EPIDEMIOLOGY OF POISONING
Although poisoning has been reported to health care institutions for a long time, its incidence is clearly underestimated. The incidence of poisoning continues to increase largely due to abuse of over-the-counter medications as well as illicit drugs. Poisoning became the leading cause of accidental death in the United States in 2008, exceeding motor vehicle accident deaths for the first time since 1980 (1). In 2014, the Toxic Exposure Surveillance System (TESS) data compiled by the American Association of Poison Control Centers (AAPCC) revealed that there were almost 2.3 million reported human poison exposure cases. Over 600,000 exposures led to a visit in a health care facility (HCF), 27% of them required hospital admission resulting in 1,261 deaths in the United States (2). The majority (80%) of these cases were unintentional, but suicidal intent was suspected in 10.5% of cases. Poisoning may also result from malicious intent, drug–drug interaction (12.5%), or from occupational or environmental exposure. The top five substance classes most frequently involved in all human exposures are analgesics (11.6%), cosmetics/personal care products (7.9%), household cleaning substances (7.2%), sedatives/hypnotics/antipsychotics (6.0%), and antidepressants (4.1%) (2). Analgesics and sedative/hypnotics/antipsychotics exposures as a class increased most rapidly over the last 13 years for cases showing more serious outcomes. The National Poison Data System (NPDS) documented 2,477 human exposures resulting in death of which 2,113 human fatalities judged related. Again, analgesics were the most common cause of death, and acetaminophen is the analgesic most often implicated in fatal poisoning. Children younger than 3 years were involved in 35.5% of exposures and children younger than 6 years accounted for approximately half of all human exposures (48.0%). Male predominance was found among cases involving children younger than 13 years, but this gender distribution was reversed in teenagers and adults, with females comprising the majority of reported exposures. Most human exposures (87.9%) were acute cases (single, repeated, or continuous exposure occurring over 8 hours or less). The route of exposure to the poison was ingestion in 83.4% of cases followed in frequency by dermal (7.0%), inhalation/nasal (6.1%), and ocular routes (4.3%). Of the 2,188,013 human exposures reported, 71.8% of calls originated from a residence (own or other), but 93.5% actually occurred at a residence. Another 20.3% of calls were made from an HCF. Beyond residences, exposures occurred in the workplace in 1.6% of cases, schools (1.3%), health care facilities (0.3%), and restaurants or food services (0.2%) (2).
Most cases reported to poison centers were managed in a non-HCF (68.7%), usually at the site of exposure, primarily the patient’s own residence. Treatment in an HCF was rendered in 27.5% of cases. Of these, 16.5% were admitted for critical care, and 11.2% were admitted to a noncritical unit. Older age-groups exhibit a greater number of severe medical outcomes. Overall, the mortality rate was 0.11% of all exposures but much higher in hospitalized patients with suicidal overdose, who account for 32% of all deaths. In most of the fatal cases in adolescents and adults, poisoning was intentional. Because most poisoning fatalities occur before the patients reach an HCF, it is probable that the total number of fatalities resulting from poisoning is higher than that gathered by the AAPCC report. Extracorporeal treatments were used in 2,385 cases of poisoning; 97.5% of these were hemodialysis and 2.6 % were hemoperfusion (2).
The approach to diagnosis and treatment of poisoned individuals is usually divided into six stages. These include stabilization, laboratory assessment, decontamination of the gastrointestinal tract, administration of specific antidote if available, enhancing the elimination of the offending agent, and disposition. In this chapter, I will focus on the appropriate use of extracorporeal methods of elimination of common types of poisons.
PRINCIPLES OF MANAGEMENT OF POISONING
Diagnosis of Poisoning
In order to establish the correct diagnosis of poisoning, a systematic approach to the management of poisoned individuals must be followed. This should include careful history, physical examination, routine, and toxicologic laboratory evaluations (FIGURE 36.1). Moreover, particular attention should be paid to the clinical course of the patient’s condition. The history is clearly of critical importance and should include the time, route of administration, duration, and intent of exposure. The exact name and amount of the drug, chemical, or ingredient involved; the time of onset, nature, and severity of symptoms; and the medical and psychiatric history should also be obtained. Unfortunately, it may not be possible to obtain this information from the victim who may be confused, comatose, unable, or unwilling to admit to self-poisoning. Under these circumstances, relevant history may be obtained from family or friends, paramedics, police, or other physicians. Sometimes, a search of the patients’ belongings and residence may reveal a suicidal note or a container of drugs or chemical. Of critical importance is to establish, if possible, the timing of the exposure since this may have implications on the onset of symptoms and the patient’s clinical course. It is also important to establish the reason behind the exposure and whether the exposure was a suicide attempt (3). Physical examination should focus initially on the vital signs, cardiopulmonary system, rate and depth of breathing, and neurologic status. Moreover, examination of the eyes for nystagmus, pupil size, and reaction; abdomen for bowel activity; and skin for color, dryness, temperature, and presence of medication patches may reveal findings of diagnostic value. It is also crucial to perform frequent reexamination since the physical findings may change rapidly and patients may abruptly deteriorate. Routine laboratory tests should include complete blood count, chemistries, and arterial blood gases. Other blood tests should be obtained for suspected drugs or toxins in which blood concentration will not only help to confirm exposure but also help to predict toxicity and guide the approach for definitive therapy (FIGURE 36.1).
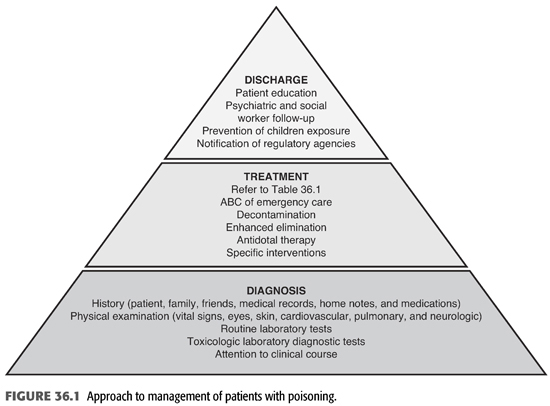
General Treatment Measures
Administration of appropriate treatment for the patient with poisoning should be done without delay (TABLE 36.1). In fact, diagnosis and treatment may have to be done sequentially or simultaneously, depending on the patient’s clinical course. Aggressive supportive care is of utmost importance and should follow the ABCs of emergency care; airway, breathing, and circulation for all poisoned victims in order to ensure adequate respiration; stable hemodynamics; and maintenance of fluid, electrolyte, and acid–base balance. These supportive measures have been shown to reduce mortality and morbidity to very low levels (3–6). The recognition of the critical role of supportive care in the treatment of poisoning has led to important changes in our approach to managing poisoning. First, abandonment of the use of stimulants (when the offending agent is a sedative). Second, reduced emphasis on the use of antidotes, except in a few special types of intoxications for which specific antidotes are available (TABLE 36.2). Third, decontamination which refers to preventing or reducing absorption of the suspected substance has also evolved over time. Gastric lavage, ipecac or cathartics, once mainstay of therapy of poisoning, have been questioned and deemed inappropriate since they may increase rather than reduce absorption and may even be harmful (7–12). However, administration of activated charcoal in an awake patient with intact airways may be used to enhance the elimination of ingested toxins (13). This adsorbent can be administered by mouth or by nasogastric intubation, preferably within 1 hour of ingestion and even before the identity of the toxic agent is known because of its capacity to bind a wide variety of toxins. However, activated charcoal does not bind lithium, heavy metals, or toxic alcohols. Multiple-dose activated charcoal (MDAC) can be administered over several hours in an attempt to enhance the elimination of a substance, such as carbamazepine and theophylline (3,14). By virtue of interrupting these toxin’s enterohepatic circulation, activated charcoal is effective even when the toxin is no longer present in the stomach or, as in the case of theophylline, when the toxin has already been systemically absorbed. Fourth, use of “forced diuresis” which entails administration of large fluid volume coupled with a diuretic agent to ensure a urine flow rate of 3 to 6 mL/kg/h has been largely abandoned. This approach was previously popular because of the belief that it would accelerate the elimination of most intoxicants through the kidney. However, given the lack of evidence for the efficacy of forced diuresis in treatment of poisoning on the one hand, and its association with significant risk of volume overload, pulmonary edema, and electrolyte abnormalities on the other, forced diuresis is no longer recommended in the management of patients with suspected poisoning. Finally, alkalinization of the urine is employed to promote the elimination of toxins that are weak acids in patients who have adequate urine output and normal renal function. The basis for this approach is that renal tubular cell membranes are more readily permeated by the lipid-soluble nonionized form of the drug in question. Thus, increasing the urine pH will lead to increasing the ionized fraction of the substance which is lipid insoluble and thus cannot be reabsorbed and will be trapped in the renal tubular lumen and be excreted in the urine. Urine alkalinization is achieved by the administration of one ampule of sodium bicarbonate as a bolus followed by dextrose 5% in water (D5W) to which 100 to 150 mEq of sodium bicarbonate is added to each liter and infused at 150 to 250 mL/h. The goal is to have a urine pH >7.5 (15,16). This degree of urinary alkalinization was thought to be helpful in patients with exposure to drugs that are known to be excreted unchanged by the kidney, have low degree of protein binding, exist in a weakly acidic form, and are primarily distributed in the extracellular fluid compartment such as salicylate, phenobarbital, chlorpropamide, 2,4-dichlorophenoxyacetic acid, diflunisal, fluoride, mecoprop, and methotrexate (15,16). Urinary acidification for weak bases, such as phencyclidine or amphetamines, should not be done due to risk of rhabdomyolysis and acute kidney injury (AKI) (17). Hemodialysis and other extracorporeal methods are commonly used for removal of toxins, particularly in patients with severe acid–base or electrolyte disorders, even when the nature of the toxic agent is not known.
