Epithelial Na + channels (ENaCs) have a key role in the regulation of extracellular fluid volume and blood pressure. Over the two decades since the initial characterization of ENaCs at a molecular level, investigators have identified key structural features within channel subunits and have defined mechanisms by which hormones and other factors regulate ENaC activity. Mutations within ENaC subunits have been identified that result in a gain or loss of function, which have profound effects on blood pressure. The identification of Liddle syndrome mutations led to the elucidation of a network of proteins, including Sgk1, GILZ, and Nedd4-2, which have important roles in the regulation of ENaC activity. The high-resolution structure of ASIC1, in combination with functional studies, has provided new insights regarding the role of the extracellular region in the regulation of ENaC gating.
Keywords
ENaC; Epithelia; Amiloride, Sodium; ASIC; Aldosterone; Nedd4-2; kinase
Introduction
From the late distal convoluted tubule, connecting tubule, and throughout the collecting duct, Na + exits the urinary space by passive diffusion through an apical membrane epithelial Na + channel, referred to as ENaC. Na + exits cells across basolateral membranes via the Na + ,K + -ATPase. Koefoed-Johnson and Ussing initially proposed this model of electrogenic transepithelial Na + transport, with diffusion of Na + across an apical membrane conductance pathway, in 1958. Studies using both noise analysis and single channel recordings demonstrated the presence of apical membrane Na + selective ion channels. Subsequent to the cloning of ENaC, knockout studies have confirmed the role of ENaC in the reabsorption of filtered Na + in the distal nephron, as well as its role in facilitating renal K + secretion.
Electrophysiologic Characteristics
The basic electrophysiologic characteristics of epithelial Na + channels have been defined using both macroscopic and single channel studies, and are presented in Figure 30.1 . ENaCs are Na + – and Li + -permeable channels that exhibit negligible K + conductance, with a single channel conductance of 4 to 5 pS at room temperature with Na + as the charge carrier. These channels exhibit a slight increase in open probability under hyperpolarizing membrane potentials. ENaCs characteristically exhibit long open and closed times, in the order of seconds to tens of seconds, although a population of ENaCs has been described that has brief open times and long closed times that likely represent channels that have not been processed by proteases. Channels are blocked by submicromolar concentrations of amiloride, a pyrazinoylguanidine derivative. Amiloride is a weak base and has a pK a of 8.8 in water. It is the charged, or protonated, form of amiloride that blocks ENaC. Other organic weak bases, including triamterene, trimethoprim, and pentamidine also inhibit ENaC.

Biochemical and Molecular Characteristics of ENaC
Amiloride was first demonstrated to inhibit electrogenic transepithelial Na + transport in 1968. With an IC 50 of ~100 nM, amiloride and several related compounds proved to be highly selective Na + channel inhibitors. Prior to the cloning of ENaC subunits, several different approaches were used to isolate a Na + channel complex. The relationship of this channel complex to ENaC is still unclear, although antibodies raised against a subunit of the cloned Na + channel have been observed to recognize a polypeptide within the purified channel complex.
The molecular characteristics of this channel were elucidated in studies from Canessa and co-workers and Lingueglia and co-workers in 1993 and 1994. An expression cloning technique led to the identification of a cDNA, termed α ENaC, whose cRNA induced expression of amiloride-sensitive Na + currents when injected into Xenopus oocytes. However, Na + currents were lower than expected. Two subsequent cDNA clones were isolated based on their ability to complement α ENaC cRNA in the expression of amiloride-sensitive Na + currents in Xenopus oocytes, and were termed β and γ ENaC. Xenopus oocytes co-injected with the three cRNA species expressed amiloride-sensitive Na + channels with characteristics nearly identical to that of Na + channels expressed in renal cortical collecting tubules and in cultured cell lines derived from the distal nephron. Na + currents were not observed when either the β- or γ-subunits were expressed alone.
The three ENaC subunits are likely derived from a common ancestral gene, given their limited (~30 to 40%) sequence identity. ENaC subunits have been cloned from a variety of species, including rat, human, mouse, rabbit, guinea pig, chicken, cattle, sheep, salamander, clawed African frog ( Xenopus laevis ), and bullfrog. The human α-subunit gene Scnn1a spans 17 kb on human chromosome 12p13. The β- and γ-subunit genes Scnn1b and Scnn1g are closely linked on human chromosome 16p12-p13. The genes encoding the three ENaC subunits have a conserved exon–intron architecture, with 13 exons. Splice variants have been described that alter the cytoplasmic N-termini or alter the extracellular domains. Variants that result in a termination codon in the region coding the extracellular domain of the α-subunit have also been reported. Some of these variants are associated with a reduction or loss of channel activity when expressed in heterologous systems, and with an autosomal recessive loss of function phenotype (pseudohypoaldosteronism) in humans.
ENaC/Degenerin Gene Family
Canessa and co-workers noted that ENaC subunits were related to genes identified in Caenorhabditis elegans that participate in mechanosensation specific mutations of which result in degeneration of selected neurons. ENaCs belong to the ENaC/Degenerin gene family ( Figure 30.2 ). There are two additional ENaC subunits, referred to as δ and ε, that appear to be functionally related to the α-subunit. δ ENaC is a proton-activated channel expressed in primates, although its physiologic role is still unclear. The ε-subunit was identified in Xenopus , and has an altered Na + self-inhibition response suggesting altered gating properties. Members of this family also include genes identified in C. elegans that are involved in mechanosensation ( mecs and degs ) or control of defecation rhythm ( flrs ); H + -gated channels (referred to as acid-sensing ion channels (or ASICs)) that are expressed in mammalian central and peripheral nervous systems and have a role in nociception, mechanosensation, fear-related behavior, and seizure termination; a family of 16 genes expressed in Drosophila , referred to as pickpocket, that may have roles in airway fluid clearance, mechanosensation, salt sensation, and detection of pheromones; and peptide-gated channels expressed in marine snails.

Structure and Function of ENaC
Each ENaC subunit has only two predicted membrane spanning domains, similar to the topology of members of the Kir family of K + channels, and members of the P2X family of purinergic receptors that are ligand-gated ion channels ( Figure 30.3 ). Three independent groups published topologic analyses of ENaC subunits. Each subunit has intracellular amino and carboxyl termini and two α helical membrane spanning domains connected by a large extracellular domain. The cytoplasmic domains have kinase phosphorylation sites, as well as protein–protein and protein–lipid interaction motifs that affect channel gating or trafficking.

Jasti and colleagues resolved the crystal structure of ASIC1, a member of the ENaC/Degenerin family. Although initially lacking the N- and C-terminal cytoplasmic regions, this structure provided important insights into the structural organization of ASIC and related family members, including ENaC. ASIC1 is a trimer, suggesting that ENaC has a subunit stoichiometry of α1β1γ1, in contrast to the higher ordered stoichiometry that had been proposed based on biophysical and biochemical studies. The extracellular region is a highly ordered structure that resembles an outstretched hand containing a ball, and has clearly defined domains termed wrist, finger, thumb, palm, knuckle, and β-ball (see Figure 30.3 ). Jasti et al. suggested that ASIC1 proton-dependent gating occurs in conjunction with conformational changes within the thumb and finger domains, which are transmitted to the transmembrane domains. The three ENaC subunits contribute residues that line the channel’s pore. The resolved ASIC1 structure suggests that the channel’s gate resides in the outer part of the pore, in the vicinity of the “degenerin” site where the introduction of bulky residues in: (1) ENaC subunits; (2) mec4 or mec10 (a component of the mechanosensitive channel in C. elegans ); and (3) ASIC subunits result in a dramatic increase in channel open probability.
ASIC1 is the only member of the ENaC/Degenerin gene family whose structure has been resolved. This structure provides a starting point to generate models of ENaC subunits. Kashlan and co-workers have built models of the extracellular region of the α-subunit of ENaC based, in part, on homology to ASIC1. The extracellular regions of ENaC subunits and ASIC1 are reasonably homologous, except in the finger domain. For example, the α-subunit of ENaC has 73 additional residues when compared to ASIC (see ). The α-subunit is proteolytically processed at specific sites within its finger domain, releasing an inhibitory tract. To determine the molecular architecture of the α-subunit finger domain, sites within α ENaC that interact with an 8 residue inhibitory peptide derived from the portion of the α-subunit that is removed by furin cleavage were determined. The working hypothesis was that the inhibitory tract within a non-cleaved α-subunit and the 8 residue inhibitory peptide share common binding sites. Distance constraints were introduced to construct a model of the α-subunit. The model places the inhibitory tract at an interface of the finger and thumb domains. Kashlan and co-workers suggested that the inhibitory tract blocks channel activity by stabilizing the movement of the finger domain relative to the thumb domain.
Stockand and co-workers have also generated a model of an ENaC trimer, based on the structure of ASIC1. To generate the model areas that lacked sequence similarity within the finger regions of ENaC subunits were removed. Although this limits the utility of the model, the model provides important insights regarding sites of intersubunit interactions.
ENaC Biogenesis
Na + channel subunits likely undergo assembly in the endoplasmic reticulum (ER), where core, high mannose Asn- (or N)-linked glycans are added at specific sites. Each subunit is modified by N-linked glycosylation at multiple sites. For example, rat α-, β-, and γ-subunits have six, twelve, and five consensus sites (Asn-X-Ser/Thr) for N-linked oligosaccharide addition, respectively. Exit of assembled channels from the ER appears to be inefficient. A motif that facilitates the exit of ENaC from the ER was found in the proximal cytoplasmic carboxyl terminus of the α-subunit.
Channel subunits that do not exit the ER are likely degraded via proteasome-mediated ER associated degradation (ERAD). Integral membrane proteins such as ENaC are co-translationally inserted into the ER. The proper folding and assembly of polypeptides synthesized in the ER involves interactions with a variety of chaperone proteins. Specific chaperones that participate in ENaC folding and assembly or targeting misfolded subunits for degradation are starting to be defined. These include members of the luminal Hsp40s, the small heat shock protein alpha A-crystallin, and calreticulin. Cytoplasmic Hsp70s also have roles in channel trafficking, although at present it is unclear whether their roles are related to facilitating ER exit or post-ER trafficking events.
The half-life of newly synthesized subunits, determined by metabolic labeling/pulse-chase experiments, is approximately an hour, consistent with the notion that the majority of ENaC subunits synthesized within the ER are targeted for degradation via ERAD. A longer-lived pool of channels is apparent in pulse-chase studies, which likely reflects properly assembled channels that have exited the ER. Several groups have determined the half-life of channels that have reached the surface. The rate of degradation of channels that have reached the surface may be in the order of many hours to days, although some investigators have reported a shorter half-life for channels that have reached the cell surface of A6 cells and MDCK cells expressing exogenous ENaCs. These differences in the half-life of channels that have reached the cell surface could result from differences in cell type, and whether ENaCs are expressed endogenously or exogenously.
