Perhaps nothing underscores the special relationship between the kidney and the eicosanoids better than the profound clinical effects non-steroidal anti-inflammatory drugs (NSAIDS) have on kidney function. NSAIDs are widely used to treat pain and inflammatory diseases, and work by blocking the enzymatic synthesis of prostaglandins, a type of eicosanoid, from arachidonic acid. However, chronic NSAID use is often complicated by major side effects, including renal sodium retention, resulting in edema, hypertension, and congestive heart failure. Conversely, in the sodium depleted state, NSAIDs can reduce renal blood flow, glomerular filtration rate, and cause acute renal failure. These observations underscore the critical role cyclooxygenase-derived arachidonic acid metabolites play in maintaining normal kidney function – particularly in the setting of physiological stress.
Perhaps nothing underscores the special relationship between the kidney and the eicosanoids better than the profound clinical effects non-steroidal anti-inflammatory drugs (NSAIDS) have on kidney function. NSAIDs are widely used to treat pain and inflammatory diseases, and work by blocking the enzymatic synthesis of prostaglandins, a type of eicosanoid, from arachidonic acid. However, chronic NSAID use is often complicated by major side effects, including renal sodium retention, resulting in edema, hypertension, and congestive heart failure. Conversely, in the sodium depleted state, NSAIDs can reduce renal blood flow, glomerular filtration rate, and cause acute renal failure. These observations underscore the critical role cyclooxygenase-derived arachidonic acid metabolites play in maintaining normal kidney function – particularly in the setting of physiological stress.
Cellular Origin of Eicosanoids
Eicosanoids are a family of biologically active, oxygenated metabolites derived from arachidonic acid (AA). AA is comprised of 20 carbon atoms configured as a polyunsaturated fatty acid chain with four double bonds (C20:4). Mammals lack the enzymatic machinery to synthesize AA de novo , instead it must be formed from dietary linoleic acid (C18:2) by addition of two carbons and further desaturation. Essential fatty acid (EFA) deficiency occurs in the absence of dietary linoleic and other fatty acid AA precursors, depleting the hormone-responsive pool of AA metabolite products. Of the approximate 10 gm of linoleic acid ingested per day, only about 1 mg/day is eliminated as end products of AA metabolism. Following its formation, AA is esterified into cell membrane phospholipids, principally at the 2 position of the phosphatidylinositol fraction (i.e., sn-2 esterified AA). This source comprises the major hormone-sensitive pool of AA that is susceptible to release by phospholipases.
Phospholipase-Mediated Arachidonic Acid Release
Multiple stimuli lead to release of membrane-phospholipid esterified AA via activation of cellular phospholipases, principally phospholipase A2s (PLA 2 ). This cleavage step is rate-limiting in the production of arachidonate metabolites. Activation of phospholipase C or PLD, on the other hand, releases AA via the sequential action of the phospholipase C-mediated production of diacylglycerol (DAG), with subsequent release of AA from DAG by DAG lipase. The physiological significance of AA release by these other phospholipases remains uncertain since, at least in the setting of inflammation, phospholipase A 2 action appears to be essential for the generation of biologically active AA metabolites. Cellular levels of free arachidonic acid available for eicosanoid production are primarily controlled by phospholipase A2 (PLA2). So far, more than 30 enzymes with PLA 2 activity have been identified, and have been classified into four groups: secretory PLA 2 (sPLA2); cytosolic PLA2 (cPLA2); calcium-independent PLA2 (iPLA2); and PAF acetylhydrolases (PAF-AH). The activity of cPLA2 is regulated by diverse cell membrane receptors, including the EGF receptor, and transmembrane guanine-nucleotide protein coupled (GPCRs) including adrenergic receptors, angiotensin II receptors, and purinergic receptors. These receptors activate guanine nucleotide-binding (G) proteins, leading to PLA 2 -mediated release of AA from membrane phospholipids. Alternatively, these receptors may activate cPLA 2 via mitogen-activated protein kinases (MAPK), protein kinase C (PKC), and Ca 2+ -calmodulin-dependent kinases.
Ambient physical conditions in the kidney including hypoxia, oxidative stress, and mechanical stretch can also activate PLA 2 activity. Dysregulated renal PLA 2 activity with attendant change in AA release results in altered substrate availability for the production of downstream metabolic products. This activation is believed to contribute to pathologic processes including acute kidney injury, diabetic nephropathy, and inflammatory glomerulonephritis. Some snake and bee venoms are imbued with high levels of secretory PLA 2 activity and, in part through this activity, can induce acute renal failure . A role for secretory PLA 2 in the pathogenesis of acute ischemic-reperfusion renal injury has also been supported by studies showing that sPLA 2 neutralizing antibodies protect rats from this form of injury.