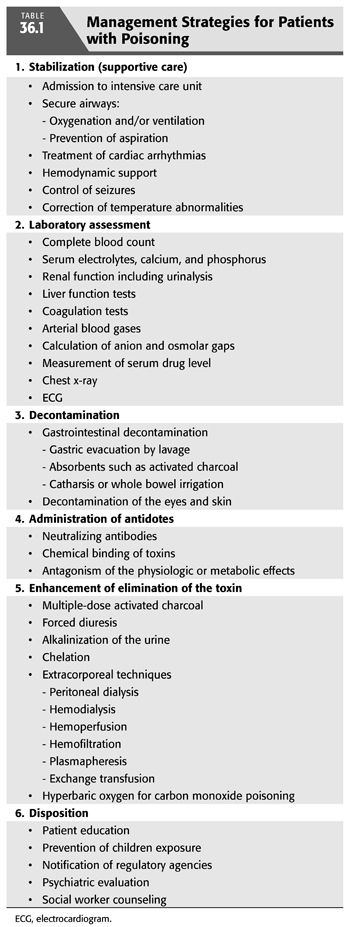
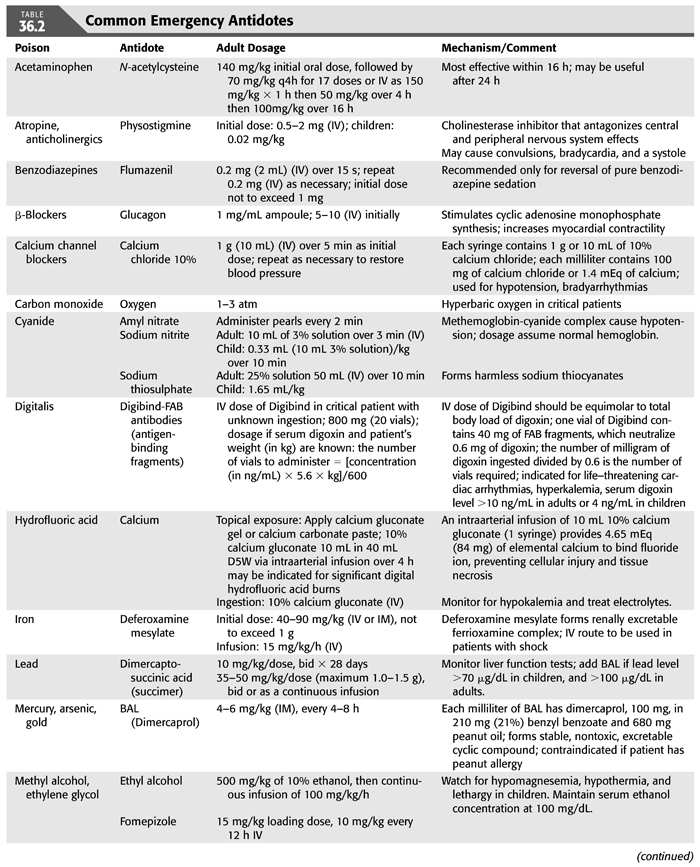
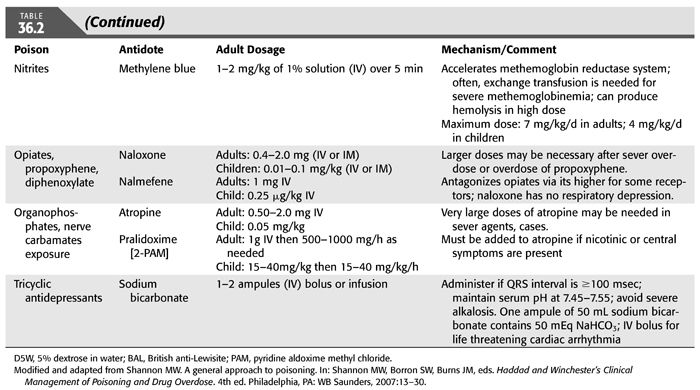
EXTRACORPOREAL TREATMENT OF POISONING
Available Extracorporeal Methods
Extracorporeal treatments (ECTRs) were required in 0.1% of all intoxications in the United States (1). Although the number may represent a small fraction of all toxic exposures, nephrologists are routinely consulted to help in the management of poisoned victims. The basis for their role in this clinical setting is fourfold. First, drugs and toxins may affect the renal function directly or through their effects on renal hemodynamics leading to AKI. Second, poisons may lead to serious clinical consequences such as acid–base and electrolyte abnormalities for which nephrologists are asked to manage. Third, the realization that poisons can be cleared from the body either by the kidney or by the use of extracorporeal techniques. Finally, their expertise in the dialysis procedure and presumed knowledge in the use of extracorporeal methods for enhancing toxins elimination.
The rationale for the application of extracorporeal techniques in the treatment of acute intoxication lies in their ability to increase the clearance of the offending toxin. Thus, active removal of toxins from blood would markedly reduce the duration of exposure to toxins and reduce the morbidity and mortality associated with poisoning and overdose. An array of methods has been proposed and studied, both singly and in combination. These include intermittent hemodialysis (IHD), sustained low-efficiency dialysis (SLED), intermittent hemofiltration (IHF) and intermittent hemodiafiltration (IHDF), continuous renal replacement therapy (CRRT), hemoperfusion (HP), therapeutic plasma exchange (TPE), exchange transfusion, peritoneal dialysis (PD), albumin dialysis, cerebrospinal fluid (CSF) exchange, extracorporeal membrane oxygenation (ECMO), and emergency cardiopulmonary bypass (TABLE 36.3) (18). The use of an optimal method of extracorporeal removal of toxins is often based on clinical judgment and anecdotal reports rather than on evidence-based controlled trials (18,19). A large body of work has emerged in this area and consists of theoretical considerations, in vitro and in vivo laboratory studies, and clinical observations ranging from large case series to anecdotal reports. However, in the selection of one method over the others, one must consider a number of factors that influence the removal of the toxin by these techniques (TABLES 36.4 and 36.5). The following is a brief description of the methods used for removal of toxins.
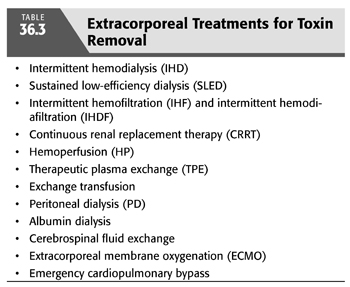
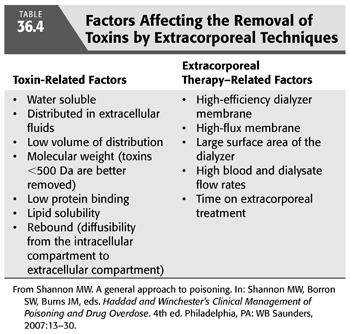
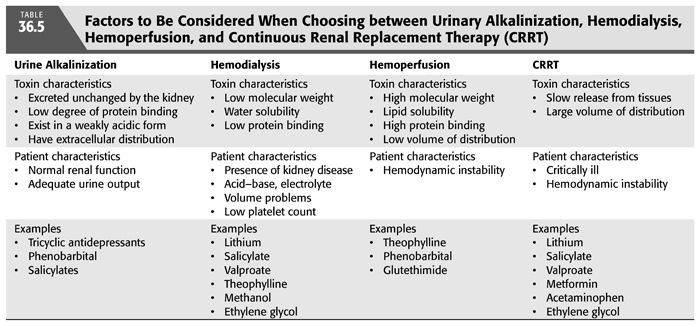
Hemodialysis
HD is the most commonly used technique for extracorporeal removal of toxins. The principles governing toxin removal by HD are essentially similar to those for treatment of uremia (19–23). HD is based mainly on the principle of diffusion. Because small solutes exhibit random molecular motion to a greater extent than larger solutes, the former solutes can diffuse through the semipermeable membrane more readily than the latter. Hence, the efficiency of dialysis in drug removal is inversely related, other things being equal, to the molecular size of the drug involved. The availability of the more permeable high-flux dialysis membranes will facilitate removal of toxins with larger molecular weights (MWs).
Drugs present in the circulation can be removed to a variable degree by HD depending on a number of factors (TABLE 36.4). These include the blood and dialysate flow rates; the physical and biologic characteristics of the offending agent such as a molecular size below the cutoff of the dialysis membrane, the volume of distribution (Vd) in the body, degree and affinity of protein binding, water or lipid solubility, state of ionization, and the physical characteristics of the dialyzer membrane [such as surface area, mass transfer area coefficient (KoA), porosity, electric charge, ability to adsorb the offending substance]; rate of ultrafiltration; and concentration gradient between blood and dialysate. For a solute that has a low MW, is not ionized, is water-soluble, and is not protein-bound, plasma dialyzer clearance can even exceed plasma flow rate and approximate blood-flow rate if that particular solute is readily equilibrated across the red cell membrane. Methanol is a drug that best exemplifies this model. Therefore, HD should be considered for poisoning resulting from toxins that have these favorable physical characteristics (TABLES 36.5 and 36.6). By contrast, HD may not be effective for removal of toxins that are lipid-soluble, protein-bound, or possess high MWs. However, the newer dialysis membranes have the capacity for removal of larger molecules and highly protein-bound poisons such as carbamazepine and phenytoin (24–27). TABLE 36.6 lists the various drugs that can be removed by HD.
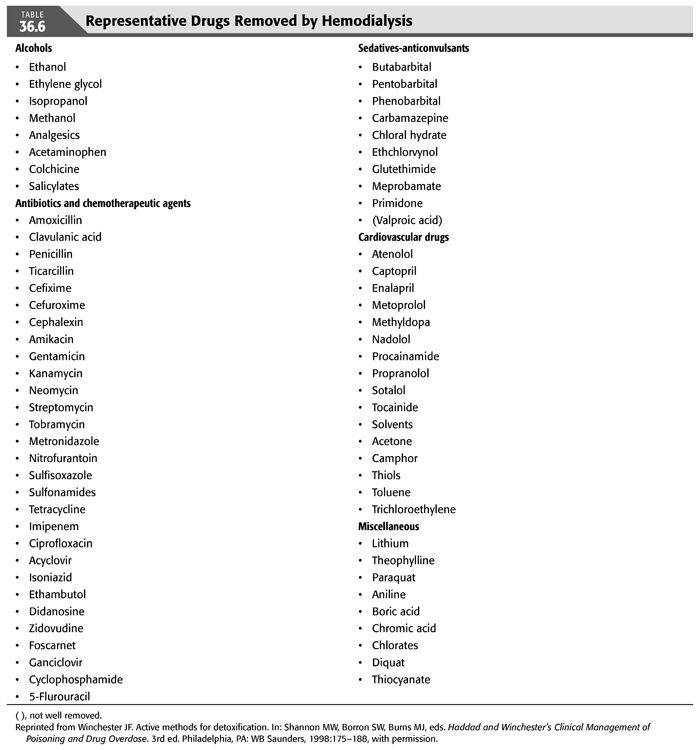
There are a number of advantages for intermittent HD use in treatment of poisoning. HD services are now widely available, even in poorer countries, are relatively easy to use and of low cost, and are associated with lower complication rate when compared to other methods of extracorporeal techniques. HD also corrects concomitant electrolyte and acid–base abnormalities and removes excess fluid with ultrafiltration. Clearly, HD should be employed whenever the intoxicated patient suffers concurrently from kidney disease or has developed acid–base, electrolyte, or volume abnormalities that can be readily rectified by HD. Finally, given that it can be quickly prepared and that outcome may be dependent on prompt removal of the poison from the body, intermittent HD remains the treatment of choice for most poisonings worldwide. However, HD treatment should be adjusted to each patient’s individual needs. Therefore, in hemodynamically unstable intoxicated patients, administration of physiologic saline, the use of bicarbonate-based dialysate, cool (e.g., 35°C) dialysate, and the use of vasoconstrictors such as midodrine are helpful. The composition of the dialysate should also be individualized. Therefore, a dialysate potassium concentration of 3 to 4 mEq/L is appropriate in the absence of hyperkalemia or hypokalemia. Similarly, the bicarbonate concentration in the dialysate should be adjusted according to the patient’s acid–base balance. Therefore, dialysate bicarbonate level should be lowered in patients’ with metabolic alkalosis but maintained at a high value in the presence of severe metabolic acidosis.
