RESPIRATORY DISTURBANCES
Introduction
Breathing is an automatic, rhythmic, and centrally regulated process by which contraction of the diaphragm and rib cage moves gas in and out of the airways and alveolae of the lungs. Respiration includes breathing, but it also involves the circulation of blood, allowing for O2 intake and CO2 excretion.
Two patterns are involved in the control of breathing: automatic and volitional. The automatic component is largely under the control of partial pressure of carbon dioxide (PCO2). The control center for this breathing resides in the brainstem within the reticular activating system (Figure 9.1). There are 2 major regions that control automatic ventilation: the medullary respiratory areas and the pontine respiratory group. Interestingly, less is known about volitional control than automatic control of respiration; consequently, we restrict our discussion to automatic breathing.
FIGURE 9-1. Control of ventilation. Schematic illustrating that central control of ventilation is largely through partial pressure of arterial carbon dioxide (PaCO2)-sensitive chemoreceptors in the pons and medulla, whereas peripheral input is largely through partial pressure of arterial oxygen (PaO2)-sensitive chemoreceptors in the carotid body. Output is to the diaphragm via the phrenic nerves and thoracic muscles largely via intercostal innervations.
Two main types of chemoreceptors—central and peripheral—are involved in the control of automatic breathing. The most important ones are located in the medulla of the central nervous system (CNS). The main peripheral chemoreceptors are within the carotid bodies, although less-important receptors were identified in the aortic arch. Central chemoreceptors respond to changes in partial pressure of arterial carbon dioxide (PaCO2) largely through changes in brain pH (interstitial and cytosolic). This is a sensitive system, and PaCO2 control is generally tight. In contrast, respiratory control by oxygen tensions is much less important until partial pressure of arterial oxygen (PaO2) falls to levels below 70 mmHg. This is a result of the hemoglobin-oxygen (Hb-O2) dissociation curve, as Hb saturation is generally above 94% until the PaO2 falls below 70 mmHg. O2 control of respiration is mediated largely through peripheral chemoreceptors which, in response to low O2, close adenosine triphosphate (ATP)-sensitive K+ channels and depolarize glomus cells in the carotid body. The 2 systems interact, in that, with hypoxia, the central response to PCO2 is enhanced. As we discuss later, with chronic hypercapnia, control of respiration by CO2 is severely blunted, leaving some patients’ respiration almost entirely under the control of O2 tensions.
In addition to neural control, the physical machinery of breathing is also extremely important in gas exchange. This physical machinery involves the lungs, bones, and the thorax musculature that interact to move air in and out of the pulmonary air spaces. Just as there may be neural defects that impair respiration, abnormalities of the skeleton, musculature, airways, air spaces, or lung blood supply may impair respiration. To some degree, these abnormalities are assessed and characterized by pulmonary function tests. Although it is beyond the scope of this chapter to discuss this topic in detail, it should be clear to the reader that modern pulmonary function tests readily differentiate problems with airway resistance (eg, asthma or chronic obstructive pulmonary disease) from those of alveolar diffusion (eg, interstitial fibrosis) or neuromuscular function (eg, phrenic nerve palsy, Guillain-Barré syndrome). Figure 9.1 shows a simplified schematic of the elements involved in controlling ventilation.
Pulmonary ventilation refers to the amount of gas brought into and/or out of the lung. Pulmonary ventilation is expressed as minute ventilation (ie, how much air is inspired and expired within 1 minute) or in functional terms as alveolar ventilation (VA). The difference between these terms occurs because the portion of ventilation confined to the conductance airways does not effectively exchange O2 for CO2 in alveolae. Because O2 uptake and CO2 excretion are so critical, we can reference ventilation with regard to either of these gases. However, because CO2 excretion is so effective and ambient CO2 tensions in the atmosphere are so low, pulmonary ventilation generally is synonymous with pulmonary CO2 excretion. Note that CO2 is much more soluble than O2 and exchange across the alveolar capillary for CO2 is essentially complete under most circumstances, whereas some O2 gradient from alveolus to the alveolar capillary is always present.
