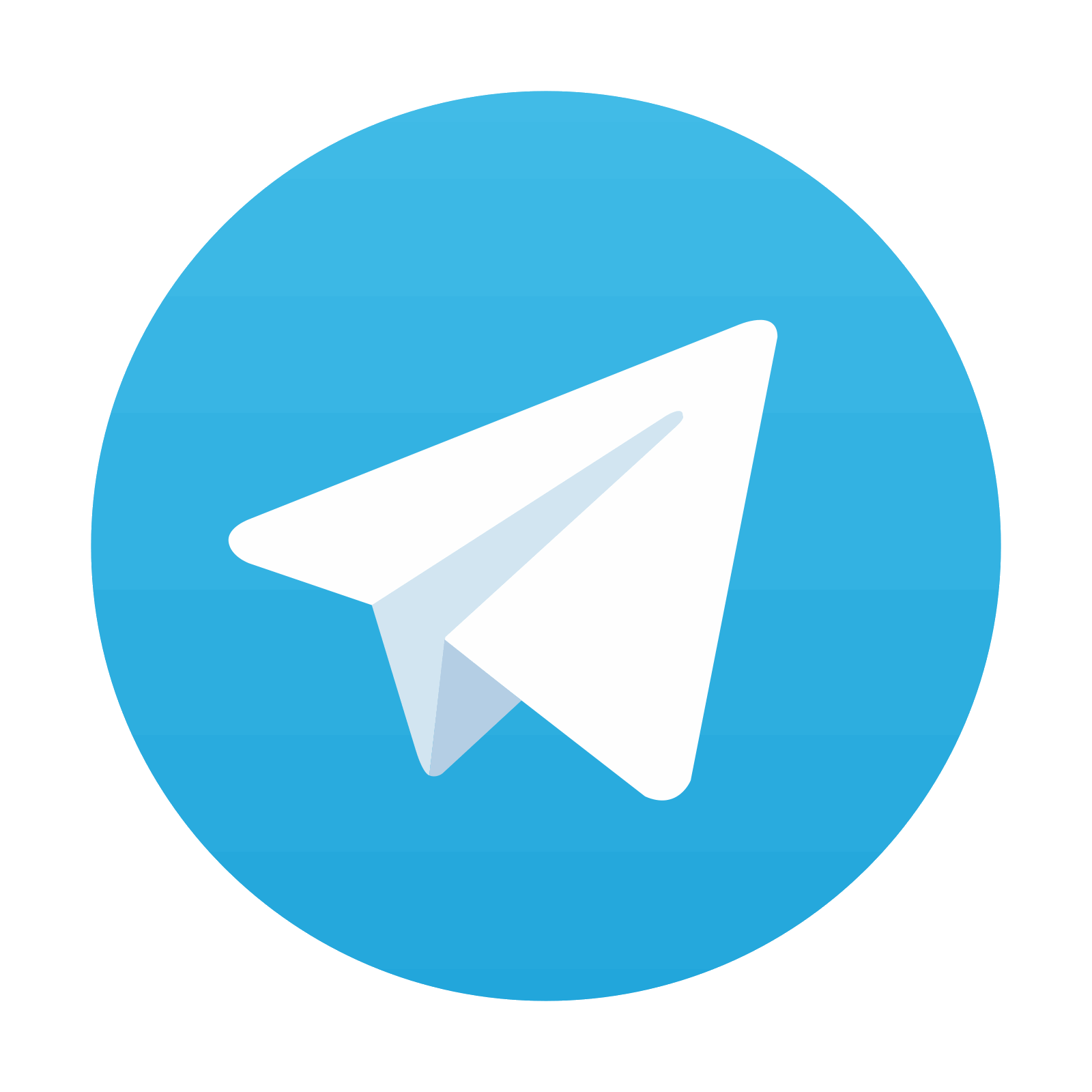
Stay updated, free articles. Join our Telegram channel
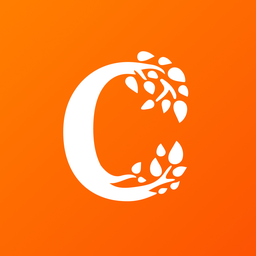
Full access? Get Clinical Tree
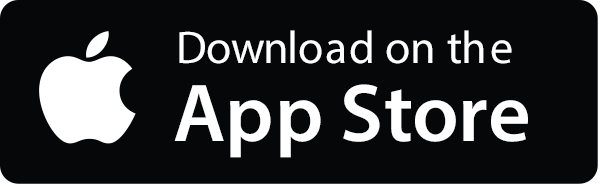
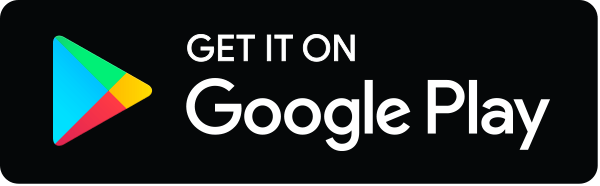