Hemoperfusion
This method is based on the adsorption of a poison to a sorbent-containing cartridge that is perfused by blood from a poisoned patient (28–30). The column is most commonly made from activated charcoal, but resins have also been used (28). As mentioned earlier, activated charcoal is an effective and commonly used oral agent for preventing gastrointestinal absorption of poisons. On the other hand, charcoal columns were used since the 1960s in HP to enhance elimination of poisons. Most commonly used HP cartridges are packed with 100 to 300 g of activated charcoal or an exchange resin and coated with a thin (0.05 mm), relatively porous semipermeable membrane. Adsorption occurs because of the hydrophobic properties of charcoal. HP can also be performed using commercially available resins which are made from heat-stable polymeric material in the form of cross-linked insoluble beads which can either be charged (e.g., Amberlite IRA-900) or uncharged, (e.g., Amberlite XAD-4) (24,30–32). The total amount of drug removed by HP is usually small and is not of great clinical consequence. However, although the extraction of highly protein-bound poisons is less than that for unbound poisons (33), studies using an adsorbent cartridge showed extraction ratios >80% when the proportion of protein-bound poison is <90% (30,34).
Removal of many toxins is influenced by the affinity of the charcoal for the toxin and by how avid the toxin binds to plasma proteins (28,30,32,35). In HP cartridges, the semipermeable membrane is not designed to withstand pressure but rather to impede the release of the particulate matter from the sorbent into the circulation and to prevent or reduce the adherence of platelets and other formed elements of blood onto the sorbents. Consequently, this membrane is extremely thin and does not significantly restrict solute diffusion. The process of activation of the charcoal sorbent has lead to enormous increase in the total available surface area of the sorbent. However, despite the large adsorbent area, HP cartridges may become saturated after 4 to 8 hours and lose their efficiency over time, so that they may need to be replaced. Moreover, HP can be associated with several other potential complications including a 20% to 30% decrease in the blood platelet count, leukopenia, hypocalcemia, hypoglycemia, charcoal embolism, and hypothermia. However, the frequency of these complications was reduced with the use of albumin cellulose nitrate to coat charcoal. TABLE 36.7 lists the various drugs that can be removed by HP.
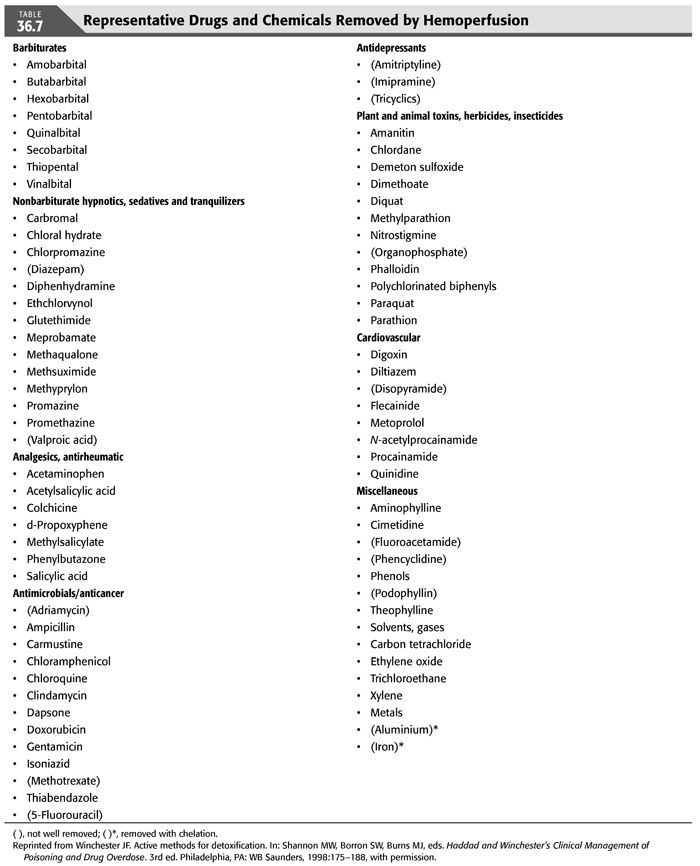
A frequently asked question is whether HD or HP is superior for treatment of poisoning. Early clinical experience with HP suggested that it was more effective than HD or PD for poison removal. However, recent studies have shown comparable results with the newer high-flux, high-efficiency dialyzers (36,37). TABLES 36.4 and 36.5 list the factors that should be considered when choosing between HD and HP as a treatment modality for poisoning. HP is preferred when the toxin is lipid-soluble or significantly protein-bound (36). Activated charcoal is capable of adsorbing and, thereby, extracting lipid-soluble substances much more efficiently than HD. On the other hand, HP does not contribute to the overall treatment of the patients with kidney disease and/or with acid–base, electrolyte, and fluid problems. Also, poisons with a large Vd are not likely to be efficiently removed by HP. Moreover, HP is associated with more frequent complications and higher cost, particularly since the cartridges need to be replaced regularly because of saturation. As a result, the number of HP reports has steadily declined during the last two decades (FIGURE 36.2). It should be noted that both HD and HP require the establishment of a vascular access and the administration of anticoagulants. However, anticoagulant-free HD can be performed by infusing 100 mL of physiologic saline into the dialyzer blood inlet every 30 minutes for short-duration HD.
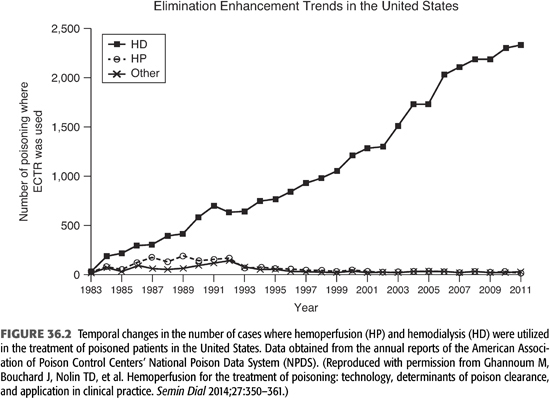
The earlier discussion may not be applicable to those systems that employ sorbent cartridges that regenerate dialysate. These systems employ small volumes of dialysate, and the cartridges used do not effectively bind alcohols. Consequently, such systems are not indicated in the treatment of ethylene glycol, methanol, or isopropyl alcohol poisoning. Although the cartridge may adsorb salicylate or lithium, there are, to our knowledge, no published studies documenting the effectiveness of sorbent systems in these intoxications. Finally, sorbent-based systems are said to be efficacious in the treatment of theophylline intoxication, but, again, there are no published reports available on this subject.
Sustained Low-Efficiency Dialysis
This technique allows the use of conventional HD machines to perform slow, low-efficiency HD using both reduced dialysate and blood-flow rate (32,35,38,39). Conventional hemodialyzers are used with a reduced dialyzer blood-flow rate of 200 to 300 mL/min and a slower dialysate flow rate of 300 mL/min. Each treatment can last up to 6 to 12 hours daily (35,40). The relatively long treatment duration compensates for the low efficiency of the procedure. Because of the slow blood and dialysate flow rates, the procedure is better tolerated by critically ill patients with hemodynamic instability (35,39). It is conceivable that this method may serve as an adjunctive approach to conventional extracorporeal measures in the treatment of poisoning. Although SLED uses a higher dialysate flow rate than CRRT, small solute clearance is reportedly similar (39,41). However, one study showed that the daily clearance of lithium was higher with SLED than with CRRT (42). Unfortunately, there are only a limited number of reports in the literature that describe the use of SLED in poisoning.
Continuous Renal Replacement Therapy
CRRT includes several techniques that utilize convective transport alone or in combination with diffusive transport. These techniques include (a) continuous venovenous hemodialysis (CVVHD), (b) continuous venovenous hemofiltration (CVVH), and (c) continuous venovenous hemodiafiltration (CVVHDF). The continuous venovenous techniques have largely replaced continuous arteriovenous therapies. Hemofiltration involves the removal of an ultrafiltrate from the blood through the semipermeable membrane of a filter. This is usually accompanied by the simultaneous, appropriate replacement with a physiologic solution that resembles plasma. The efficiency of the procedure is related to the total volume exchanged. Ultrafiltration is based on the principle of convection, and ultrafiltration is obtained by the application of a transmembrane pressure gradient. For all solutes that can pass through the pores of a semipermeable membrane, the relatively larger solutes and their smaller counterparts all pass through the membrane at the same rate (43,44). On the other hand, hemodiafiltration involves simultaneous HD and hemofiltration using the same dialyzer/filter. The procedure is more efficient than either HD or hemofiltration alone in the removal of poisons that are small enough to pass through a semipermeable membrane (36,37,44).
These CRRT techniques were initially promoted as superior to HD in the treatment of poisoning, particularly those associated with lithium, valproic acid, and theophylline (22). However, there is no clear evidence for this assertion (20). CRRT has been proposed as effective in the removal of tissue-bound substances, such as paraquat, in which the offending agent is released slowly from its reservoir and associated with the occurrence of a “rebound phenomenon” (45). However, in most circumstances, the amount of drug removed is hampered by the slow rate of CRRT therapies. Moreover, since most toxins have a relatively small MW, they can be removed easily by HD. In most cases, the need for poison removal is urgent, and therefore, SLED and CRRT should not be used as first-line treatment. In CRRT, the blood and effluent flow rates are lower than during intermittent HD, and thus, clearance will be lower over a similar period of time (46). This has been shown to be true in methanol poisoning where the clearance is limited to <50 mL/min with CRRT compared with >200 mL/min with HD (47). Also, carbamazepine clearance is 3 times lower with CRRT compared with HD (48). However, CRRT can be used following conventional HD for treatment of poisoning with known tendency for rebound as in lithium intoxication, or they can be started as primary therapeutic modalities when hemodynamic instability militates against the use of conventional HD, hemofiltration, or hemodiafiltration (44). However, there is no clear evidence for a beneficial effect of CRRT or SLED in such circumstances (46).