ENaC Processing in the Biosynthetic Pathway
As assembled ENaCs exit the ER, it has been thought that channels follow the route used by other proteins in the secretory pathway. This involves trafficking through the Golgi where most N-glycans are processed, and the trans-Golgi network where channels are sorted into endosomes that are delivered to the apical membrane. N-glycan processing is often monitored by the enzyme endoglycosidase H, an enzyme that removes high mannose N-linked glycans prior to processing events that occur in the medial Golgi complex.
N-glycans on all three subunits of assembled channels are modified to complex-type Endo H-resistant forms. Surprisingly, subunits with endoglycosidase H sensitive N-glycans have also been described. Hughey and co-workers reported that two distinct pools of ENaC subunits were expressed at the plasma membrane: subunits with processed N-glycans and cleaved α- and γ-subunits; and full-length subunits that have non-processed N-glycans. Processing of subunits within a channel complex appears to be an all-or-nothing event. These findings suggest that a population of channel complexes exiting the ER transits through Golgi and post-Golgi compartments where subunits are processed ( Figure 30.4 ). These processed channels likely represent the pool of active, functional channels. A distinct population of channels exiting the ER appears to bypass Golgi and post-Golgi processing events. This distinct pool of non-processed channels is likely a functionally inactive pool, as proteolysis of ENaC subunits appears to have a dramatic effect on increasing channel open probability. Proteolytic cleavage of these inactive channels provides a potential mechanism to increase rates of Na + transport in the distal nephron. The role of proteolysis of ENaC subunits in regulating channel gating is discussed in detail later in this chapter.

Intracellular Trafficking of ENaC
Functional channels are delivered to the apical plasma membrane via the traditional secretory pathway ( Figure 30.4 ). The exocytic insertion of channels into the apical plasma membrane occurs as a regulated process that is increased in response to a variety of hormones, including vasopressin, aldosterone, and insulin. Vesicle and target SNAREs (soluble N-ethylmaleimide sensitive factor attachment protein receptors) participate in this process, and overexpression of specific SNARE proteins disrupts the intracellular trafficking of ENaC subunits. Channels at the plasma membrane appear to reside within specific compartments. Several groups have reported that a population of channels resides within lipid-rich microdomains, referred to as lipid rafts, although other groups have not confirmed this finding. While lipid rafts have been reported to facilitate the co-localization of membrane proteins and signaling molecules, the functional consequences of ENaCs within lipid rafts are still unclear. ENaCs interact with cytoskeletal elements, including actin and α-spectrin, which may have a role in localizing the channel to the plasma membrane and in modulating ENaC activity.
The residency time of channels at the plasma membrane has been examined by several groups, with reported half-lives in the order of minutes to hours. Mutations within a carboxyl-terminal PY motif within the β- and γ-subunits are associated with increases in the half-life of the channel at the plasma membrane via mechanisms that are discussed later in this chapter. Internalization of channels from the plasma membrane has been proposed to occur via a dynamin-dependent process. Dynamin is required for clathrin-dependent endocytosis, as well as for caveolae-dependent endocytosis. Ubiquitin conjugation of defined lysine residues within ENaC subunits at the plasma membrane targets the channels for endocytosis, presumably via a clathrin-dependent mechanism ( Figure 30.4 ). Once internalized, some channels are targeted for degradation via proteasomes or possibly lysosomes. A significant fraction of the pool of endocytosed channels may undergo recycling to the plasma membrane in a regulated manner ( Figure 30.4 ). Specific deubiquitination enzymes have a role in removing ubiquitin from internalized channels, facilitating the recycling of channels to the plasma membrane.
Localization within the Kidney and Other Organs
The aldosterone-sensitive distal nephron is the final site of Na + reabsorption within the nephron. ENaCs are expressed in principal cells in the late distal convoluted tubule, connecting tubule, and through the collecting duct, and are the major pathway for Na + entry across the apical plasma membrane. In the more proximal segments of the aldosterone-sensitive distal nephron, Na + reabsorption via ENaC is coupled to K + secretion mediated by apical membrane K + channels, including Kir1.1 (or ROMK) and the large conductance Ca + -activated K + channel (maxi-K). ENaC-dependent reabsorption of Na + in the distal nephron has a major role in the control of extracellular fluid volume, blood pressure, and renal K + secretion.
The cellular localization of individual ENaC subunits may differ within the nephron. When maintained on normal laboratory diet, β- and γ-subunits were localized within an intracellular compartment in principal cells within the cortical and outer medullary segments of the rat aldosterone-sensitive distal nephron. One group reported that the α-subunit was localized primarily to apical part of principal cells, whereas other groups have observed either modest cytoplasmic localization or have failed to detect the α-subunit. Within the inner medullary collecting duct, all three ENaC subunits were localized primarily within an intracellular compartment. When placed on a low-Na + diet or following administration of aldosterone, all three subunits were expressed at the apical membrane of principal cells.
Mice lacking expression of the β- or γ-subunits or that have reduced expression of the α-subunit exhibit renal Na + -wasting. Mice that lack expression of the α-subunit are unable to clear airway fluids at birth, leading to death in the early postnatal period. Recent work suggests that the density of ENaC expression may be greatest in the connecting tubule, an early segment within the aldosterone-sensitive distal nephron that connects the distal convoluted tubule to the collecting tubule. Mice that lack expression of α ENaC beyond the connecting tubule are able to maintain Na + and K + balance, even in the setting of dietary Na + restriction or K + -loading.
In addition to its expression in the nephron, ENaCs are expressed within numerous other organs. They are expressed throughout the airways, as well as in both type I and type II alveolar cells. ENaC has a key role in the reabsorption of airway fluids. Maintaining an appropriate volume of airway surface liquids has an important role in facilitating mucociliary clearance. ENaCs are also expressed in the distal colon, sweat ducts, salivary ducts, inner ear, lingular epithelium, keratinocytes, lymphocytes, and vascular smooth muscle. ENaC expression has also been reported in endothelia and in various sites within the eye, including epithelia within the retina, lens, and pigmented ciliary body and iris. The functional roles of ENaCs within many of these tissues are unclear.
ENaC Structure and Function
Specific structural features within channel subunits have key roles in defining the biophysical properties of ENaC. All three ENaC subunits contribute to the formation of the conduction pore, as pore properties are altered by mutations within each of the three subunits. The published structures of ASIC1 reveal a homomeric trimer ( Figure 30.3 ). The structures of the extracellular domain (ECD) and transmembrane domain (TM) are well-defined, while the cytoplasmic amino- and carboxyl-terminal domains are not resolved in the crystal structures. The cASIC1 structures confirm a longstanding notion that the channel pore is formed largely by the second transmembrane domain (TM2s) that determines the basic biophysical properties of ENaC. Both the extracellular and cytoplasmic domains have roles in modulating channel-gating and trafficking. The functional roles of individual domains within ENaC subunits, and potential mechanisms of ion permeation, ion selectivity, channel-gating, and amiloride block are reviewed below with insights from the ASIC1 structures.
Functional Domains within ENaC Subunits
Amino-Terminus
The cytoplasmic amino-termini have regions that affect channel-gating, trafficking, and regulation by intracellular factors. Chalfant and co-workers identified a Lys-Gly-Asp-Lys tract within the rat α-subunit corresponding to residues 47–50, that may function as an endocytic signal that regulates the number of channels in the plasma membrane. A domain that affects channel-gating has been characterized within the distal portion of the amino-terminus of the α-subunit, which includes a highly-conserved His-Gly tract. A mutation in the corresponding Gly in the β-subunit was described in a patient with pseudohypoaldosteronism. Reduced channel activity attributed to a decreased open probability was observed with a mutation of the conserved Gly in each subunit, suggesting that the His-Gly tract within the amino termini of all three subunits influences channel-gating. A recent study showed that mutations of this Gly in the α and γ ENaC subunits induced a strong inward rectification in the whole cell currents, reflecting voltage-dependent gating. Mutations at other sites have also been found to reduce channel activity and induce voltage-dependent gating.
Phosphatidylinositol 4,5-bisphosphate (PIP2) activates ENaCs, an effect that reflects an increase in channel open probability as a result of direct interactions between PIP2 and ENaC. Several groups have proposed that the amino-termini of the β- and γ-subunits of ENaC harbor putative PIP2-binding domains containing basic amino acid residues. In addition, Helms and co-workers suggest that PIP3 mediates aldosterone-induced ENaC activity and trafficking, and interacts with γ ENaC. The ENaC amino-termini also contain Cys residues that may interact with intracellular metals (Cd 2+ and Zn 2+ ) and thiol-reactive chemicals, leading to a reduction in channel open probability. Palmitoylation of amino-terminal Cys-43 and carboxyl-terminal Cys-557 of mouse β ENaC enhances channel open probability (see below).
First Transmembrane Domain (TM1)
The ASIC1 structures were apparently resolved in a desensitized state. In these structures, the channel pore is primarily lined by TM2 helices from three identical subunits, with the three TM1 helices packed tangentially behind the TM2 helices and contacting the lipid bilayer. The TM1 helix from an individual subunit also makes extensive interactions with the TM2 helix of the same subunit, as well as specific contacts with helices from an adjacent subunit. The closed-pore conformation of ENaC may be similar to that of ASIC1. A Trp scanning of the TM1 of α ENaC showed that mutations within the amino-terminal portion of αTM1 alter channel activity, selectivity, and gating, consistent with the extensive intrasubunit interaction between TM1 and TM2 helices revealed in the ASIC1 structures. A recent Cys scanning of ASIC1 TM domains is also consistent with the relative locations of TM1 and TM2 helices in the resolved structures.
Extracellular Domain (ECD)
Each ENaC subunit has a large, ~450 residue extracellular region with 16 conserved Cys residues clustered within two Cys-rich domains. Other members of the ENaC/DEG family also possess extracellular Cys-rich domains. The size and the apparently conserved structural organization of the extracellular domain suggest that this region has important functional roles. Recent studies have examined the role of the ECD in modulating channel-gating in response to proteases, sheer stress, external Na + , metals, H + , and Cl − . These functional studies and insights from the ASIC1 structure suggest that the ECD of ENaC functions as a sensor or receptor for a variety of extracellular signals. The extracellular domains also facilitate the assembly of the heteroligomeric channel complex within the endoplasmic reticulum, and influence intracellular trafficking.
Several proteases, including prostasin and related channel-activating proteases, furin, trypsin, chymotrypsin, and elastase have been shown to activate ENaC. Activation of ENaC by proteases is a result of proteolytic cleavage of ENaC subunits within their extracellular domains at defined sites, releasing intrinsic inhibitory tracts. Mechanisms by which proteases activate ENaCs are discussed below.