We should also point out that ventilation occurs at the tissue level as well. In this case, rather than inspired air removing CO2 in its gaseous form, CO2 produced by cells is largely (approximately 75%) converted to bicarbonate () and removed from the local cellular environment by blood flow. Although it is an extreme case, when CO2 tensions in expired gases are monitored during cardiac arrest, the institution of effective circulation is accompanied by a sharp increase in expired CO2.
KEY POINTS
RESPIRATORY ACIDOSIS
Respiratory acidosis is defined as a primary increase in PaCO2 secondary to decreased effective ventilation with net CO2 retention. This decrease in effective ventilation can occur from defects in any aspect of ventilation control or implementation. Table 9.1 summarizes these different causes.
TABLE 9-1. Causes of Respiratory Acidosis
Compensation for respiratory acidosis occurs at several levels. Some of these processes are rapid, analogous to what is seen with major compensatory mechanisms for metabolic acidosis or alkalosis, whereas others are slower. This allows us to clinically distinguish between acute and chronic respiratory acidosis in some cases.
With respiratory acidosis, a rise in bicarbonate concentration [] is a normal, compensatory response. As is the case for metabolic disorders, a failure of this normal adaptive response is indicative of the presence of metabolic acidosis in the setting of a complex or mixed acid–base disturbance. Conversely, an exaggerated increase in
producing a normal pH indicates the presence of metabolic alkalosis in the setting of a complex or mixed acid–base disturbance.
Mechanisms by which respiratory acidosis increases concentration are as follows. First and probably foremost, increases in PaCO2 and decreases in O2 tension stimulate ventilatory drive, antagonizing the process that led to CO2 retention in the first place. Next, mechanisms by which CO2 transport occurs from tissues to lungs become operant. In other words, increases in PaCO2 are immediately accompanied by a shift to the right of the reaction:
and increases in concentration result. The amount of this increase in [
] in mEq/L is 0.1 times the increase in PaCO2 in mmHg (±2 mEq/L). The kidney provides the mechanism for the majority of chronic compensation. Once PaCO2 increases and arterial pH decreases, renal acid excretion and retention of bicarbonate become more avid. Some of this is a direct chemical consequence of elevated PaCO2 and mass action facilitating intracellular bicarbonate formation. Other portions involve genomic adaptations of tubular cells involved in renal acid excretion. On this latter topic, enzymes involved in renal ammoniagenesis (eg, glutamine synthetase), as well as apical and basolateral ion transport proteins (eg, Na+ -H+ exchanger, Na+-K+-adenosine triphosphatase [ATPase]) are synthesized in increased amounts at key sites within the nephron. In sum, chronic respiratory acidosis present for at least 4 to 5 days will be accompanied by a [
] increase equal to 0.4 times the increase in PaCO2 (mmHg) (±3 mEq/L). Note that renal correction also never completely returns the arterial pH to the level it was at prior to CO2 retention.