Peritoneal Dialysis
PD allows the diffusion of toxins from mesenteric capillaries across the peritoneal membrane into the dialysate within the peritoneal cavity. In PD, clearance of solutes including poisons depends on the volume and number of exchanges, the peritoneal surface area, as well as the MW of the solute to be removed. In general, the clearance of solutes is quiet low in the range of 10 to 15 mL/min, and therefore, PD is not considered an effective technique for treatment of poisoning (19,49). In order to enhance clearance by PD, continuous flow peritoneal dialysis (CFPD) was developed. This technique requires the insertion of a dual-lumen PD catheter or two single-lumen catheters at opposite positions. A large volume of dialysate, up to 300 mL/min, is continuously exchanged in and out of the peritoneal cavity (50). Again, there is very limited data using this technique for removal of poisons. Therefore, PD is seldom used as a primary modality in the treatment of poisoning except in circumstances where the more efficient ECTRs are not available or cannot be performed.
Plasmapheresis or Exchange Blood Transfusion
TPE (plasmapheresis) is a process in which separation of plasma from the blood cellular components is archived by centrifugation or filtration. The volume of plasma removed during a single procedure is between 30 and 60 mL/min (51,52), and each treatment lasts 2 to 4 hours (53). The patient’s plasma is replaced by other solutions such as fresh-frozen plasma, albumin, or crystalloid solution. On the other hand, exchange blood transfusion involves removal of the patient’s own blood and replacing it with fresh blood. These procedures are not usually effective in the treatment of poisoning because of the limitations on the amount of plasma or of blood that can be exchanged, especially in the case of toxins with large volumes of distribution (51,54,55). However, plasma exchange may be useful for removal of toxins that are highly protein-bound or have very low Vd. The American Society for Apheresis (ASFA) guidelines recommend an exchange volume of one to two total plasma volumes per day until clinical symptoms of poisoning have decreased and release of toxin from tissues is no longer significant (55). As of now, there are no clinical indications for the use of plasmapheresis in the treatment of poisoning and thus should be reserved for circumstances where alternative therapies are unavailable.
Albumin Dialysis
Albumin dialysis or extracorporeal liver assist devices (ELAD) includes the Molecular Adsorbent Recirculating System (MARS), the Prometheus system, and single pass albumin dialysis (SPAD) (56). With MARS, selective removal of albumin-bound substances can be achieved in a high-flux dialysis setting by the enrichment of dialysate with human albumin that functions as a molecular adsorbent. The albumin-enriched dialysate is then recirculated over sorbents. This novel dialytic approach has the theoretical advantage of enhancing elimination of protein-bound poisons in addition to excess water and water-soluble substances (57–59). With that in mind, this technique has been used successfully in anecdotal reports for the treatment of poisoning resulting from acetaminophen and cytotoxic mushrooms in the presence or absence of concomitant renal insufficiency (59,60). However, the role of albumin dialysis in poisonings remains unclear (61).
Clearance versus Drug Removal and Clinical Efficacy
A number of mathematical methods for describing the kinetics of drug removal during various extracorporeal techniques have been proposed in order to assess the efficacy of these techniques in achieving drug removal. During HD, the dialyzer clearance of a substance is defined as the volume of blood from which the substance is completely removed per unit time. If the drug concentrations in the blood entering and exiting the dialyzer and the rate of dialyzer blood flow rate are known, the dialyzer clearance of the drug can be calculated as follows (EQUATION 36.1):

where
C = dialyzer clearance
QB = blood-flow rate through the dialyzer
A = the blood concentration of the drug at the blood inlet of the dialyzer
V = the blood concentration of the drug at the blood outlet of the dialyzer.
Alternatively, dialyzer clearance can be calculated by timed collection of spent dialysate from the dialysate outlet of a dialyzer and simultaneously obtaining arterial blood (or plasma) at the blood inlet at 30 seconds (62). Then the dialyzer clearance of a substance (in mL of blood or plasma/minute) will equal the amount of substance in mg collected in dialysate/arterial blood (or plasma) concentration of the substance in milligrams per milliliter.
Using either of these two methods, it can be shown that, given the proper drug characteristics and the use of appropriate dialyzers/ultrafilters or HP apparatus, drugs can be extracted from circulating blood with startling efficiency. In this example, the clearance of many toxins may approximate the blood-flow rate, and the extraction ratio may approach 100%; that is, drug concentration at the dialyzer/ultrafilter outlet may be close to zero.
Although these mathematical calculations imply that drugs are eliminated efficiently, thereby supporting the use of extracorporeal techniques for treatment of various intoxications, such an interpretation is not always accurate. An efficient extracorporeal procedure does not necessarily indicate that a clinically significant amount of intoxicant is being removed from the patient. This is because the total amount of drug removed depends, among several factors, on the apparent Vd of the toxin. A drug with a large Vd indicates that the substance is sequestered somewhere other than the vascular compartment such as the interstitial or intracellular fluid or bound to cellular proteins, cellular lipids, or cellular membranes. Under these circumstances, the extravascular distribution volume of the toxin results in slow release of the toxin into the vascular compartment. Such a scenario means that only a small fraction of the total amount of the toxin present in the body is accessible for removal by extracorporeal techniques during a given period of time. This is often the case with digoxin or tricyclic antidepressants poisoning. With these drugs, the largest amount of the ingested drug is not circulating but rather is bound to intracellular components; therefore, the total quantity of drug available for extraction from the blood is minimal. Even if one is successful in completely extracting the drug from the circulation in one treatment session by extracorporeal device, only a small fraction of the total body toxin load is removed. Therefore, efforts to remove the drug by extracorporeal techniques may be futile and may even be harmful putting in mind potential complications of the procedure and its cost. Moreover, the use of these techniques for removal of drugs with these characteristics may distract from implementing the full range of supportive measures the value of which vastly outweigh the possible minimal gain derived from the procedure (63–68).
CRITERIA FOR EXTRACORPOREAL TREATMENT OF POISONING
From the preceding discussion, it is clear that not every patient with poisoning is a candidate for ECTR. Therefore, in order to consider extracorporeal means of detoxification, one should determine that this modality will significantly augment toxin removal above that achieved by spontaneous elimination either by metabolism or by the native kidneys. Unfortunately, the indications for ECTR of poisoning are not well delineated since no randomized controlled trials have been conducted in patients with poisoning. However, the EXtracorporeal TReatments In Poisoning (EXTRIP) workgroup is developing recommendations on the use of ECTR for at least 16 poisons (69,70). In clinical practice, the following clinical and toxicologic characteristics must be present before one can justify the use of extracorporeal techniques for toxin removal (TABLE 36.8 and FIGURE 36.3) (69,71):
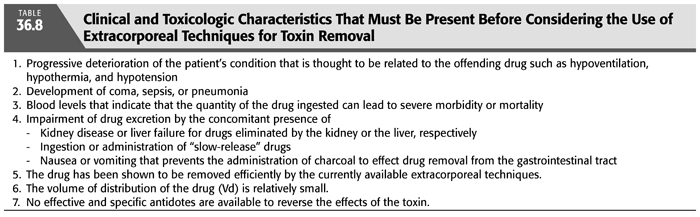
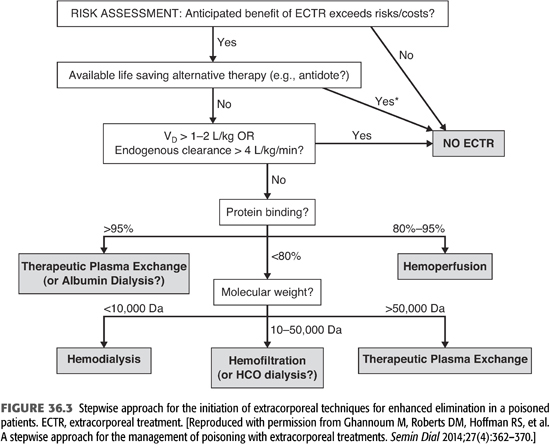
1. Progressive deterioration of the patient’s condition thought to be related to the offending drug
• Depression of the midbrain functions leading to hypoventilation, hypothermia, and hypotension
• Development of coma, sepsis, or pneumonia
2. Blood levels indicating that the quantity (dose) of the drug ingested can lead to severe morbidity or mortality
3. Impairment of drug excretion by the concomitant presence of
• Kidney disease or liver failure for drugs eliminated by the kidney or the liver, respectively
• Ingestion or administration of “slow-release” drugs
• Nausea or vomiting that prevents the administration of charcoal to effect drug removal from the gastrointestinal tract, especially in theophylline intoxication
4. The drug has been shown to be removed efficiently by the currently available extracorporeal techniques. This implies that the drug is not significantly bound to plasma proteins or lipids and that its molecular size, shape, and charge permit removal through dialyzer/ultrafilter membranes or by adsorption to sorbents.
5. The Vd of the drug is relatively small, implying that a large fraction of the drug is present in body water, from which it can be readily removed.
6. No effective and specific antidotes are available to reverse the effects of the toxin [e.g., in digoxin poisoning, the digoxin-specific antibody Fab fragment (Digibind) can be used].
With these criteria in mind, it becomes apparent that only few toxins can be effectively removed by extracorporeal techniques. Unfortunately, many of the toxins that account for a high percentage of total morbidity and mortality are not amenable to removal by extracorporeal techniques despite the high clearance rate achieved by extracorporeal techniques. Examples of such drugs and/or toxins include the following:
Tricyclic antidepressants. The tricyclic antidepressant (TCA) class of drugs is typified by a high Vd because of high lipid solubility. The fraction of drug available for extraction from the circulation is minuscule. The EXTRIP workgroup concluded that TCAs are not dialyzable and stated that ECTR is not recommended in severe TCA poisoning because poisoned patients with TCAs are not likely to have a clinical benefit from extracorporeal removal (72).