ENaCs are inhibited by extracellular Na + , which presumably binds to sites within the ECD. This response, referred to as self-inhibition, reflects a reduction in channel open probability. The structural basis of Na + self-inhibition has begun to emerge from mutagenesis studies. At present, the sites of Na + -binding, as well as the subsequent allosteric changes that lead to a reduction in channel open probability, have not been defined. In addition to Na + , other external cations such as Ni 2+ , Zn 2+ , Cu 2+ , Cd 2+ , and Hg 2+ affect ENaC activity, presumably by binding to sites within the ECDs and altering channel open probability. The effects of these metals on ENaC are both metal- and species-specific.
Extracellular anions also interact at sites within the extracellular domains of ENaC subunits and alter ENaC gating. Collier and Snyder found that external Cl − regulates ENaC activity in part through enhancing Na + self-inhibition. Their findings provide a mechanism by which changes in extracellular Cl − modulates epithelial Na + absorption. They identified two Cl − inhibitory sites in ENaC, one formed by residues in the thumb domain of the α-subunit and the palm domain of the β-subunit, and the other formed by residues at the interface of the thumb domain of the β-subunit and the palm domain of the γ-subunit. Based on the effects of mutagenesis on Cl − inhibition, the additive nature of mutations, and on differences in the mechanisms of Cl − inhibition, the authors propose a model in which ENaC subunits assemble in an αγβ-orientation when viewed from above ( Figure 30.3 ). This subunit arrangement is also suggested by Chen et al., based on the identification of a putative Cu 2+ -binding site at the α- and β-subunit interface within the ECD of human ENaC. However, the model is based on the assumption that the functional ENaC complex is a heterotrimer. The possibility that multiple arrangements co-exist needs to be tested.
Within the ENaC extracellular domains are 16 Cys residues, which are largely conserved among other members of the ENaC/degenerin family. Firsov and colleagues proposed that specific Cys residues (the first and sixth Cys in all three subunits, as well as the eleventh and twelfth Cys in the α- and β-subunits) have an essential role in the efficient transport of assembled channels to the plasma membrane. A mutation of the first cysteine residue in the human α-subunit (aC133Y) is associated with PHA-1. Sheng et al. proposed that there are several intrasubunit disulfide bonds, based on analyses of additivity of double Cys mutations and responses to sulfhydryl reagent of single and double mutants. These predicted disulfide bonds (Cys1-Cys6, Cys4-Cys5, Cys7-Cys16, Cys11-Cys12, Cys10-Cys13, and Cys8-Cys15 (conserved Cys numbered sequentially)) were present in the resolved ASIC1 structure. ASIC subunits have 14 conserved Cys residues within the ECD that form seven intrasubunit disulfide bonds, one in β-ball, one in palm, and five in thumb domain. The locations of the disulfide bonds are consistent with their roles in stabilizing the conformations of these domains.
Second Transmembrane Domain (TM2)
The TM2 region is thought to be the segment that lines the conduction pore and contains important functional sites, including the degenerin (DEG) site, amiloride-binding site, and selectivity filter site ( Figure 30.5 ). The structure of the ENaC pore is expected to be similar to that of ASIC1, given the high degree of sequence homology and similar biophysical properties. For example, three ENaC TM2s likely line the channel pore, while TM1s contact the lipid bilayer. As the resolved ASIC1 structures likely represent non-conducting states, they provide limited information regarding the roles of TM2 residues in ion permeation and selectivity. Two distinct TM domain structures of ASIC1 have been described, adding an additional layer of complexity in interpreting structure and function relationships of the TM2 domains from the resolved ASIC1 structures. Here we discuss mutagenesis results, mainly in reference to the most recent ASIC1 structure.

Amiloride Binding
Schild and co-workers identified a ring of residues at homologous positions within each of the three subunits (αS583, βG525, and γG537 in rat ENaC) as a putative amiloride-binding site ( Figure 30.5 ). The substitution of βG525 or γG537 weakened amiloride IC 50 by about three orders of magnitude. In contrast, the substitution of αS583 with amino acids bearing side chains with different functional groups (e.g., Gly, Leu, Asn, Gln) had only a slight effect on amiloride block, suggesting that the side chain of αS583 does not participate in amiloride binding. However, amino acids with aromatic side chains introduced at αS583 led to a large reduction in amiloride affinity, suggesting that large side chains at position α583 protrude into the pore, and limit access of amiloride to this site. The effects of Cys substitutions at the amiloride-binding ring on the inhibitory constant largely reflected an increase in the microscopic k off rate, consistent with an effect on the bound complex. An adjacent three residue Gly/Ser-X-Ser tract that forms the primary selectivity filter may also have an important role in amiloride binding. Mutations introduced in the first position of this tract in the α- or β-subunit were associated with a large reduction in amiloride affinity, suggesting that amiloride also interacts with this site.
The introduction of Cys residues at the amiloride-binding ring rendered channels sensitive to block by external Zn + -, Cd 2+ -, and sulfhydryl-reactive methanthiosulfonate (MTS) derivatives. Moreover, mutations of these residues reduced single channel conductance. This site was also shown to be located within the membrane electrical field. These observations suggest that this ring of residues line the conducting pore, as was observed in the homologous residue in the resolved ASIC1 structure.
Selectivity Filter
A three residue tract (Gly/Ser-X-Ser) starting four residues distal to the amiloride-binding site has a key role in conferring cation selectivity ( Figure 30.5 ). Systematic examination of the ENaC TM2 domains by several groups found that mutations of either the first and/or third residue within the Gly/Ser-X-Ser tract of the α-, β-, and γ-subunits resulted in significant K + permeation. Certain substitutions of the third residue within this tract in the α-subunit allow for permeation of divalent cations.
It is unclear whether backbone carbonyl oxygens or side chains within this three residue Gly/Ser-X-Ser tract face the lumen. Kellenberger et al. initially proposed that carbonyl oxygen atoms from third position Ser residues provide a Na + -binding site, based on a positive correlation between increases in K + permeability and the volumes of several substituted residues at αSer589. Alternatively, Sheng et al. proposed that the side chains of the selectivity filter residues faced the pore lumen, based on the sensitivity of channels with an introduced Cys at the first or third residue in the selectivity filter to externally applied Cd 2+ . This proposed side chain orientation is also consistent with the need for a hydroxyl group at position α589 to prevent K + permeation. The dispute regarding the orientation of residue side chains within the selectivity filter will likely remain until a high-resolution structure of ENaC or ASIC in an open state is available.
The distal (C-terminal) portions of the TM2 domains have three well-conserved negatively charged residues that likely line one face of an α helix. The introduction of mutations at specific sites containing acidic residues has been reported to reduce channel activity without altering surface expression and result in K + permeation, suggesting that some of these residues may also have a direct or indirect role in conferring cation selectivity.
Channel Gate
A five residue motif (G 432 DIGG 436 ) in the outer part of the ASIC1 TM2 domain has been proposed to function as the desensitization gate ( Figure 30.5 ). Gly432 is conserved among ASICs and is homologous to Ala442 in MEC4, the degenerin site where substitutions with a bulky residue result in degeneration of specific neurons in Caenorhabditis elegans . The three ENaC subunits bear a Ser at this site. Mutations at the degeneration site in MECs, ASICs, and ENaCs have the common phenotype of an increase in channel open probability, suggesting similar gating machinery. ENaCs with a Cys substitution at the degenerin site are activated by externally applied sulfhydryl reagents. Modification of Cys residues at the degenerin site is dependent on channels being in an open state, suggesting that either: (1) there is an extracellular gate controlling access to the degenerin site or (2) that this site undergoes a conformational change in association with channel-gating that alters the accessibility of the cysteine side chain to chemical reagents. The desensitization gate of ASIC1 is formed by a helical bundle crossing, which favors the second possibility.
In addition to the degenerin site, external sulfhydryl reagents activate channels with Cys substitutions at nearby sites with a periodicity suggestive of an α-helical structure, suggesting that an extended helical region functions as a gating domain. Consistent with the hypothesis, the sulfhydryl reactive agent MTSET transitions channels with an introduced Cys distal to the α-subunit degenerin site to a high open probability state. Furthermore, these modified channels lose their response to external Na + and to laminar shear stress. Carattino and co-workers reported that mutations at multiple sites within the TM2 of the α-subunit altered the magnitude and time course of ENaC activation by laminar shear stress, suggesting that this region has a role in the channel’s response to external mechanical forces. While ENaCs do not undergo desensitization, these results suggest that the primary gate within ENaCs is located in the same region as the ASIC1 desensitization gate.
Previous studies also suggest that there are additional sites within the TM1 and TM2 domains that affect rates of ion permeation or channel-gating. Several amiloride-binding site mutants (see above) exhibited an altered single channel conductance, although cation selectivity was preserved. Moreover, channels with a cysteine residue at the γ-subunit amiloride-binding site (γG537) had a high open probability, failed to respond to shear stress stimulus, and lacked a Na + self-inhibition response. These results suggest that the αS583-βG525-γG537 ring participates, either directly or indirectly, in ion permeation and channel-gating, in addition to its role in amiloride-binding. The carboxyl-terminal portion of TM2 may also have a role in the regulation of gating.
Structure of the Pore
Analogous to the ASIC1 pore structure, the ENaC pore is likely lined primarily by the TM2 helices. The TM1 helices may insulate the TM2 helices from the lipid bilayer. However, uncertainties regarding the ENaC pore structure remain, due in part to the distinct pore structures of the two ASIC1 structures that apparently were resolved from channels in the desensitized state. It is not clear which structure represents the native pore conformation and the extent to which structural distortion was generated by crystal lattice contacts. It remains possible that these two structures represent two native conformations that are captured at different stages of desensitization. The TM domains in the original structure are asymmetric with the TM2 helices of two of the subunits showing a ~30–33° kink at Gly 435, with the third subunit being nearly straight. The kinks bring the TM2 helices close together, allowing Leu 440 side chains to occlude the pore and separate the outer and inner vestibules. In contrast, the second structure has a symmetric pore design with all TM helices tilted at ~50° relative to the membrane normal, with the TM2 helices crossing at the proposed desensitization gate (G 432 DIGG 436 ) that separates the extracellular and intracellular vestibules. The defined degenerin site is located at the top of the gate. Assuming that the ENaC pore has a similar structure, the amiloride-binding and selectivity filter sites would be located within the intracellular vestibule. Mutagenesis studies of the ENaC pore are consistent with an asymmetric open state pore structure.
Cytoplasmic Carboxyl-Terminus
The region immediately following TM2 contains clusters of basic residues that effect channel-gating through interactions with acidic phospholipids, such as PIP3, as well as cation selectivity. A major function of the carboxyl-termini appears to be related to the internalization of the channel complex, through a well-characterized Pro-Tyr (PY) motif (PPPXYXXL) that interacts with the ubiquitin ligase Nedd4-2. ENaC subunit ubiquitination serves as a signal for channel internalization from the plasma membrane. It is unclear whether the YXXL sequence within this motif serves as an independent signal for channel internalization. Other sites within the carboxyl termini may influence rates of channel endocytosis. For example, an A663T polymorphism in the α-subunit of the human ENaC affects rates of channel internalization from the plasma membrane. ER exit of ENaC is regulated by a signal within the α-subunit carboxyl cytoplasmic tail.