KEY POINTS
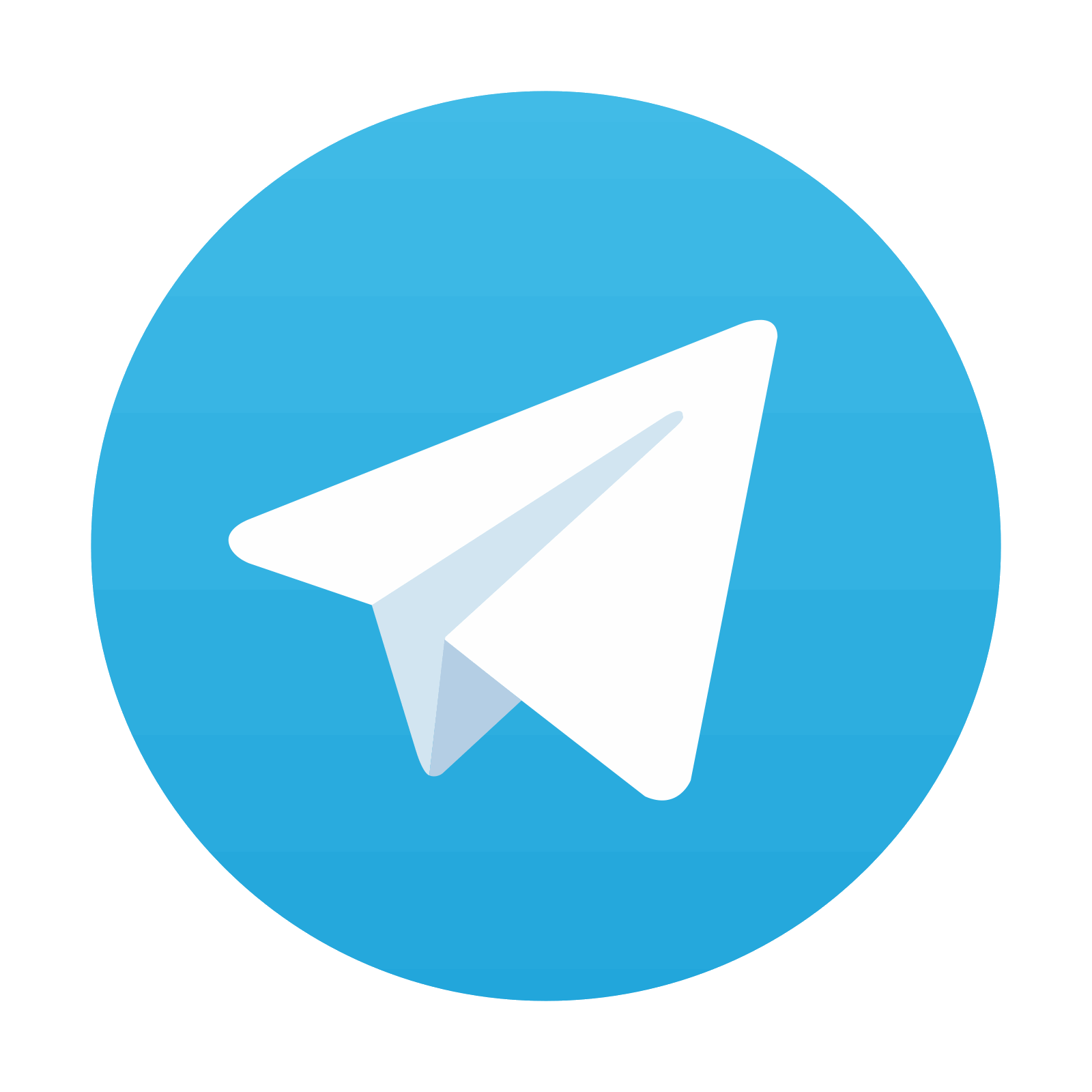
Stay updated, free articles. Join our Telegram channel
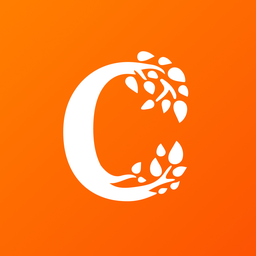
Full access? Get Clinical Tree
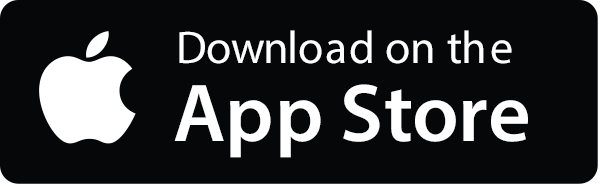
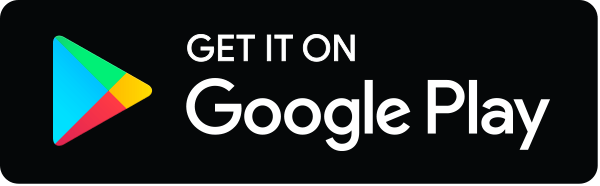