Barbiturates. The short-acting barbiturates are highly lipid-bound and poorly dialyzable. On the other hand, longer acting barbiturates, especially phenobarbital, are more water-soluble and therefore are efficiently removed by renal excretion and by HD. Alkalinization of the urine has been used to increase their rate of excretion. HP, although effective in removing circulating long-acting barbiturates, has not been proven to provide clinical benefits. HD is indicated in the patient intoxicated with long-acting barbiturates and who have poor renal function. The EXTRIP workgroup made four key recommendations. (a) The use of ECTR should be restricted to cases of severe long-acting barbiturate poisoning. (b) The indications for ECTR in this setting are the presence of prolonged coma, respiratory depression necessitating mechanical ventilation, shock, persistent toxicity, or increasing or persistently elevated serum barbiturate concentrations despite treatment with MDAC. (c) Intermittent HD is the preferred mode of ECTR, and MDAC treatment should be continued during ECTR. (d) Cessation of ECTR is indicated when clinical improvement is apparent (73).
Acetaminophen. Acetaminophen overdose is one of the most common agents leading to patients’ death. Unfortunately, it is not efficiently extracted by extracorporeal procedures. Early and prolonged use of N-acetylcysteine (NAC) remains the therapy of choice (74). The EXTRIP workgroup agreed that acetaminophen is dialyzable but recommended ECTR only in patients with excessively large overdoses who display features of mitochondrial dysfunction manifested by early development of altered mental status and severe metabolic acidosis prior to the onset of hepatic failure. They suggested that IHD is the preferred ECTR modality in acetaminophen poisoning (75).
Narcotics and “street” drugs. A common cause of overdose and death, narcotics and other street drugs are not amenable to extraction by extracorporeal techniques. Opium antagonists and supportive therapy are the mainstays of treatment.
Nonbarbiturate hypnotics, sedatives, and tranquilizers. Many of these drugs cause little morbidity and mortality, even in apparently severely intoxicated patients. Furthermore, they all display a high apparent Vd and lipid solubility and therefore are not removed well by extracorporeal means.
Other miscellaneous toxins. This group includes paraquat, amanita mushroom toxin, and methotrexate. Contrary to some opinions and anecdotal reports, there is little evidence that extracorporeal techniques are clinically effective for the treatment of poisonings resulting from these toxins. However, because paraquat and N-acetyl procainamide (NAPA) are tightly tissue bound and released slowly, it has been proposed that CRRT may be efficacious (16,67). Although clinical case reports have suggested that patients suffering from life-threatening NAPA intoxication could be helped with the various extracorporeal measures, their effectiveness remains debatable (67). TABLE 36.9 lists some agents for which various extracorporeal methods should be used for treatment of acute intoxications.
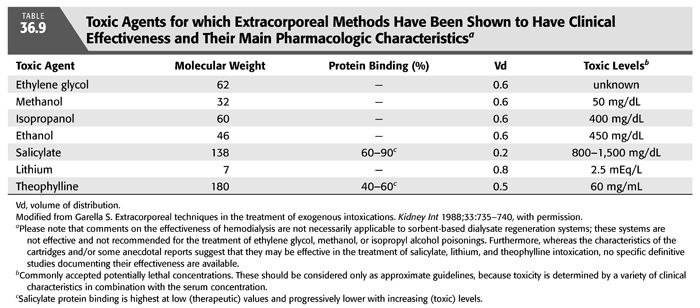
POISONING FROM TOXIC ALCOHOLS
The term alcohol usually implies ethyl alcohol, the predominant alcohol in alcoholic beverages. However, alcohol also includes any hydroxyl derivative of a hydrocarbon. Methanol is the simplest primary alcohol that can be produced by distillation of wood. It is used in paints, windshield washer fluid, solvents and thinners, plastics, synthetic textiles, as antifreeze, and as a fuel. Ethylene glycol is an organic compound primarily used as a raw material in polyester fibers and fabric industry, and polyethylene terephthalate resins in bottling, and is also used in other applications such as antifreeze. Both methanol and ethylene glycol are low MW, clear, and colorless alcohols. Methanol has an alcoholic taste, but ethylene glycol is sweet. Methanol and ethylene glycol are toxic alcohols that can result in serious morbidity and mortality. Accidental or intentional ingestion of these alcohols may result in blindness, renal dysfunction, chronic brain injury, or death (76). Factors associated with increased mortality include large osmolal gap, anion gap, and low pH <7.22. However, pH <7.22 has the highest predictive value for mortality (77). Methanol and ethylene glycol constitute medical emergencies for which early diagnosis and treatment may be lifesaving. Most of the toxicity related to methanol and ethylene glycol are related to their metabolites such as formate for methanol and oxalate and glycolate for ethylene glycol. The introduction of fomepizole as a competitive inhibitor of alcohol dehydrogenase has revolutionized treatment of toxic alcohol poisonings.
Ethylene glycol and methanol poisoning are common, but ethylene glycol poisoning is very rare in mid- and low-income countries compared to high-income countries. In 2009, there were 8,139 poisoning due to toxic alcohols in the United States. In general, poisoning with these alcohols in industrialized countries tends to be intentional in adults and accidental in children, while in developing world, it is often accidental. The minimum lethal dose for ethylene glycol is commonly cited as 100 mL or 1 to 1.5 mL/kg but also with considerable variability (76).
METHANOL POISONING
Methanol, also known as wood alcohol, is a clear, colorless, low MW alcohol commonly found in a variety of products including industrial solvents, windshield wiper fluid, windshield deicer, antifreeze, stains, dyes, varnish, shellac, canned fuel (Sterno), as well as an alternate fuel (76). Methanol poisonings most often occur from the ingestion of “moonshine” liquor (78) as well as from ingestion of windshield washer fluid but can also arise from its dermal application or inhalation. Because methanol (MW = 32 Da) possesses inebriating qualities similar to those of ethanol, is inexpensive, and widely available, it has been responsible for large outbreaks of poisoning (79–81). In the United States, during the years 2000 to 2013, there were 30,395 methanol exposures, 85,891 ethylene glycol exposures, and 3,235 combined exposures (82). Most of the exposures that have been recorded were acute and were primarily due to unintentional exposures to windshield wiper fluid and other automotive products with 25% of these occurring among children younger than 12 years old (2).
Methanol is rapidly and completely absorbed from the gastrointestinal tract with peak plasma levels attained in 30 minutes to 1 hour. It can penetrate skin, and thus, there have been reported cases of poisoning by extensive dermal contact or inhalation (83,84). Methanol does not bind to protein and has an apparent Vd of approximately 0.6 to 0.7 L/kg. Only about 5% of ingested methanol is excreted unchanged in the urine; the rest is oxidized in the liver. Some methanol is also eliminated by the lungs. If untreated, the elimination half-life of methanol is 1 to 3 hours at low concentrations and approximately 24 hours at high concentration.
Methanol is initially metabolized by alcohol dehydrogenase to formaldehyde (FIGURES 36.4 and 36.5). This process occurs slowly and constitutes the rate-limiting step of this reaction. Formaldehyde is cytotoxic as it may be involved in causing blindness (85,86). However, it does not accumulate because it has a very short half-life of only 1 to 2 minutes and undergoes rapid conversion to formic acid (formate). Formic acid molecule dissociates into a hydrogen ion and a formate anion, which in turn is broken down via a folic acid–dependent pathway to carbon dioxide and water. The body has limited capacity to transform formic acid; thus, accumulation of this toxin may occur increasing the susceptibility to its toxicity. The released hydrogen ion from formic acid lowers the pH of body fluids causing acidosis. In addition to formic acid, lactic acid also contributes to the severe metabolic acidosis (76,85,87). Because the first step of methanol metabolism depends on the action of alcohol dehydrogenase, which has a 10-fold greater affinity for ethanol or fomepizole than for methanol, these compounds are currently used as antidotes in the therapy of methanol poisoning (see section “Treatment”). The same mechanism explains the prolongation of the half-life of methanol to more than 30 hours (compared with the normal of 12 to 20 hours) in patients who had ingested both methanol and ethanol at the same time (88). Oxidation of methanol, like that of ethanol, proceeds independently of the serum concentration but at a rate of only 15% of that of ethanol. Thus, complete oxidation and excretion of methanol usually requires several days which also explains the delay in the onset of toxic manifestations (84).
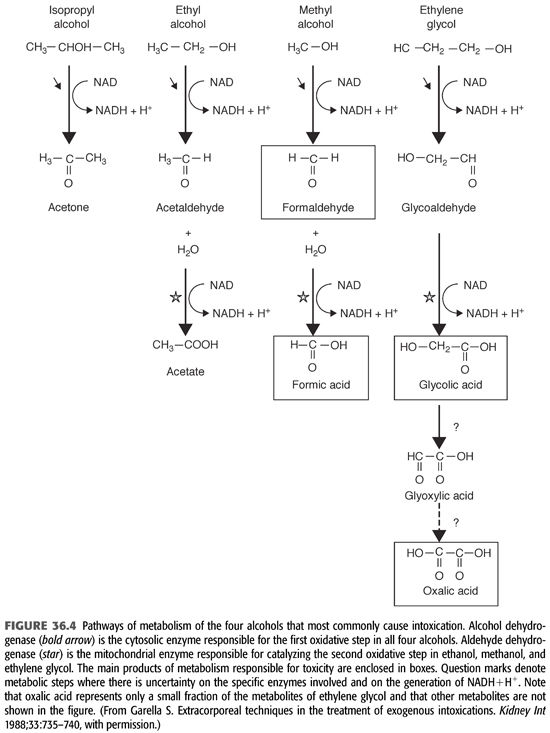
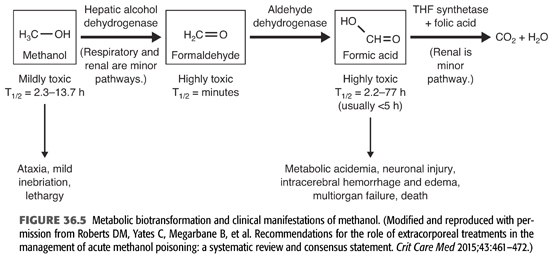
Clinical Picture and Laboratory Data
The clinical manifestations of methanol poisoning depend on the amount ingested, the elapsed time since ingestion, and whether there was coingestion of ethanol or other toxic substances (76). The clinical course of methanol toxicity is characterized by three distinctive stages and involves the central nervous system (CNS), the eyes, and the gastrointestinal system (TABLE 36.10) (88–90). An initial and fairly brief stage is characterized by CNS depression in which the patient appears intoxicated; manifestations largely due to the effects of the parent compound. This is followed by a latent stage of 6 to 30 hours during which the patient may be asymptomatic except for blurred vision. This asymptomatic stage is followed by a third stage in which formic acid accumulates and more serious toxicity develops. Formic acid is directly toxic to the retina, and therefore, visual manifestations of methanol poisoning tend to develop rapidly (91). These manifestations include central scotoma, blurred vision, decreased visual acuity, photophobia, and progression to complete blindness. Examination reveals decreased papillary response to light and visual field defects. Later on, the patient develops fixed dilated pupils, hyperemia of the optic disc, papilledema, and optic atrophy (91,92). The CNS manifestations include headache, vertigo, delirium, restlessness, and confusion. These may progress to seizures, coma, and death in severe methanol intoxication. The minimal lethal doses of methanol are not well established, but it is commonly estimated at 15 mL (76,93). In 10% to 20% of cases, hemorrhagic and nonhemorrhagic necrosis of the putamen can occur (94,95). Permanent blindness and parkinsonism are common sequelae among survivors (96). Indeed, acute Parkinson’s syndrome was reported in several patients with severe methanol poisoning and was thought to result from acute hemorrhagic necrosis of the basal ganglia (96–98). Lastly, methanol poisoning commonly result in significant gastrointestinal manifestations such as nausea, vomiting, diarrhea, and abdominal pain (probably as a result of gastritis and/or pancreatitis).