The carboxyl-termini have sites that are targeted by specific protein kinases that modulate ENaC activity via phosphorylation. These kinases include an extracellular regulated kinase (ERK), casein kinase 2 (CK2), a serum and glucocorticoid regulated kinase (SGK1), and a G-protein-coupled receptor kinase (Grk2). ERK1/2-dependent phosphorylation of the carboxyl-termini of the β- and γ-subunits enhances ENaC’s interactions with Nedd4. Both forskolin and phorbol 12-myristate 13-acetate (PMA) have been reported to enhance phosphorylation of the β- and γ-subunits, although the target residues that are phosphorylated have not been identified. A region in the proximal part of the carboxyl-terminus of the α-subunit has a role in conferring sensitivity to a staurosporine-sensitive kinase that has not been identified. The carboxyl termini also bind α spectrin and possibly actin, linking the channel to the cytoskeleton. Grk2 activates ENaC by phosphorylating the carboxyl terminus of the β-subunit, and prevents Nedd4-2-dependent inhibition of ENaC. Cysteine residues in the amino and carboxyl termini of ENaC subunits may participate in the inhibition of ENaC that is observed with intracellular thiol reactive reagents. Selected cytoplasmic cysteine residues in the β- and γ-subunits are targets of modified palmitoylation. β-Subunit palmitoylation modulates channel-gating, possibly by facilitating interactions between cytoplasmic domains and the plasma membrane.
Cation Permeation and Selectivity
While a detailed understanding of the molecular mechanisms underlying permeation and selectivity awaits a high-resolution structure of ENaC in the open state, there is a growing body of information regarding ion permeation and selectivity. Organic or inorganic cations larger than Na + are unable to pass through the channel, in contrast to voltage-gated Na + channels, suggesting that permeant cations must dehydrate to cross the narrowest part of the channel pore (i.e., the selectivity filter). Within the Gly/Ser-X-Ser tract that comprises ENaCs selectivity filter, backbone carbonyl oxygens or hydroxyl oxygens present on the side chains of Ser residues may coordinate permeating Na + or Li + ions.
The selectivity sequence of ENaC (Li + >Na + >>> K + , organic cations) suggests that the relative permeability of an ion is inversely related to its ionic size, a relationship consistent with a mechanism in which ENaC discriminates cations through molecular sieving. Alternatively, cation selectivity could be achieved by placing a negatively (or partially negatively) charged site with strong field strength that preferentially binds small cations within the channel pore. In this regard, the selectivity sequence for ENaC corresponds to sequence XI of Eisenman. This sequence was determined based on the presence of strong electrostatic interactions between ions and the selectivity site that override differences in dehydration energies for ions. Interestingly, the selectivity filter of a voltage-gated Na + channel has a high-field-strength anionic coordination site.
Selectivity filters appear to be flexible, not static, structures. A dynamic selectivity filter of ENaC has been suggested, and appears to be likely in the light of the resolved ASIC1 structures. Both ASIC1 structures likely represent a desensitized state, and the selectivity filter residues are not in close proximity. We speculate that these residues are brought sufficiently close in the open state to interact with permeating cations. A recent study of ASIC1 is consistent with this notion.
Mechanisms of ENaC-Gating
ENaC-gating is characterized by unusually long open and closed times (up to seconds or even tens of seconds) compared to other channels such as voltage-gated channels ( Figure 30.1 ). This gating pattern seems appropriate for this channel, given its major physiological role of mediating bulk Na + transport across the apical membrane of epithelial cells, for which slow transitions between open and closed states may be beneficial for transport efficiency. Transitions between open and closed states appear to occur spontaneously. Another feature of ENaC-gating is the high variability in open probability observed in patch-clamp recordings. Subunit composition and regulatory factors that affect gating may account for this variability. As discussed in detail below, proteolytic cleavage of ENaC subunits converts channels that have a low open probability to channels that exhibit either an intermediate or high open probability. Mechanical forces, external metals, and temperature have also been shown to affect channel-gating. Studies on Na + self-inhibition and effects of metals on ENaC-gating have raised the question of whether ENaCs should be considered a ligand-gated channel, similar to FaNaCh and ASIC.
A detailed understanding of ENaC-gating mechanisms is lacking, despite the identification of several sites within ENaC where the introduction of mutations affects ENaC-gating kinetics. These sites are present within the amino-terminal domain, the extracellular domain, TM2, and the intracellular carboxyl-terminal domain. However, it is unclear whether these different regions control channel-gating in an independent or coordinated manner, nor is it known whether there is a single gate or multiple gates. The location of the ENaC gate is another open question, although the region corresponding to the desensitization gate of ASIC1 is an attractive candidate.
Several recent studies have suggested that the pore helix of cation channels may be a central gating structure. Yeh and co-workers suggested that the regulation of TRPV5 (a member of the transient receptor potential channel subfamily)-gating by extracellular and intracellular protons is mediated by a rotational movement of the pore helix, and the subsequent closing of a gate within the selectivity filter gate. As the amino-terminal helical portion of the TM2 helices of ENaC participates in channel-gating, it is possible that rotation of the TM2 helix that is initiated by conformational changes at other sites of the channels alters ENaC-gating kinetics, as suggested for ASIC1. Li and co-workers proposed a gating motion for ASIC1 involving a straightening of the tilt of TM2 without significant rotation.
Permeation and gating of ion channels were proposed to be two independent processes 60 years ago. While previous studies have supported this concept, recent studies suggest that connections exist between permeation and gating. For example, channel gating is often modulated by permeant or blocking ions. Mutations within selectivity filters are associated with changes in gating kinetics in K + channels, voltage-gated Na + channels, and ENaC. Lu and co-workers provided evidence suggesting conformational changes of the selectivity filter contribute directly to the spontaneous gating of an inward rectifier K + channel (Kir2.1). Significant differences in the selectivity filter structure of KcsA K + channel were observed when the crystals were soaked in low-K + and high-K + solutions, suggesting that the selectivity filter of this channel is flexible. Molecular simulations using the high resolution structures of bacterial K + channels support this idea of a flexible selectivity filter. Conformational changes in the selectivity filter have been proposed to occur in the ligand-initiated gating of cyclic nucleotide-gated ion channels. A similar notion has been proposed for inward rectifier K + channels. We anticipate that future studies will examine whether ENaC’s selectivity filter also serves as a gate.
ENaC Regulation
ENaC is subject to a wide variety of regulatory influences that alter channel activity over long or short time periods in order to respond to the physiologic needs of the organism. In the kidney, these regulatory influences determine the final Na + concentration of urine, which may vary from virtually Na + free to >100 mM. Abnormalities in these regulatory mechanisms in the cortical collecting duct have been convincingly linked to excess Na + reabsorption and hypertension, when disordered regulation leads to gain-of-function; and salt-wasting, hypotension and hyperkalemia when abnormal regulation leads to loss-of-function. Altered or abnormal regulation of ENaC in the lung has been linked to abnormal alveolar fluid clearance in disease states such as cystic fibrosis, high altitude pulmonary edema, and acute lung injury. In many cases, studies of abnormal ENaC function in disease states have complemented basic observations concerning channel function made in experimental settings, and led to remarkable insights concerning molecular mechanisms of regulation of channel activity.
Channel activity is subject to regulation through alteration of one of its intrinsic kinetic properties, either its number, open probability or single channel conductance. Since significant changes in single channel conductance are not found under physiologic conditions, channel regulation may be considered primarily as a matter of alterations in channel number and/or open probability. Thinking about channel regulation from this perspective would seem to simplify the subject, and certainly provides a framework within which regulation may be considered. However, the subject remains enormously complex given the number of regulatory influences that may modify either channel number or open probability.
There has been a significant paradigm shift, in that we now recognize that “near silent” channels are present in the membrane, in addition to constitutively active channels. These “near silent” channels are capable of activation and may be viewed as the extreme case of open probability regulation, where channels move from very low to a measurable open probability, giving the appearance of increased numbers of channels. The levels of “near silent” channel expression in the distal nephron under various physiological and pathological conditions are currently unclear. Channel regulation serves to either enhance or diminish Na + reabsorption from luminal fluids of the distal nephron, lung or colon, in accord with the needs of the organism. Given the wide variations in rates of Na + reabsorption and luminal Na + gradients that this involves, other intrinsic regulatory influences are required to maintain constant cell volume and ion gradients. To respond to these needs under normal physiologic conditions, channel activity is regulated by a number of hormones, including steroids, vasopressin, and insulin; by a variety of accessory proteins; by kinases, proteases, methyltransferases, and other signaling mediators; by other channels such as CFTR; and by ion concentrations and pH.
Cellular Regulation
Ubiquitination and Deubiquitination
Liddle syndrome is a hereditary form of salt-sensitive hypertension associated with increased ENaC activity. The most common defects in ENaC primary structure leading to this disorder involve the proline-rich regions in carboxyl-termini of the β- or γ-subunit. Rotin and colleagues used this region of the β-subunit as bait in a yeast two-hybrid screen to identify proteins that interact with ENaC and might regulate ENaC expression. They isolated the protein Nedd4 (Neuronal precursor cells Expressed Developmentally Downregulated) using this technique. In a series of elegant studies they examined the role of this protein in the regulation of ENaC expression. Nedd4 is an E3 ubiquitin ligase composed of a C2 domain, three or four WW domains that are protein interaction modules, and a ubiquitin ligase Hect domain. The WW domains of Nedd4 bind ENaC, with the strongest interaction being between the carboxyl-terminus of the β-subunit and the third WW domain. The Hect domain is an E3 ligase that receives ubiquitin from an E2 protein and transfers ubiquitin to lysines on target proteins. The C2 region is a Ca + – and phospholipid-binding domain. It is not present on all Nedd4 isoforms and, based on oocyte studies, does not appear to be essential for inhibition of ENaC expression. This domain does, however, serve to localize Nedd4 to plasma membrane in response to an increase in cytosolic [Ca 2+ ], and mediates association with annexin XIIIb, which may be involved in apical membrane targeting in epithelia.