Phospholipase A2 Receptors
Recently, an important role for a transmembrane cell surface secretory PLA 2 receptor (PLA 2 R) has been recognized in the pathogenesis of human idiopathic membranous nephropathy. Auto-antibodies to PLA 2 R are detected in ~70% of cases of human idiopathic membranous nephropathy. The antigen appears to be selectively expressed in podocytes ; however, the mechanism by which the auto-antibodies induce proteinuria and how these auto-antibodies arise remains to be determined. PLA 2 R is a type I transmembrane receptor and one of four mammalian members of the mannose-receptor family. PLA 2 R was initially identified as a binding protein for secreted phospholipase A 2 (PLA 2 ) that now has been expanded to a PLA 2 R family that exhibit different affinities for the secreted PLA 2 . New studies suggest these receptors could play additional transmembrane signaling roles, and may promote terminal cell differentiation and mitotic arrest.
Arachidonic Acid Metabolism
Following its release from membrane phospholipids, AA is usually rapidly re-esterified into the membrane or avidly bound by intracellular proteins, becoming unavailable for further metabolism. Should AA escape re-esterification and protein binding, it may be metabolized through one of three major enzymatic transformations, the common result of which is the incorporation of oxygen atoms at various sites of the fatty acid backbone, with accompanying changes in its molecular structure (such as ring formation). This results in the formation of biologically active molecules, collectively referred to as “eicosanoids.” The specific nature of the products generated is a function of the initial stimuli for AA release, as well as the metabolic enzymes available, as determined by the cell type involved.
Enyzmes capable of mediating AA metabolism through all three known pathways are present in the kidney, including cyclooxygenases 1 and 2, lipoxygenases, and cytochrome P450s ( Figure 17.1 ). Cyclooxygenase (COX, also called Prostaglandin H2 synthase or PGHS)-mediated AA metabolism comprises the first committed step in the formation of prostaglandins (PGs), prostacyclin, and thromboxane. The lipoxygenase pathway mediates the formation of mono-, di-, and trihydroxyeicosatetraenoic acids (HETEs), leukotrienes (LTs), and lipoxins (LXs), and the cytochrome P450-dependent oxygenation of AA mediates the formation of epoxyeicosatrienoic acids (EETs), their corresponding diols, HETEs, and monooxygenated AA derivatives. Fish oil diets, rich in n-3 polyunsaturated fatty acids ( n-3 fatty acids are those in which the double bond is three carbons from the terminal, i.e., n carbon, that is furthest from the carboxy-group atom, AA is thus an n-6 fatty acid) interfere with metabolism via all three pathways by competing with AA oxygenation, resulting in the formation of biologically inactive end-products. Interference with the production of pro-inflammatory lipids has been hypothesized to underlie the beneficial effects of fish-oil in IgA nephropathy, membranous nephropathy, and other cardiovascular diseases.

Cyclooxygenase Derived Prostanoids
Prostanoids, including the prostaglandins PGE 2 , PGF 2α , and PGD 2 , as well as the non-prostaglandin molecules thromboxane A 2 (TxA2) and prostacyclin (PGI 2 ), are derived from arachidonic acid via its di-oxygenation by cyclooxygenases 1 and 2 (COX1 and COX2). Cyclooxygenases exist as homodimers that are physically associated with, but do not pass through, the intracellular endoplasmic reticular membrane. Cyclooxygenases mediate a two-step reaction, initially converting free arachidonic acid to the unstable intermediate PGG 2 via a bis -oxygenase activity. PGG 2 is converted to PGH 2 via the peroxidase activity of COX. PGH 2 is subsequently metabolized to more stable primary biologically active prostanoids PGE 2 , PGF 2α , PGD 2 , PGI 2 , and TxA 2 by distinct enzymatic prostanoid synthases. These prostanoids exit the cell through uncharacterized mechanisms, where they exert paracrine or autocrine activity on specific and distinct cell surface G-protein coupled receptor(s). There is also less definitive evidence that prostanoids may provide physiologically relevant ligands for nuclear hormone receptors, including peroxisome proliferator activated receptors.
Two isoforms of COX have been identified, designated COX1 and COX2. Based on transcriptional elements in its 5′ upstream sequence, COX1 is believed to serve a constitutive housekeeping role, responsible for maintaining basic physiological function such as cytoprotection of the gastric mucosa, and control of platelet aggregation. Conversely, COX2 upstream promoter region has NF-κB, NFAT, and its expression is potently induced by inflammatory mediators and mitogens, consistent with its role in pathophysiologic processes including angiogenesis, inflammation, and tumorigenesis.