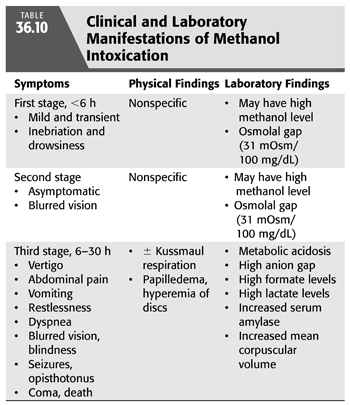
Laboratory findings include increased serum amylase value, high anion gap metabolic acidosis, and elevated serum formate and lactate levels. The increased lactate values are, as in the case of ethylene glycol intoxication, most likely the result of both an increased nicotinamide adenine dinucleotide (NADH/NAD) ratio and a reduction in tissue perfusion. A high osmolal gap may be also apparent. Methanol contributes 31 mOsm/kg for each 100 mg/dL; therefore, a sizable osmolal gap is more likely to be present in the initial stages of the intoxication before the alcohol is metabolized. Other abnormal laboratory tests include an increased erythrocyte mean corpuscular volume attributed to a toxic effect of formaldehyde on cellular ion transport.
The quantity of ingested methanol that is required to cause serious morbidity and mortality is highly variable. While some patients may develop severe complications following the ingestion of a few milliliters of methanol, others survive the ingestion of several hundred milliliters. In addition, the serum concentration of methanol does not correlate well with the severity of the clinical picture or with prognosis (88,89). However, there are a number of findings that indicate poor prognosis (76,99). These include dyspnea and Kussmaul’s respiration, which result from severe metabolic acidosis. Moreover, blindness, seizures, coma, elevated formic acid level, bradycardia, and hypotension also indicate poor prognosis (97,98). Respiratory failure is the most common cause of death in methanol poisoning. Untreated methanol poisoning is associated with death in 28% and visual deficits or blindness in 30% in survivors (78).
Laboratory Diagnosis of Methanol Intoxication
Measurements of plasma or serum methanol concentration using colorimetric or enzymatic assays can provide confirmation of toxic exposure to methanol. Unfortunately, these quantitative assays for methanol are not available in most hospitals. Even when these are available, results must be interpreted with caution. Plasma osmolality increases in proportion to the concentration of methanol or ethylene glycol, and its measurement provides a clue to ingestion of these agents if the result is abnormally high. The serum osmole gap provides a simple screening method of checking for increased osmolality that is not explained by other factors. An increased serum osmole gap in conjunction with a normal serum anion gap and absence of metabolic acidosis is frequently seen early after ingestion of methanol or ethylene glycol, before significant metabolism of methanol to formate or ethylene glycol to glycolate. However, as methanol and ethylene glycol are metabolized, their plasma concentrations decrease, and thus, their contribution to plasma osmolality and the osmolar gap also decrease (100,101). Consequently, if the patient presents after most of these alcohols have been metabolized, the serum osmolar gap may well have returned to normal despite the presence of lethal concentrations of their toxic metabolites (FIGURE 36.6). Nonetheless, metabolic acidosis develops, and the anion gap progressively increases, signifying accumulation of the toxic metabolites. The metabolic acidosis of methanol and ethylene glycol poisoning is often very severe with dangerously low blood pH as well as extremely high serum anion gap. Blood lactate levels are occasionally increased in both methanol and ethylene glycol poisoning. This may be due to use of NAD+ and excess accumulation of NADH from the metabolism of methanol or ethylene glycol which raises the NADH to NAD+ ratio, leading to increased transformation of pyruvate to lactate in order to replenish NAD+ (102). Other factors may also contribute to lactic acidosis including shock, hypoxia, and seizures. In methanol intoxication, plasma formate levels correlate with blood pH and serum anion gap (89,100,102). Other laboratory abnormalities that can be seen include hypocalcemia, increased serum amylase, and thrombocytopenia.
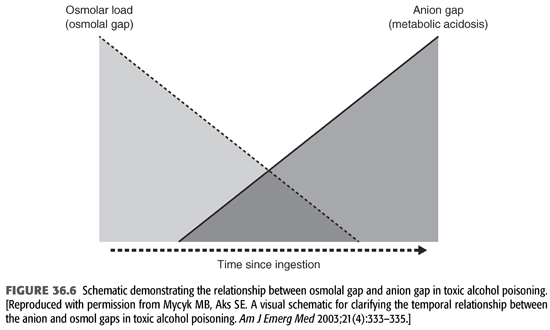
Treatment
Supportive care should be directed at stabilizing the airways and correcting ventilatory and circulatory disorders. Concomitantly, other interventions should be instituted with the aim to, first, correct metabolic acidosis; second, prevent other serious complications by limiting the accumulation of the toxic metabolites of methanol; and third, removal of methanol and its byproducts such as formic acid. Metabolic acidosis should be treated with intravenous (IV) infusion of sodium bicarbonate. Seizures are managed primarily with parenteral benzodiazepines. Gastrointestinal drainage can be attempted but is usually ineffective because of the rapid absorption of methanol (88,90,103,104). Folic acid administered as 50 mg IV every 4 hours for five doses then once daily may be helpful as it has been shown to increase the metabolism of formic acid to carbon dioxide and water (87,105). As with chronic ethanol use, routine administration of thiamine to patients with known or suspected toxic alcohol ingestion may be justified (76).
In addition to the aforementioned supportive therapy, aggressive treatment must be started promptly when there is a reasonable suspicion of significant methanol intoxication. The prompt administration of inhibitors of alcohol dehydrogenase enzyme such as ethanol or fomepizole is very effective in preventing the development of metabolic acidosis by markedly decreasing the oxidative metabolism of methanol (87,90,106). Fomepizole was approved in the United States for the treatment of ethylene glycol poisoning in 1997 and in 2000 for methanol toxicity. Indications for starting IV ethanol or fomepizole administration include (a) documented recent history of ingestion of more than 0.4 mL/kg body weight of methanol; (b) a serum methanol level greater than 6 mmol/L (20 mg/dL); (c) osmolar gap greater than 10 mOsm/kg H2O; and (d) history or strong clinical suspicion of methanol poisoning and at least two of the following findings: arterial pH <7.3, a serum bicarbonate level <20 mEq/L, or an osmolar gap >10 mOsm/kg H2O (88,90,97) (TABLE 36.11). Ethanol administration, both oral and IV, has been used for years as an antidote for both methanol and ethylene glycol poisoning. The basis for ethanol therapy is that both alcohol dehydrogenase and aldehyde dehydrogenase, along with nicotinamide cofactors are involved in the metabolism of both ethanol and methanol. In this reaction, ethanol is converted to acetaldehyde then to acetic acid, while methanol is converted to formaldehyde then to formic acid. Acetaldehyde is less toxic than formaldehyde, and acetic acid is essentially nontoxic, while formic acid has substantial toxicity (76). The two alcohols compete for the active sites on these two enzymes. However, the affinity of alcohol dehydrogenase for ethanol is far greater than for methanol or ethylene glycol. Thus, in presence of ethanol plus either methanol or ethylene glycol, there preferentially metabolizes ethanol and impedes that of methanol of ethylene glycol allowing time for these alcohols to be slowly excreted by the lungs and kidneys or to be slowly metabolized (107). For ethanol, a serum or blood alcohol concentration in the range of 100 to 150 mg/dL (22 to 33 mmol/L) is recommended in order to fully inhibit alcohol dehydrogenase (76). However, fomepizole, which is also a competitive inhibitor of alcohol dehydrogenase, has several advantages over ethanol and is now the preferred antidote for methanol poisoning (76,106). The doses of ethanol or of fomepizole used are similar to those recommended for ethylene glycol poisoning (90). TABLE 36.12 outlines the various dosing regimens for fomepizole before, during, and after the initiation of HD in patients with both methanol and ethylene glycol poisoning (90,104,108).
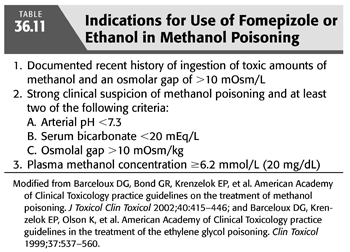
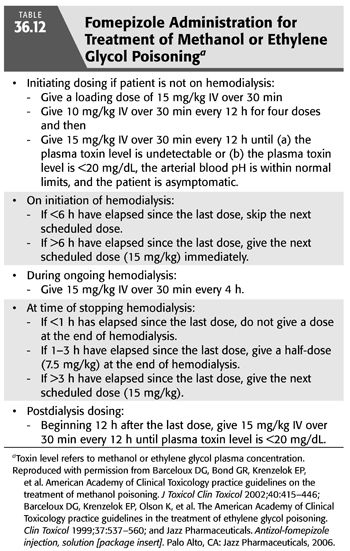
Unfortunately, ethanol or fomepizole does not completely prevent the formation of the methanol or ethylene glycol toxic metabolites. However, since these alcohols and their toxic metabolites are small molecules, they are readily dialyzable. Intermittent HD is the most effective means of removal of both methanol and formate with clearance rates of 125 to 215 and 203 mL/min, respectively (90,97,98). However, continuous modalities can be used as alternative therapeutic measures when conventional HD cannot be performed (87). HP using activated charcoal is ineffective.