Nedd4-2, the isoform most active in binding ENaC, is detected in tissues that express ENaC. The Nedd4-2 WW domains interact in vitro with the proline-rich region of carboxyl terminus of the β- and γ- (and possibly α-) subunits of ENaC. Considerable evidence indicates that the interaction between Nedd4-2 and ENaC results in ubiquitination of the channel ( Figure 30.6 ). ENaC has been shown to be ubiquitinated in endogenously expressing A6 cells, and when overexpressed along with Nedd4-2 in HEK-293 cells. Co-expression studies with Nedd4-2 and ENaC in oocytes demonstrated that Nedd4-2 decreases ENaC surface expression, and that this is dependent both on the E3 ligase domain of Nedd4-2 and on the presence of Lys residues on the amino-termini of the target subunits. Taken together, these studies strongly support the model that surface expression of ENaC is regulated by ubiquitination, which serves as a signal for retrieval from the plasma membrane. It is likely that ENaC is ubiquitinated at other cellular sites, including the endoplasmic reticulum. Unassembled subunits are likely degraded by the proteasome by a process involving polyubiquitination, while fully assembled trimeric channels are degraded by either the proteasome or lysosomal–endosomal pathway following ubiquitination at the cell surface. At present, it is unclear whether monoubiquitination of ENaC occurs at the cell surface. However, there is considerable evidence suggesting that ubiquitination is a signal for channel internalization from the plasma membrane, and that channel internalization is a dynamin-dependent, clathrin-dependent, as well as caveolin-dependent process.

The interaction between Nedd4-2 and ENaC is itself subject to regulation by specific hormones and kinases. The regulation of ENaC by Nedd4-2 represents a final common pathway of several regulatory influences affecting ENaC surface expression, providing further evidence for the importance of this interaction in ENaC regulation (see below).
Recent work has elucidated an important role for deubiquitinating enzymes (DUBs) in the regulation of ENaC expression and trafficking in cells. Specifically, the DUB Usp2-45 was found to be upregulated by aldosterone in mouse polarized collecting duct (mpkCCD c14 ) cells, which causes deubiquitination of ENaC, and an increase in ENaC surface expression and activity. The subcellular site(s) of action of Usp2-45 on ENaC and its precise physiological role are currently unclear. Butterworth and colleagues identified another DUB, UCH-L3, in the early endosomal compartment of these cells, which also deubiquitinates ENaC, and enhances the recycling of ENaC to the apical membrane. UCH-L3 may play an important role in reversing Nedd4-2-mediated ubiquitination of ENaC upon retrieval from the plasma membrane, thereby rescuing ENaC from degradation and promoting apical recycling of the channel ( Figure 30.4 ). Finally, Usp10 was recently identified as a vasopressin-stimulated DUB, leading to enhanced ENaC abundance and activity at the plasma membrane. In summary, DUBs may respond to hormonal inputs and appear to antagonize Nedd4-2-dependent ubiquitination and inhibition of ENaC, promoting ENaC stabilization and function at the plasma membrane.
Kinases
Protein phosphatase inhibitors, such as okadaic acid, activate ENaC, while nonspecific kinase inhibitors, such as staurosporine, inhibit basal channel activity, suggesting that kinases play an important role in regulating the channel. In many cases, the regulation of ENaC by a protein kinase is indirect, with kinase activation occurring within a pathway of hormonal regulation or cellular stress. Examples of this are: (1) SGK1 activation by steroid hormones induces SGK1-dependent phosphorylation of Nedd4-2 (the subsequent binding of 14-3-3 proteins to phosphorylated Nedd4-2 prevents the interaction of Nedd4-2 with ENaC subunits, and reduces ubiquitin-based retrieval of channels from the plasma membrane ) ( Figure 30.7 ); (2) insulin-dependent activation of phosphatidyl-inositol 3 kinase and 3-phosphoinositide-dependent kinase 1 results in the phosphorylation and activation of SGK1 and ENaC; (3) activation of protein kinase A by adenylate cyclase and cAMP in response to vasopressin or beta-adrenergic agonists leads to phosphorylation of Nedd4-2 at sites that overlap the SGK1 phosphorylation sites, and a reduction in ENaC endocytosis, as well as exocytotic insertion of Na + channels into the plasma membrane; (4) activated IKKβ, the kinase-regulating NF-κB, interacts with the cytoplasmic carboxyl terminus of β ENaC and phosphorylates Nedd4-2 at a residue that is also targeted by SGK1 and PKA, reducing channel endocytosis (IKKβ provides a mechanism of integrating inflammatory cascades and ENaC activation); (5) endothelin-dependent activation of Src kinase results in a decrease in channel open probability without directly phosphorylating channel subunits ; (6) selected protein kinase C isoforms also reduce channel open probability in a Ca + -dependent manner, protein kinase C also decreases expression of the β- and γ-subunits, which is dependent on activated ERK kinase ; (7) AMP-activated protein kinase (AMPK) is a ubiquitous metabolic sensor that phosphorylates Nedd4-2, enhances Nedd4-2 binding to β ENaC, and inhibits ENaC activity and surface expression.

ENaC is also a substrate for kinases that are involved in its regulation. Studies of ENaC expressed in MDCK cells have demonstrated increased phosphorylation of the β- and γ-subunits in response to stimulation by aldosterone and insulin. Phosphopeptide mapping indicated that the phosphorylated sites were carboxyl terminal Ser and Thr residues. Shi and colleagues described phosphorylation of Ser631 in the β-subunit and Thr599 in the γ-subunit by the pleiotropic but essential kinase CK2. Kunzelmann and colleagues found that a specific CK2 inhibitor blocked ENaC activity, and expression of a trimeric channel lacking both CK2 sites inhibited ENaC conductance and rendered ENaC insensitive to CK2. They concluded that phosphorylation by CK2 is essential for ENaC activation and partly regulates ENaC surface expression. Garty and colleagues also described phosphorylation of βThr613 and γThr623 by the extracellular regulated kinase (ERK). ERK-dependent phosphorylation of ENaC facilitates interactions between the channel and Nedd4-2, thereby inhibiting ENaC activity. SGK1 increases channel open probability by directly phosphorylating the carboxyl-terminus of the α-subunit at Ser-621. Finally, insulin has been described to increase phosphorylation of a fully mature 65 kDa form of α ENaC in cultured epithelial cells, which correlated with an increase in channel activity. The kinase-mediating of this effect was not directly identified, but the protein kinase C inhibitor chelerythrine blocked the insulin stimulation of transport and subunit phosphorylation.
It has also been reported that the G-protein-coupled receptor kinase, Grk2, phosphorylates Ser-633 in the carboxyl-terminus of the β-subunit. Phosphorylation at this site renders the channel insensitive to regulation by Nedd4-2, resulting in increased surface expression and channel activity. This finding is intriguing, as: (1) it is the first report of a G-protein receptor kinase directly regulating an ion channel; and (2) increased Grk2 activity has been associated with hypertension. Grk2 may also phosphorylate Nedd4-2, although the relevant site(s) and functional significance of this phosphorylation are unclear. A recent study suggests that Grk2 may also stimulate ENaC via a kinase-independent mechanism that requires its interaction with α-subunits of the Gq/11 family.
Another family of kinases linked to human hypertension is the WNK (with no lysine) family of serine-threonine kinases. These kinases are prominently expressed in the distal convoluted tubule, connecting segment, and cortical collecting duct of the kidney (i.e., the aldosterone-sensitive distal nephron), where they coordinate with angiotensin II and aldosterone signaling pathways. WNK mutations lead to hypertension and hyperkalemia typical of pseudohypoaldosteronism type II (PHA II). WNK4 inhibits the renal Na,Cl co-transporter (NCC) and ROMK, and mutations associated with hypertension relieve the inhibition of NCC, but enhance inhibition of ROMK. These observations suggested that under normal physiologic conditions, WNK4 regulates the balance between renal Na + reabsorption and K + excretion, but mutations would lead to exaggerated Na + reabsorption and hypertension with hyperkalemia, due to impaired K + excretion. In addition to NCC and ROMK, one group found that WNK4 also inhibits ENaC in vitro , and this inhibition by WNK4 is relieved by SGK1 phosphorylation near the WNK4 C-terminus. In contrast, another group suggested that WNK4 activates ENaC. Another member of this family, WNK1, has also been implicated in activating ENaC. Interestingly, intronic deletions that could lead to hypertension and hyperkalemia result in overexpression of WNK1. Aldosterone has also been shown to stimulate expression of a kidney-specific isoform of WNK1, and overexpression of this kinase stimulates Na + reabsorption in cultured collecting duct cells, and in overexpression systems. WNK1 expression activates SGK1 in a PI-3 kinase-dependent manner, indicating that the kinase activates ENaC by increasing its expression at the apical membrane of collecting duct cells, contributing to the human hypertension seen in association with PHA II.
As suggested above, the E3 ubiquitin ligase Nedd4-2 has emerged as a central convergence point for the regulation of ENaC and other transport proteins in cells. A growing number of kinases have been shown to phosphorylate Nedd4-2, modulate Nedd4-2 function, and thereby regulate ENaC. These include the aldosterone-induced kinase SGK1, vasopressin-stimulated PKA, the metabolic sensor AMPK, the NF-κB inflammatory mediator IKKβ, Grk2, and the insulin-stimulated kinase Akt1. In general, these kinases modulate the apparent binding affinity of Nedd4-2 for ENaC and/or 14-3-3 scaffolding proteins. Of note, additional putative Nedd4-2 phosphorylation sites have been recently identified by mass spectrometry that may be targets of the c-Jun N-terminal kinase (JNK1), and possibly other kinases. Unlike the above paradigm, where kinases regulate protein–protein interactions involving Nedd4-2, these novel target phosphorylation sites appear to play an important role in regulating Nedd4-2 catalytic function.
Proteases
Proteolysis of ENaC subunits has an important role in regulating ENaC activity. The first hint that proteases modulated ENaC activity was that serine protease inhibitors reduced transepithelial Na + transport across toad urinary bladder. Subsequent studies identified a channel-activating protease, or CAP, and demonstrated that extracellular trypsin activated ENaC by increasing channel open probability. Following these initial observations, other proteases have been identified that activate ENaC when either co-expressed with the channel in heterologous expression systems or when added to a solution bathing cells expressing ENaCs. These channel activating proteases include prostasin (also referred to as CAP1), TMPRSS4 (transmembrane protease serine 4 or CAP2), and matriptase (or CAP3), elastase, chymotrypsin, kallikrein, and plasmin.
Proteolytic processing of ENaC subunits occurs within the biosynthetic pathway. This is likely mediated by furin, a member of the proprotein convertase family of serine proteases that is expressed primarily in the trans-Golgi network. There are two furin cleavage sites within the extracellular domain of the α-subunit, and a single site within the extracellular domain of the γ-subunit. Furin-dependent cleavage of the α-subunit excises a 26 residue inhibitory fragment. Simply deleting this 26 residue tract from the α-subunit in the absence of subunit cleavage was sufficient to activate the channel. Furthermore, a synthetic peptide corresponding to the released fragment is a reversible channel inhibitor. An 8 residue tract, embedded within the 26 residue fragment, is responsible for the inhibitory properties of this fragment.