HD can be critically important in patients with a significant delay between the time of ingestion and the time of administration of either ethanol or fomepizole, since significant amounts of formate or glycolate have already accumulated. The EXTRIP workgroup developed evidence-based consensus recommendations for ECTR indications in the management of methanol poisoning (TABLE 36.13) (87). HD should be performed with a bicarbonate bath because the alkali requirement may be massive due to the presence of severe metabolic acidosis associated with the intoxication (67). Methanol redistributes after discontinuation of the HD procedure and thus may result in rebound increase in methanol levels which usually occurs within the 12- to 36-hour period after stopping HD. The same is true for formate necessitating prolonged HD as a therapeutic measure (109). Also, after HD, close monitoring of acid–base balance, osmolar gap, and electrolytes should be done every 2 to 4 hours for 12 to 36 hours during which fomepizole or ethanol therapy should be continued because rebound is unpredictable. Hypophosphatemia may occur in those patients who require prolonged and intensive dialysis sessions, especially in individuals who are normophosphatemic to begin with. As previously discussed, the addition of sodium phosphate salts to the dialysate (1.3 mmol/L of phosphorus in the dialysate) has been used successfully to prevent hypophosphatemia in such patients. HD must be continued until the serum methanol level is either undetectable or less than 25 mg/dL, there is no significant metabolic acidosis, and the osmolal gap becomes normal (76,88,90,97). The addition of ethanol to the dialysate has been advocated in patients with methanol poisoning in an attempt to maintain stable levels of ethanol during dialysis (110–112). Because of the possibility of intracerebral hemorrhage in methanol poisoning, dialysis should be performed with little or no anticoagulation (113).
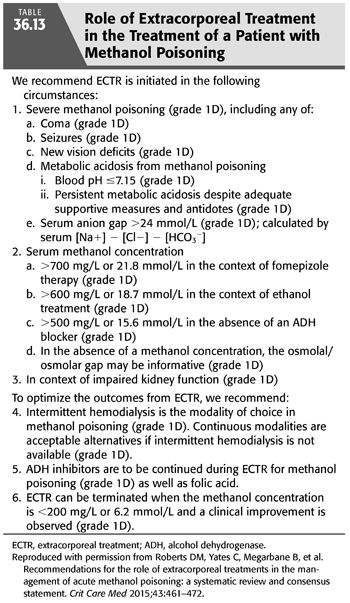
ETHYLENE GLYCOL POISONING
Ethylene glycol (EG) poisoning may occur either as isolated single cases or as epidemics (114). The majority of clusters of EG poisoning resulted from preparing illicit beverages using EG to fortify or in place of ethanol. Cases of EG intoxication have also occurred because of contaminated water systems (115). The TESS data for the year 2005 indicate a total of 5,469 reported toxic exposures to EG resulting in 16 deaths and 176 life-threatening poisonings (116). In 2007, poison centers in the United States received reports of 5,731 possible EG exposures (117). However, because of underreporting, these data likely underestimate the total number of cases. Most (84%) of the poisonings were unintentional. More recently, a study reported that during the years 2000 to 2013, there were more than 85,000 EG exposures (82). Most of the exposures were acute and again were primarily due to unintentional exposures to windshield wiper fluid and other automotive products with 25% of these occurring among children younger than 12 years old (2). Adults are typically exposed when they ingest EG as a cheap substitute for ethanol or in suicidal attempts. EG, also referred to as sweet killer, is an organic solvent commonly found in antifreeze preparations, deicers, coolants, brake and hydraulic fluids, and household cleaners. It is a sweet-tasting, viscous, colorless liquid with a molecular formula of C2H6O2 and an MW of 62 Da (88,118). EG ingestion, like methanol, can also result in life-threatening poisoning. While ingestion is by far the most common route of poisoning, a recent case of transcutaneous diethylene glycol poisoning with severe AKI was reported (119).
Pharmacokinetics
EG is an alcohol that is rapidly absorbed from the gastrointestinal tract with peak serum concentrations 1 to 4 hours after ingestion. Being highly water-soluble and nonprotein-bound, it diffuses promptly through the total body water; its Vd is approximately 0.5 to 0.8 L/kg (120). The metabolism of EG is more complex than methanol. The liver metabolizes 80% of the absorbed EG in a stepwise NAD-dependent fashion (108,121,122). Alcohol dehydrogenase, the first enzyme in the pathway, oxidizes EG to glycoaldehyde and is competitively inhibited by both ethanol and fomepizole (FIGURES 36.4 and 36.7). Glycoaldehyde is then rapidly converted to glycolic acid. The next step involves the conversion of glycolic acid to glyoxylic acid. The rate-limiting step in the metabolism of EG is the conversion of glycolic acid to glyoxylic acid, which results in the accumulation of glycolic acid in massive poisonings. Glycolic acid, with contribution from lactic acid, causes severe metabolic acidosis (121,122). Finally, glyoxylic acid is converted further to oxalic acid which combines with ionized calcium in plasma to form calcium oxalate and precipitate as calcium oxalate crystals in various tissues including the kidney, brain, meninges, blood vessel walls, liver, spleen, pericardium, and cardiac conduction system. These crystals may also appear in the urine (123). Glycolic, glyoxylic, and oxalic acid all dissociate under physiologic conditions, releasing hydrogen ions from their carboxylic acid moieties (76). Minor pathways involving thiamine and pyridoxine as cofactors can also bring about the conversion of glyoxylic acid to glycine and benzoic acid. However, these pathways are clinically insignificant, and there are no data to support the use of these cofactors in the treatment of EG intoxication (108). The average elimination half-life of EG is approximately 3 hours. Ethanol, at serum concentrations of 22 to 44 mmol/L (100 to 200 mg/dL), can effectively inhibit alcohol dehydrogenase and prolong the half-life of EG to almost 18 hours (124). Fomepizole, at serum concentrations greater than 9.8 mmol/L (0.08 mg/dL), can also prolong the half-life of EG to 20 hours (125,126).
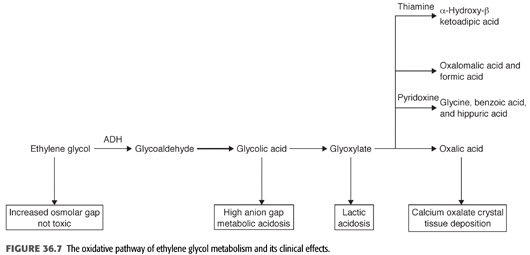
Clinical and Pathologic Effects of Ethylene Glycol
Ingestion of EG leads to a metabolic acidosis and severe damage to the CNS, heart, lungs, and kidneys. EG itself is relatively harmless and eliminated by the kidney. The mechanism of toxicity of EG poisoning is primarily due to its conversion to four toxic metabolites (FIGURE 36.7). These metabolites are cell toxins that cause CNS depression and cardiopulmonary and kidney disease. The clinical course of EG develops in three different stages, but overlap is frequently seen (TABLE 36.14) (67,127). The stage and its severity depend on the amount of EG ingested, the possible coingestion of ethanol or other chemicals, and the timing of medical interventions (88,97,126). The presence of severe acidosis, hyperkalemia, seizures, and coma on admission indicates severe intoxication (128).
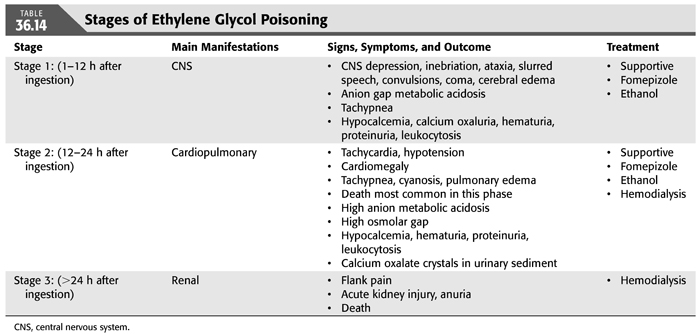
Stage 1 is predominantly neurologic and develops as a result of CNS involvement. The symptoms occur shortly after ingestion and may last for 12 hours. Initially, the patient appears intoxicated, sometimes with sustained nausea and vomiting, but without the odor of ethanol (if no ethanol has been ingested concomitantly). These symptoms are probably related to the direct effects of EG on the CNS. This is followed by hyporeflexia, coma, and focal or generalized seizures, often accompanied by ophthalmoplegia, nystagmus, and papilledema. These manifestations are most likely to reach their peak between 6 and 12 hours after ingestion and have been attributed to the toxic effects of the glycoaldehyde metabolites. Cerebral edema and diffuse petechiae are found in patients who die during this stage; focal deposition of calcium oxalate crystals is also seen. A lesser recognized devastating complication of EG is acute Parkinson’s syndrome. In one such case, it was thought to result from acute hemorrhagic necrosis of the basal ganglia (98). Moreover, EG intoxication during pregnancy may mimic eclampsia (129).
During stage 2, cardiopulmonary manifestations develop usually 12 to 24 hours after ingestion. These consist of tachycardia and mild hypertension but usually progress to pulmonary edema and cardiovascular collapse in massive poisoning. These manifestations result from deposition of calcium oxalate crystals in the vessels, myocardium, and lung parenchyma. In severe cases, multiorgan failure develops, and the patient succumbs to the poisoning. It is important to note that most lethal cases occur during this stage.
Stage 3 is predominantly renal and develops in patients who survive for more than 24 hours after ingestion of EG. These patients develop AKI, with oliguria, flank pain, and the presence of calcium oxalate crystals in the urine. Calcium oxalate precipitates in the renal tubules is thought to be the cause of EG-induced AKI, but other studies suggested involvement of other metabolites in causing acute tubular necrosis (130). The mechanisms responsible for the cardiopulmonary and renal manifestations are not entirely clear but are likely due to the toxic effects of the metabolites of EG. Prompt intervention designed at removal of EG and its more toxic metabolites may lead to complete recovery of CNS, cardiopulmonary, and renal manifestations.
Diagnosis
The diagnosis of EG poisoning should be considered in every intoxicated patient without the odor of ethanol. Moreover, the presence of severe metabolic acidosis, large anion gap and a large osmolal gap (greater than 10 mOsm/kg), hypocalcemia, neurologic manifestations, and calcium oxalate crystals in the urine are virtually diagnostic of EG poisoning. Metabolic acidosis develops as a result of the production of glycolic acid and other acidic metabolites secondary to EG breakdown. The plasma anion gap, representing unmeasured anions, is elevated as a result of the accumulation of the anions of the acid metabolites. In addition, because EG, like other alcohols, is an osmotically active compound, it contributes to the high osmolar gap. Therefore, the presence of an osmolal gap is supportive of the diagnosis of EG poisoning. Osmolal gap is the difference between the measured serum osmolality as determined by freezing point depression and the osmolality calculated from the serum levels of sodium, glucose, and urea nitrogen (EQUATION 36.2).

The normal osmolal gap should be between 10 and 12 mOsm/kg. Each 100 mg/dL of EG will produce an osmolal gap of 16 mOsm/kg. An elevated osmolar gap is helpful in several ways. First, it suggests the presence of EG and/or other alcohols in the serum. Second, it helps in estimating the serum concentration of any of these alcohols. Finally, serial measurement is useful in monitoring changes in EG level during removal by HD. However, a normal osmolal gap does not exclude EG intoxication as mentioned earlier. This is because only the parent compound is osmotically active, but its half-life is about 3 hours; therefore, delayed assessment after ingestion may fail to detect an osmolal gap despite the presence of toxic levels of metabolites. However, under these circumstances, the presence of the acid metabolites will still engender metabolic acidosis and an elevated anion gap (FIGURE 36.6).
Three types of crystals can be seen in the urine of patients with EG poisoning. Calcium oxalate crystals are commonly detected in the urine of these patients and are highly suggestive of EG poisoning, particularly in the presence of hypocalcemia. This is seen in nearly 50% of the patients with EG intoxication. Urinary calcium oxalate crystals usually appear after a latent period of 4 to 8 hours; consequently, repeat urinalysis should be carried out after this period (131). These crystals can have the classic appearance of “envelopes” if they consist of calcium oxalate dihydrate or more commonly the appearance of needles or prisms if they consist of calcium oxalate monohydrate (FIGURE 36.8) (132). Hippuric acid crystals have also been reported in patients with EG poisoning (133,134). The absence of calcium oxalate crystals in the urine does not exclude EG poisoning. In the rare situation where a kidney biopsy is done, calcium oxalate crystal deposition within the renal tubules and interstitial tissue can be seen even in the absence of crystalluria. Occasionally, urine of patients with EG poisoning fluoresces under Wood’s lamp and was thought to be helpful in the diagnosis. This is because antifreeze, one of the most common sources for EG poisoning, contains sodium fluorescein, a marker geared for the detection of radiator leaks in motor vehicles (135). However, more careful studies have shown that determination of urine fluorescence is not a reliable screening tool for suspected antifreeze ingestion (136,137). Finally, the most conclusive method of diagnosis is direct measurement of serum or urine EG concentrations by gas chromatography. Unfortunately, this method is not available in most hospital laboratories, and measurement by referral laboratories may delay the diagnosis.
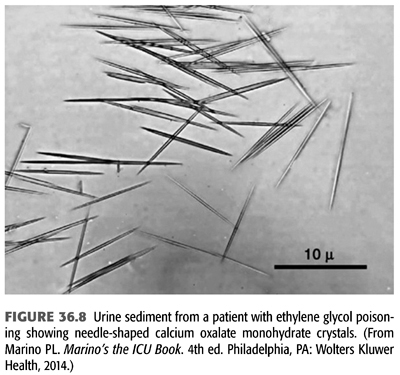
Treatment
Much of what has been discussed previously under treatment of methanol poisoning is applicable to EG poisoning. Again, treatment depends on the severity of the poisoning, depending on the dose ingested, time from ingestion to presentation, as well as on whether there was concomitant ethanol ingestion. Supportive measures such as securing the airway, breathing, and circulation are critically important. Due to the rapid absorption of EG from the gastrointestinal tract, gastric lavage may be beneficial but only within the first hour after ingestion, before toxic symptoms develop. Moreover, correction of severe metabolic acidosis with IV sodium bicarbonate therapy should be attempted. Aggressive treatment of acidosis and induction of an alkaline diuresis increase the renal elimination of glycolate and decrease the likelihood of AKI (138). Seizures, if present, can be treated with benzodiazepines and by correction of the severe hypocalcemia with 10 to 20 mL of 10% calcium gluconate given by slow IV infusion.
In addition to supportive care in all cases, administration of one of the two specific antidotes to delay further production of EG toxic metabolites is of paramount importance. Either ethanol or fomepizole can be used for inhibition of hepatic conversion of the parent compound by alcohol and aldehyde dehydrogenases, but they should not be used together. The indications for their use are listed in TABLE 36.15. Fomepizole, a competitive inhibitor of alcohol dehydrogenase, was approved for the treatment of EG poisoning by the U.S. Food and Drug Administration (FDA) in 1997. The Methylpyrazole For Toxic Alcohols (META) study group documented that fomepizole, if administered early in the course of intoxication, can prevent kidney injury (126). Brent et al. (126) conducted the only prospective clinical trial of fomepizole use in the treatment of EG poisoning. The drug was given intravenously at a loading dose of 15 mg/kg, followed by 10 mg/kg every 12 hours for four doses. Subsequently, it was given at as 15 mg/kg every 12 hours until the plasma EG concentration was less than 3.2 mmol/L (20 mg/dL). The mean time from ingestion to treatment was 11.4 hours. Seventeen of the 19 patients underwent HD, and 18 patients survived; 1 died of cardiogenic shock. Nine patients had normal renal function on presentation. The criteria for administration of fomepizole therapy are identical to those of methanol therapy (TABLE 36.12). As can be seen, because fomepizole is dialyzable, it is recommended to increase the frequency of its administration to every 4 hours during HD. Moreover, fomepizole should be given every 12 hours after the completion of dialysis until the EG level is less than 3.2 mmol/L (20 mg/dL) (108). Adverse effects are minimal and include bradycardia, seizure, dizziness, headache, and nausea (139).
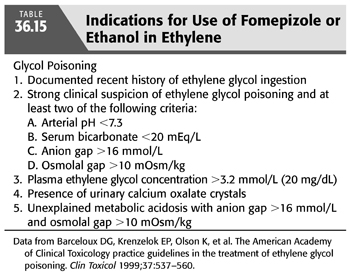
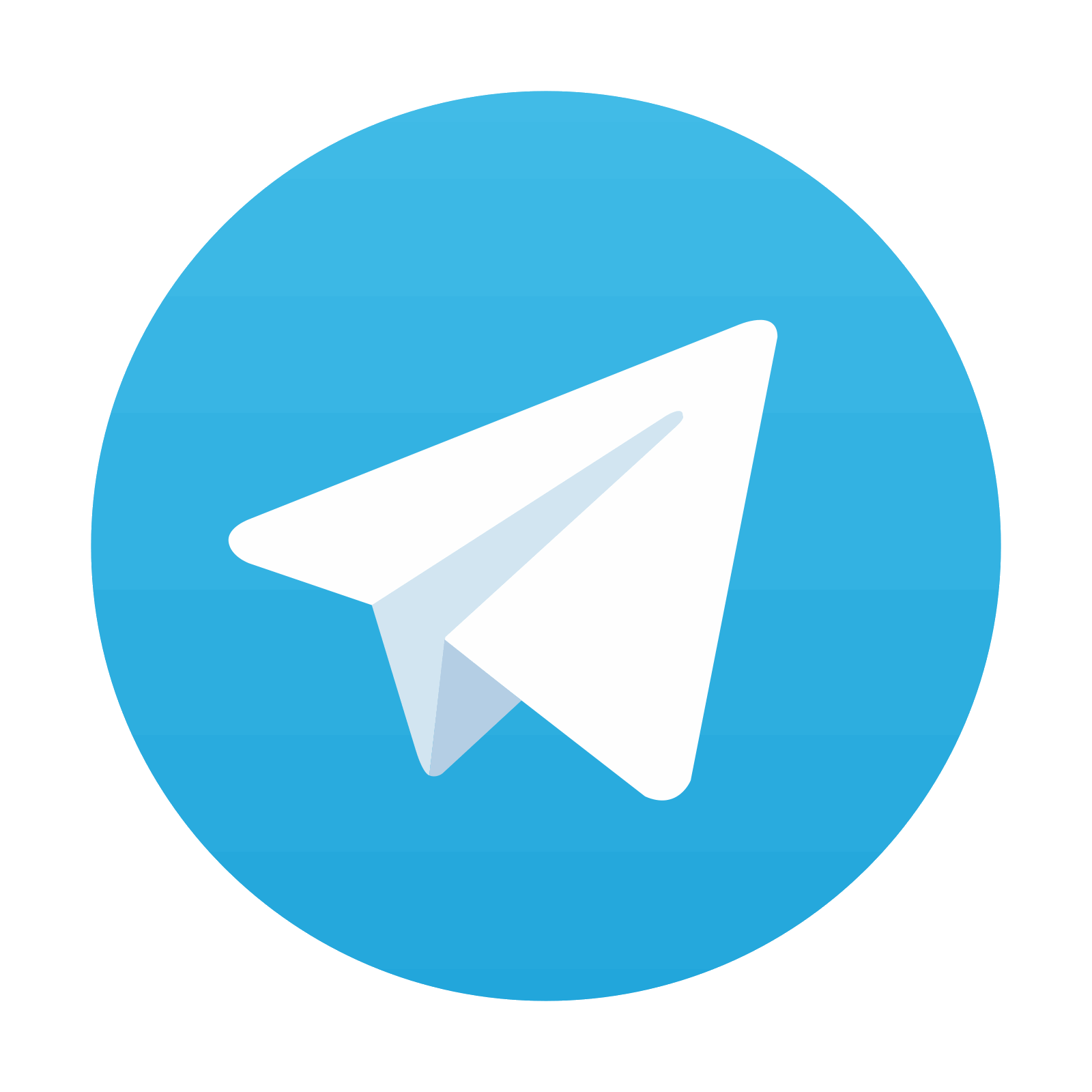
Stay updated, free articles. Join our Telegram channel
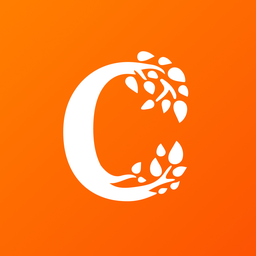
Full access? Get Clinical Tree
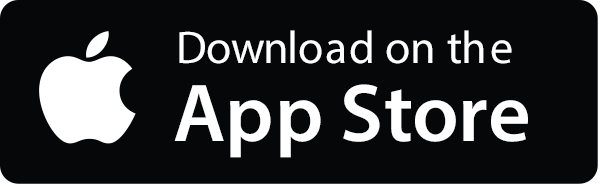
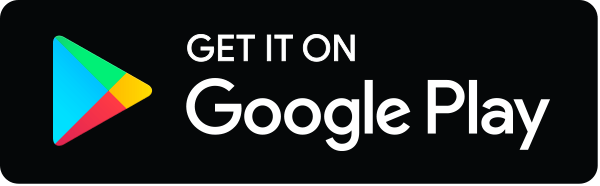