While the α-subunit is cleaved by furin twice, releasing an inhibitory fragment, the γ-subunit is cleaved only once by furin. As was observed with α-subunit processing by furin, cleavage at two sites spanning an inhibitory tract within the γ-subunit increases channel activity. While furin cleaves the γ-subunit at a site preceding an embedded inhibitory tract, multiple proteases have been shown to cleave the γ-subunit at sites distal to the inhibitory tract. Among the proteases that cleave the γ-subunit distal to the inhibitory tract are prostasin, TMPRSS4 (CAP2), elastase, and plasmin. Deleting the γ-subunit inhibitory tract dramatically increases channel activity, even in the absence of γ-subunit cleavage. An 11 residue tract is responsible for the inhibitory properties of the released fragment. With regard to channel activation, release of the γ-subunit inhibitory tract has a more profound effect than release of the α-subunit inhibitory tract.
At the single channel level, ENaCs exhibit a highly variable open probability. While ENaCs often exhibit open and closed times in the order of seconds to tens of seconds, a distinct population of ENaCs have very brief open times and long closed times. Caldwell and co-workers have shown that this latter population of channels responds to external trypsin, with a dramatic increase in channel open probability. These inactive channels likely represent a population of channels whose subunits have not been processed by proteases. Channels with α-subunit furin site mutations that have retained α- and γ-subunit inhibitory tracts have a very low open probability that reflects a dramatically enhanced reduction in ENaC activity in response to external Na + (referred to as Na + self-inhibition). When these channels are examined in the presence of a low external [Na + ], their activity dramatically increases.
Both processed (i.e., cleaved) and unprocessed subunits are expressed at the cell surface. These non-processed channels provide a pool of ENaCs that could be activated by proteases in a regulated manner. In this regard, rats placed on a low-Na + diet or receiving exogenous aldosterone have increased levels of whole kidney expression of the processed form of the γ-subunit.
The sites of protease cleavage in the α- and γ-subunits are located with the finger domain. A model of the α-subunit generated by Kashlan and co-workers suggests that the α-subunit inhibitory tract binds to sites at an interface of the finger and thumb domains. They proposed that the inhibitory tract reduces channel activity by stabilizing the movement of the finger domain relative to the thumb domain.
Syntaxins
The factors regulating ENaC delivery to the apical membrane in epithelial cells have not been fully-defined. The SNARE proteins (soluble N-ethylmaleimide sensitive factor attachment protein receptors) have been linked to directed exocytosis and intracellular trafficking in a number of tissues. A typical interaction involves binding of vesicle-associated v-SNARES to target membrane-associated t-SNARES. These interactions may be regulated by accessory proteins such as Munc 18. The t-SNARE syntaxin 1A has been demonstrated to interact directly with ENaC in co-immunoprecipitation experiments, and overexpression of this protein inhibited ENaC expression in oocytes. This effect was blocked by co-expression of Munc 18. These findings implicate the SNARE proteins in the process of exocytosis of ENaC, but are somewhat counterintuitive in that overexpression of the trafficking partners results in downregulation of the channel. The results suggest that the balance of t- and v-SNAREs may regulate this process, and that overexpression alters this interaction in a negative fashion.
The direct interaction of syntaxin 1A and ENaC suggests that there are cargo-specific interactions between SNARE proteins and apical membrane resident proteins such as ENaC. In a series of studies, Condliffe and colleagues identified domain-specific interactions between the carboxyl-termini of ENaC subunits and the H3 domain of syntaxin 1A. Interestingly, there was an effect of the H3 domain to decrease ENaC open probability, suggesting that SNARE proteins may regulate both channel exocytosis and gating. A more recent study suggests that syntaxin 1A regulates ENaC function by multiple mechanisms that include PKA, phospholipase C, PI-3 kinase, and MAP kinase signaling systems. Distinct inhibitory and stimulatory domains of syntaxin 1A interact with ENaC subunits, and the overall effect of syntaxin 1A on ENaC function depends on distinct physiological conditions.
Cystic Fibrosis Transmembrane Conductance Regulator (CFTR)
Patients with cystic fibrosis (CF) have increased amiloride-sensitive transport in airway epithelia. Indeed, increased ENaC activity in airway epithelia has been proposed as one mechanism for the drying of airway fluids, which promotes progression of airway disease. Lung specific overexpression of β ENaC or knockout of Nedd4-2 in mice results in a phenotype similar to CF airway disease in humans. CF is caused by function impairing mutations in the cystic fibrosis transmembrane conductance regulator (CFTR), an ATP-gated Cl − channel. On the basis of studies comparing ENaC activity in the presence and absence of CFTR expressed in MDCK cells, Stutts and colleagues proposed that CFTR functions as a cAMP-dependent regulator of ENaC, and the absence of this function in CF airways explained the increased ENaC activity in CF patients. Ling and co-workers demonstrated that inhibiting CFTR expression in a renal distal nephron cell line (A6) led to an increase in ENaC open probability. Studies in Xenopus oocytes or other overexpression systems have demonstrated that CFTR inhibits ENaC activity, although this has been disputed. However, several recent studies have questioned the role of CFTR in modulating ENaC activity. There is also evidence that ENaC enhances the activity of CFTR, due to an increase in the number of channels expressed at the plasma membrane, as well as an increase in CFTR open probability.
It is unclear if the interaction between CFTR and ENaC is indirect or results from a direct physical interaction. Changes in the intracellular [Cl − ], as well as electrochemical coupling, have been proposed as potential mechanisms by which CFTR regulates ENaC. One group has proposed that there are interactions between the regulatory domain of CFTR and ENaC, although the manner in which this interaction results in ENaC regulation is not clear. Moreover, ENaC–CFTR interactions appear to vary depending on the tissue of expression. For example, in sweat ducts, CFTR enhances ENaC activity. Although both CFTR and ENaC are expressed in collecting duct principal cells, no significant abnormalities of renal Na + handling have been described in CF patients. In airway epithelial cells, CFTR and ENaC co-immunoprecipitate, and interestingly expression of the most common CF-causing ΔF508 CFTR mutation was recently found to increase the proportion of cleaved to uncleaved α ENaC that was bound to CFTR. These findings suggest that wild-type CFTR may normally impede the proteolytic stimulation of ENaC in airway cells, although mechanistic details of how this may occur are unclear. Elastase is one of perhaps several proteases that are present in inflamed CF airways and could contribute to an increase in the extent of γ ENaC subunit proteolysis. Another recent study suggested that CFTR regulates the whole cell and surface expression of ENaC in airway epithelial cells, and that absence of this regulation may foster ENaC hyperactivity in CF airway epithelia. In summary, with current data it is difficult to explain the regulatory interactions between CFTR and ENaC solely on the basis of physical interactions or alterations in electrochemical driving forces or the intracellular Cl − concentration. The regulatory interactions may reflect changes in ENaC open probability (cleavage status) and surface expression, and vary among differing tissues, suggesting that other proteins or factors not yet recognized may be critical for functional CFTR–ENaC interactions.
Methyltransferases
Methylation reactions have long been implicated in the activation of ENaC by aldosterone. Aldosterone stimulates carboxylmethylation of proteins and phospholipids, and inhibition of these reactions blunts the ENaC response to steroid stimulation. A few potential targets of methyltransferases have been identified: k-ras, β ENaC, and more recently, histones involved in the aldosterone-dependent control of transcription. Aldosterone induces k-ras in a Xenopus distal nephron cell line (A6) cells, and this small G-protein is methylated by isoprenylcysteine carboxylmethyltransferase (PCMTase). PCMTase is not induced by aldosterone, but is regulated by the enzyme S-adenosyl-homocysteine hydrolase that is stimulated by aldosterone and results in increased activity of PCMTase. The enzyme (PCMTase) itself does not appear to stimulate ENaC, so it is unlikely to directly methylate the channel. Induction and processing of k-ras appears to be important for regulation of ENaC in A6 cells, but it is not clear that this occurs in mammalian tissues. Direct methylation of β ENaC has been demonstrated using a partially purified membrane preparation as a source of enzyme, but the enzyme itself has not been identified. Methylation of ENaC in planar lipid bilayers has been shown to lead to an increase in open probability of the channel. Edinger and co-workers identified a methyltransferase that activates ENaC when co-expressed with the channel in oocytes. Methylation does not appear to play a role in basal channel activity.
Several recent studies have explored the role of Dot1a, which has histone H3K79 methyltransferase activity and is widely expressed in the kidney, in the transcriptional control of α ENaC in response to aldosterone. Aldosterone releases repression of α ENaC by reducing expression of Dot1a and its partner AF9, and by impairing Dot1a–AF9 interaction via SGK1-mediated AF9 phosphorylation. This network also appears to regulate transcription of several other aldosterone target genes, including SGK1.
Calcium
Increases in intracellular calcium have been shown to inhibit ENaC activity by several groups. This appears to happen as a biphasic process with a very quick early response and a slower downregulation after 5 minutes. This is likely an indirect effect on the channel, as the activity of channels from cortical collecting ducts in excised patches exposed to increased cytosolic [Ca + ] was not altered. Activation of protein kinase C has been suggested to mediate the Ca 2+ -dependent inhibition of ENaC, since this is known to decrease channel activity. A second intriguing possibility, related to the delayed effect of Ca 2+ on ENaC activity, is Ca 2+ -dependent recruitment of Nedd4-2 isoforms that possess the Ca 2+ -binding C2 domain to the plasma membrane, with a subsequent increase in channel retrieval from the apical membrane.
pH and Oxidative Stress
Acidic intracellular pH, below 7.2, has been shown to decrease amiloride-sensitive Na + transport in isolated epithelial tissues, suggesting that intracellular pH may act as an intrinsic regulator of ENaC activity. In conditions of ischemia or hypoxia where intracellular pH might fall, activation of AMPK might act to decrease channel activity, as noted above. Hypoxia is known to decrease activity of ENaC through a decrease in channel expression in cultured type II alveolar cells, but the mechanism of this response is unknown. The effect of pH on channel activity appears to be direct. In excised, inside-out patches from apical membranes of rat cortical collecting tubule, a fall in pH from 7.4 to 6.4 resulted in a progressive and dramatic fall in open probability of the channel. The mechanism of this regulation is unknown. However, ENaC activity is clearly downregulated under cellular conditions where ATP is limiting. It has been proposed that ENaC may be sensitive to changes in intracellular redox potential through oxidation of intracellular cysteine residues. It has also been shown that transcription and expression of α ENaC subunit variants are suppressed by oxidative stress in lung epithelial cells. Conversely, oxidative stress induced by hydrogen peroxide exposure appears to stimulate ENaC activity through a PI 3-kinase-dependent pathway in cultured kidney collecting duct cells. Such oxidative stress-induced stimulation of ENaC has been proposed to contribute to the pathogenesis of salt-sensitive hypertension. Similarly, superoxide formation and release by NADPH oxidase (NOX2) downstream of epidermal growth factor and Rac1 has been reported to stimulate ENaC open probability in the lung.
Nitric Oxide
Several studies have shown that nitric oxide (NO) inhibits ENaC activity in both alveolar type II (AT II) cells and in cultured renal epithelial cells. This effect may be important in inflammatory conditions such as acute respiratory distress syndrome, where NO levels may be elevated due to increased expression of inducible nitric oxide synthase (iNOS). NO appears to inhibit the open probability of the channel in a cGMP-dependent manner. Interestingly, aldosterone has also been shown to inhibit NO production from ATII cells, and this appears to be related to an effect of SGK1. These results suggest a second, novel mechanism by which SGK1 could enhance ENaC activity through downregulation of iNOS activity, and a decrease in NO inhibition of ENaC open probability. In addition, the superoxide generation that occurs with oxidative stress appears to antagonize the NO-mediated inhibition of ENaC in lung slice patch-clamp experiments.
Lipids
A number of membrane lipids and lipid intermediates have been shown to modify ENaC activity, often in complex ways. Inhibition of phospholipase A2 (PLA2) by aristolochic acid reduced arachidonic acid (AA) levels and increased ENaC activity in both Xenopus oocytes and A6 cells in culture. Direct application of AA to rat cortical collecting ducts markedly reduced ENaC activity in a dose-dependent manner. While this effect was not reproduced by application of a non-metabolized analog of AA, 5,8,11,14-eicosatetraynoic acid (ETYA), it was reproduced by the CYP-epoxygenase metabolite 11,12-epoxyeicosatrienoic acid (EET). A recent study found that, along with 11,12-EET, 8,9-EET, and 14,15-EET are additional eicosanoids that inhibit ENaC activity downstream of CYP2C8. These results suggest that arachidonic acid effects are mediated by CYP epoxygenase metabolites. In contrast, in A6 cells current stimulation by PLA2 inhibition was blocked by ETYA, suggesting a direct effect of arachidonic acid on the channel, rather than the effect of a metabolite. The effect of ETYA on ENaC was to reduce open probability. In oocytes, however, both arachidonic acid and ETYA inhibited ENaC, and this was due to an alteration in the number of surface channels. Analysis of ENaC surface expression showed a downregulation of channels consistent with a trafficking effect mediated by both increased endocytosis and decreased exocytosis. Taken together, these experimental observations indicate that PLA2 activity leads to increased arachidonic acid levels that inhibit ENaC activity, but the precise molecular mechanisms remain in dispute.
Cellular phosphoinositides also affect ENaC function. Phosphatidylinositol-4,5-bisphosphate (PIP2) is a signaling molecule related to a number of intracellular processes, including endocytosis and exocytosis. It has also been implicated in the activation of several ion channels. Patch-clamp studies have shown that anionic phospholipids, including PIP2 and PI-3,4,5-P3, can directly alter channel activity presumably by binding to cationic sequences within β and γ ENaC. A distinct PIP3-binding site has been identified in the initial part of the carboxyl-terminus of the γ-subunit. PIP2 also directly interacts with ENaC at a site distinct from the PIP3 binding site, and may be permissive for channel gating. Interestingly, increases in cellular PIP2 levels have also been shown to increase surface expression of ENaC, presumably by stimulating exocytosis. Kunzelmann and colleagues demonstrated that purinergic inhibition of ENaC by extracellular ATP in tracheal epithelia resulted in depletion of PIP2 from cells, and appeared to require a PIP2-binding region of the amino-terminus of the β-subunit.
There are three isoforms of phosphoinositide-5-kinase, which produces PI-4,5-P2 from PI4P. These kinases have differing effects on cellular processes such as endocytosis. PIP2 may thus induce varying effects on ENaC function, depending on the isoform of PI5K that is activated or the spatial localization of kinases and phosphatases that regulate PIP2 activity within the cellular microenvironment. In summary, phosphoinositides functioning either as signaling agents or directly binding to ENaC affect channel activity.
Intracellular cytoplasmic Cys residues in β- and γ-subunits are modified by palmitoylation. Two Cys residues (Cys43 and Cys557) in the mouse β-subunit were shown to be modified by palmitoylation. Mutation of these Cys to Ala to prevent palmitoylation at these sites was associated with a reduction in channel open probability. Secondary structural predictions suggest that Cys557 is within an amphipathic α helix near the second transmembrane domain, while Cys43 is in proximity to the first transmembrane α helix. Mueller and co-workers suggested that β-subunit palmitoylation modulates channel-gating by enhancing interactions between cytoplasmic domains and the plasma membrane.
Intracellular Na +
Since ENaC is present in epithelia that generate a steep lumen-to-bath Na + gradient, it is potentially exposed to significant variations in both internal and external [Na + ]. ENaC is modulated by both intracellular and extracellular [Na + ]. Low levels of extracellular [Na + ] increase ENaC activity while elevated levels decrease activity, a process known as Na + self-inhibition. Cytoplasmic [Na + ] is usually maintained quite low through the action of the Na + ,K + -ATPase on the basolateral surface of epithelial cells. Since the pump is normally functioning well below its maximum capacity, Na + entry into cells is the rate-limiting step for Na + reabsorption. Under conditions of rapid increases in ENaC activity, regulation may also be achieved through increases in the intracellular [Na + ], a process referred to as feedback inhibition. This phenomenon has been observed in epithelial cells and Xenopus oocytes. Feedback inhibition in response to an increase in the intracellular [Na + ] is a slow process that occurs over a period of minutes, in contrast to Na + self-inhibition, which occurs over seconds. Studies in rat cortical collecting ducts suggested that PKC activation plays an important role in ENaC feedback inhibition. Studies in oocytes indicated that this feedback inhibition was not readily seen in cells expressing ENaC with Liddle mutations that inhibit Nedd4-2-binding and ubiquitin-dependent internalization. This finding focused attention on Nedd4-2 as the potential mediator of Na + feedback inhibition. In a series of studies in mouse mandibular duct cells, it was demonstrated that Na + feedback inhibition was dependent on Nedd4-2, and required binding of the second and third WW domains to carboxyl termini of β and γ ENaC. More recent studies have suggested that the A-kinase anchoring protein 15 (AKAP-15) may bind to PKC and act in concert with PKC to regulate Na + feedback inhibition or that intracellular [Na + ] may directly modulate the proteolytic activation of ENaC. In summary, a few potential signaling pathways/mechanisms have been implicated in ENaC feedback inhibition by intracellular [Na + ], although the relative contributions for each and how they may integrate with one another in different cell types are still unclear.
Extracellular Na +
Increases in extracellular [Na + ] inhibit ENaC activity, a process referred to as Na + self-inhibition. This phenomenon was originally observed in studies of native epithelial tissues in the setting of a rapid increase in extracellular Na + concentration. This process reflects a decrease in channel open probability, and is not dependent on Na + influx. Chraibi and Horisberger demonstrated that Na + self-inhibition is an intrinsic property of EnaC, and can be abolished by treatment with extracellular proteases. Na + self-inhibition is a temperature-dependent phenomenon and has a large activation energy, suggesting that a conformational change occurs in association with the inhibition of channel activity by extracellular Na + that is presumably initiated by Na + -binding to an extracellular site. Numerous sites within the extracellular domains of the α- and γ-subunits have been identified where mutations alter Na + self-inhibition. A number of important questions remain to be addressed regarding the Na + self-inhibition response, including: (1) where are the external Na + -binding site(s); (2) what are the conformational changes that occur in response to Na + -binding that result in a reduction in channel open probability; and (3) how do external proteases, presumably by cleaving ENaC subunits, diminish the Na + self-inhibition response?
Peroxisome Proliferators-Activated Receptors (PPARs)
The peroxisome proliferators-activated receptors (PPAR) have been implicated in the regulation of a wide variety of cellular processes. PPARγ is the pharmacological target of thiazolidinediones (TZDs) that have been used in the management of hyperglycemia associated with type II diabetes mellitus. PPARγ is localized along the collecting duct in kidney, and it is known that a side-effect of PPAR stimulation by TZDs is fluid retention. It has been shown that TZD-induced weight gain was prevented in mice by amiloride or by deletion of the gene encoding PPARγ from collecting duct. These studies suggested that TZDs increase ENaC activity in collecting duct cells, and increased mRNA for γ ENaC. These interesting results implicate PPARs in the regulation of transcription of at least one ENaC subunit that apparently leads to increased ENaC activity. More recent studies have suggested that the PPARγ-induced Na + reabsorption via ENaC in the kidney may be mediated through increased SGK1 expression. In contrast, work from other groups suggest that PPARγ agonists do not activate ENaC in cultured principal cells or decrease ENaC expression in the kidney.
ATP
Luminal ATP signals through P2Y2 receptors and inhibits ENaC activity through activation of phospholipase C, activation of protein kinase C, and reducing cellular levels of PIP2 and channel open probability. Recent studies suggest that this signaling pathway may have an important role in aldosterone escape, and the enhanced rates of Na + excretion in animals on a high-Na + diet. Cellular release of ATP may be occurring through connexin 30 hemi-channels.
Hormonal Regulation
Aldosterone
The major hormones regulating ENaC activity in a broad variety of tissues are corticosteroids. ENaC regulation by steroids is the subject of a separate chapter in this volume, and will not be discussed in detail here. Although non-genomic actions of aldosterone have been described in vascular and non-absorptive tissues, the bulk of the available evidence supports the notion that steroid regulation of ENaC in Na + -absorptive epithelia is mediated by processes dependent on transcription and translation. This transcriptional activity is driven by translocation of the steroid receptor to the nucleus following binding to its cognate ligand, and binding to specific domains within the genome. Interestingly, ENaC regulation varies somewhat by tissue. Steroid regulation of ENaC activity is largely due to an increase in the number of active channels in the apical membrane, although there is evidence for effects on open probability as well. The increase in the number of active channels is not apparently a simple one-step procedure. An early increase in channels appears to be a trafficking event, with altered insertion or retrieval of already synthesized subunits. A large part, but not all, of this effect is regulated by the aldosterone-induced proteins SGK1 and GILZ1 altering Nedd4-2-ENaC interactions and leading to increased membrane expression of the channel ( Figure 30.7 ). Synthesis and delivery of new channel subunits can be detected somewhat later in the course of steroid action in responsive tissues and, interestingly, also varies somewhat by tissue. In kidney, the predominant induced subunit is α, while in colon, the β- and γ-subunits are primarily induced. This is true both at the mRNA and protein level. This phenomenon has been referred to as non-coordinate regulation, and suggests a degree of complexity of ENaC trafficking that is still not fully-understood.
Vasopressin
Vasopressin has been shown to stimulate ENaC activity in the kidney and a number of epithelial cell lines derived from kidney. The response is relatively rapid, with a time course of minutes, and does not appear to depend on transcription or translation of new proteins, at least in its initial phase. Vasopressin binds to V 2 receptors on the basolateral surface of responsive epithelia, and activates adenylate cyclase. In almost all tissues studied, the action of vasopressin on Na + transport is fully reproduced by exogenous cAMP, and is felt to be secondary to activation of protein kinase A (PKA). PKA has not been shown to phosphorylate any subunit of the channel, so its actions are felt to be indirect. There has been no consistent demonstration of an effect of PKA on ENaC open probability, so it is likely that the primary effect of PKA on ENaC is to increase the number of channels at the plasma membrane. Indeed, there are now numerous demonstrations that PKA stimulation leads to an increase in surface expression of ENaC by biochemical, immunohistochemical, and electrophysiologic techniques. By analogy to its well-described effects on insertion of aquaporins in kidney cortex and medulla, it seems reasonable to expect that the primary event in PKA stimulation of ENaC is the exocytosis of channels into the apical membrane from some pre-existing cytoplasmic pool. Strong evidence supports this likelihood. Studies by patch-clamp indicate a rapid increase in the number of surface channels in response to cAMP stimulation. Stimulation of adenylate cyclase by forskolin or by addition of cAMP analogs leads to an increase in biochemical and immunohistochemically measured apical channel number, and this increase is temporally associated with an increase in apical membrane capacitance typical of exocytic events. Butterworth and colleagues have recently proposed that cAMP stimulation leads to exocytic insertion of channels from a subapical recycling pool of channels that is distinct from constitutive turnover of the channel, analogous to vasopressin regulation of water channels ( Figure 30.4 ).
It is also likely that vasopressin, acting through cAMP and Nedd4-2, alters channel retrieval. Snyder and colleagues reported that Nedd4-2 is a substrate for PKA phosphorylation, and they related cAMP regulation of ENaC to inhibition of Nedd4-ENaC interactions. Indeed, PKA appears to phosphorylate Nedd4-2 at sites that overlap the SGK1 target phosphorylation sites, suggesting a similar mechanism of action in promoting the sequestration of Nedd4-2 through binding to 14-3-3 proteins. There is an obvious interplay between the hormonal regulation of ENaC by aldosterone and vasopressin, and it is possible that it occurs at the point of kinase regulation. However, the fact that aldosterone and vasopressin are synergistic, and that ENaCs with Liddle’s mutations which should be unresponsive to Nedd4-2 inhibition are still responsive to vasopressin stimulation, suggests that, at a minimum, some distinct pathways are involved in the regulation of ENaC by these two hormones. It is also interesting to note that a long-term (days) effect of vasopressin to stimulate transcription of ENaC subunits would clearly complement and be synergistic with aldosterone effects in situations of significant Na + avidity.
Insulin
Insulin stimulates ENaC activity in renal epithelia. The exact mechanism(s) of this effect are a subject of considerable controversy. This would seem odd, as it is clear that insulin stimulates phosphoinositide 3-kinase (PI3K), which in turn regulates the activity of 3-phosphoinositide-dependent kinase 1. The latter kinase phosphorylates SGK1 and converts it to an active form. This represents an obvious convergence point for the effects of aldosterone and insulin, which have been shown to be synergistic, on the stimulation of ENaC activity. Indeed, noise analysis indicates that the primary effect of insulin is to increase the number of active apical membrane channels, with little effect on open probability. All of these observations are consistent with insulin increasing ENaC activity via decreasing Nedd4-2-dependent retrieval.
It is possible, however, that the action of insulin is more complex. Stimulation of ENaC activity by insulin is quite rapid (minutes), and has been associated with exocytosis and delivery of preformed channels to the apical membrane in a PI3K-dependent manner. Additionally it has been proposed that insulin may directly activate channels by phosphorylation that is dependent on protein kinase C or by interaction with active metabolites of PI3K, phosphoinositol 3,4 phosphate, and phosphoinositol 3,4,5 phosphate, both of which activate ENaC in excised patches. As with aldosterone and vasopressin, although a great deal has been learned about the regulation and trafficking of ENaC, the final control mechanisms by which hormones regulate the channel are complex and only partially-understood.
Angiotensin II
Angiotensin II activates ENaC by signaling through angiotensin II type 1 receptors. The signaling mechanism appears to involve activation of a Ca + -independent protein kinase C isoform and activation of NADPH oxidase, leading to an increase in channels at the plasma membrane, as well as an increase in channel open probability.
Endothelin
Endothelin-1 signaling through ETB receptors results in a decrease in ENaC open probability. The intracellular signaling pathways subsequent to ETB receptor activation involve Src kinase and MAP kinase 1/2 signaling pathways.
Regulation by Mechanical Forces
Members of the ENaC/DEG family expressed in C. elegans are mechanosensitive ion channels. Early studies directed at examining the mechanosensitivity of ENaC produced conflicting results that have led to lively debates. Different responses of ENaC expressed in Xenopus oocytes to cell swelling or shrinking have been reported. Application of a negative hydrostatic pressure to rat CCD cells by patch pipettes led to a variable increase in the open probability of Na + channels.
The distal nephron is subject to varying flow rates and volumes, dependent in part on extracellular volume status and the use of pharmacologic agents, such as loop and thiazide diuretics. Changes in flow rates within the distal nephron affect ENaC activity. Micropuncture and microperfusion studies of distal nephron segments have demonstrated that increases in flow rates within the physiologic range led to increases in the rates of net transepithelial Na + flux. Increases in tubular flow rates expose channels to a variety of mechanical forces, including hydrostatic pressure and shear stress. Carattino and co-workers demonstrated that ENaCs expressed in oocytes are activated when exposed to laminar shear stress at levels that are likely to be present at the surface of principal cells within collecting ducts exposed to flow rates within a physiologic range. The increase in ENaC activity is due to an increase in channel open probability. While mutations introduced at sites within the channel pore alter the channel’s response to shear stress, evidence suggest that the large extracellular regions function as flow sensors. ENaC subunits are also expressed in vascular smooth muscle, where they are also exposed to mechanical forces. ENaC subunits, possibly in conjunction with ASIC subunits, form mechanosensitive channels that function as arterial baroreceptors and participate in the myogenic response in vascular beds.
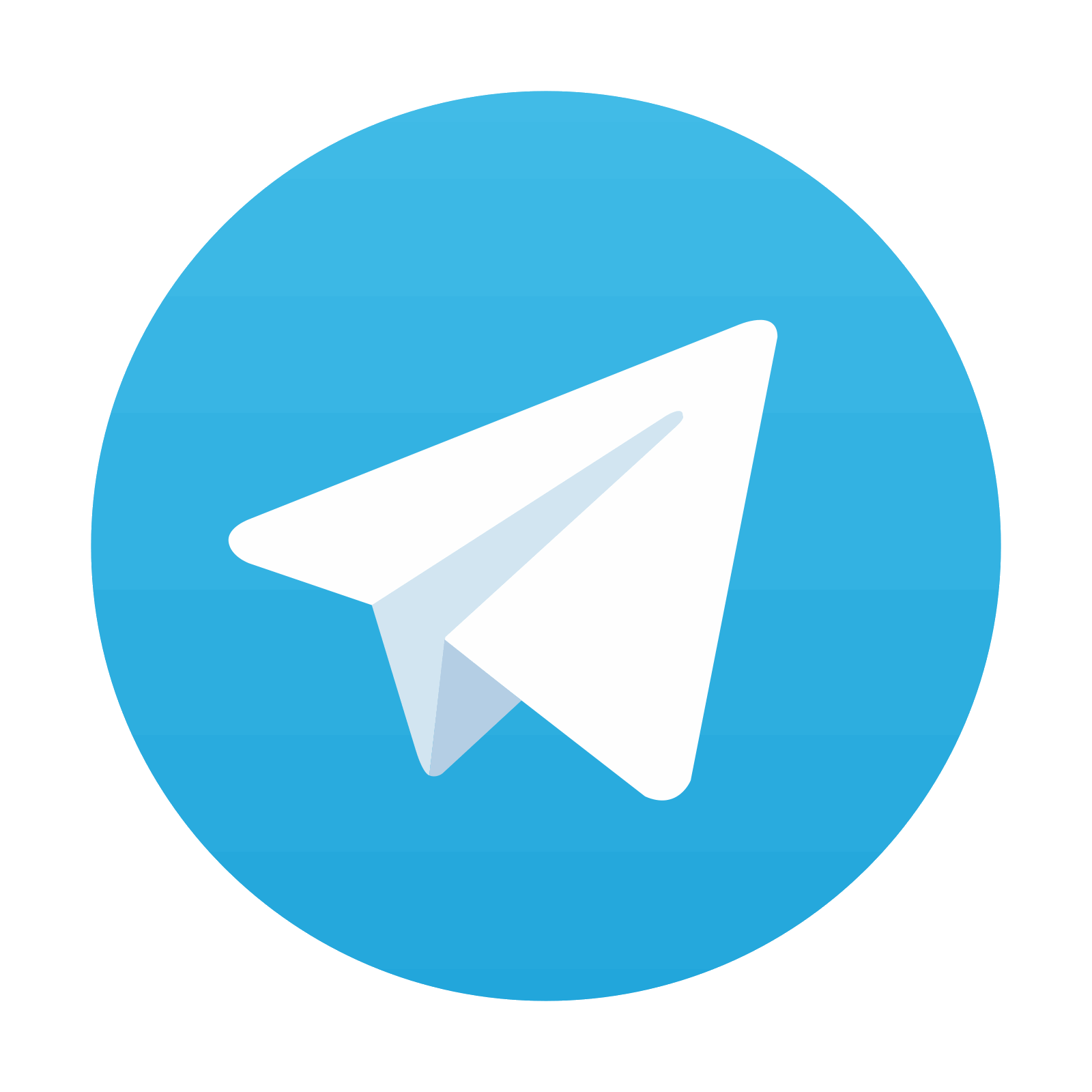
Stay updated, free articles. Join our Telegram channel
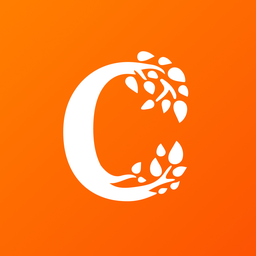
Full access? Get Clinical Tree
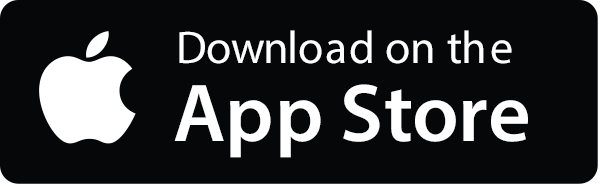
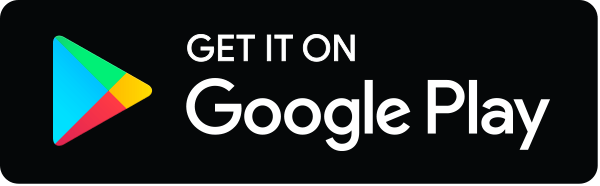