The major phenotype of COX2 knockout mice is renal dysgenesis, underscoring the special role COX2 plays in the kidney. This defect is characterized by a structurally normal medulla, but hypotrophic renal cortical development with small glomerular size, due to a defect occurring relatively late in partuition. The mechanism is undetermined, but may be related to the particular expression pattern of COX2 in the kidney since normally it is focally expressed adjacent to the glomerulus in the macula densa and the surrounding thick ascending limb cells. As in other organs, the housekeeping gene is COX1, which is also constitutively expressed at high levels in the kidney but in cellular compartments distinct from COX2, especially in the collecting duct and glomerular parietal epithelium. Low levels of COX1 are also detected in medullary interstitial cells, but these cells are also uniquely characterized by high endogenous levels of COX2.
Clinical pharmacologic studies are also consistent with a critical role of COX2 for maintaining cardiovascular homeostasis and normal renal function. Indeed, most of the clinically observed side effects associated with the use of non-selective NSAIDs, including edema, hypertension, increased congestive heart failure, hyperkalemia, and acute renal failure, have also been observed with COX2 selective inhibitors. COX2-dependent PGE 2 production is inversely related to luminal chloride concentration delivered to the macula densa, so that in volume depleted states high PGE 2 production rates may exert a vasodilator effect on the afferent arteriole, contributing to maintenance of glomerular blood flow. Impairment of renal function is presumed due to loss of specific prostanoids, derived from the metabolism of the common cyclooxygenase product PGH 2 .
Prostanoid Function
Once formed, the COX-derived arachidonate metabolite PGH 2 is further metabolized by prostanoid synthases into at least five primary biologically active prostanoids. Prostanoid synthases include PGE 2 synthase (PGES), prostacyclin synthase (PGIS), PGD synthase (PGDS), PGF synthase (PGFS), and thromboxane synthase, responsible for PGE2, PGI2, PGD2, PGF2α, and TxA2 biosynthesis respectively.
Most prostanoids are short lived, being highly susceptible to enzymatic inactivation, thereby limiting their effect to the immediate vicinity of their synthesis. The paracrine and autocrine biologic effects of COX-derived prostanoids are diverse and complex, depending on which prostanoid is produced and which receptor is available. Thus, the effects of prostanoids on kidney function rely on distinct enzymatic machinery that couples phospholipase and COX to specific prostanoid synthase in specific cells, yielding a specific prostanoid which acts locally through specific G-protein coupled receptors, exerting its particular effect.
At steady-state PGE 2 is the most abundant prostanoid in the mouse kidney, followed by PGI 2 , PGF 2α , and TxA 2 . Under basal conditions, both COX1 and COX2 pathways are responsible for the biosynthesis of these prostanoids. Similarly, PGE2 is the most abundant prostanoid in human urine, and under basal, non-stressed conditions is produced by both COX1 and COX. In contrast, COX2 primarily contributes to angiotensin II-induced PGE 2 and PGI2 generation in the kidney, and under conditions of low-sodium diet in humans. The intrarenal cellular sites where COX1 and COX2 prostanoids are synthesized remain to be fully defined.
Following their synthesis, these prostanoids become available to exert their biological effects via a diverse family of membrane spanning G-protein coupled prostanoid receptors. These include the DP, EP, FP, IP, and TP receptors, each of which is selectively activated by a specific ligand – PGD 2 , PGE 2 , PGF 2α , PGI 2 or TXA 2 , respectively. PGE2 receptors, designated EP receptors, are unique in that they are encoded by four distinct genes encoding the proteins for EP1, EP2, EP3, and EP4 receptors. Each prostanoid receptor activates a distinct G-protein coupled signaling pathway. The IP, DP1, EP2, and EP4 receptors are coupled to the stimulatory G-protein (Gs) and signal by increasing intracellular cAMP levels, whereas the TP, FP, and EP1 receptors induce calcium mobilization. The FP, DP2, and EP3 receptors can couple to an inhibitory G-protein (Gi) and reduce cAMP synthesis.
Restricted cellular expression of prostanoid receptors provides an important mechanism by which a COX-derived prostanoid can exert differential actions in physiological and pathophysiological processes. In the kidney the EP receptors map to distinct segments of the nephron. Similarly, all four EP receptors have been described in major inflammatory cells including T-lymphocytes, B-lymphocytes, macrophage, and mast cells ; however, whether these receptors are simultaneously expressed in individual cells is uncertain. It has been proposed that activation of different receptors on different cells at different stages of inflammation may account for the pro- or anti-inflammatory action of PGE 2 .
Prostaglandin E2
PGE 2 is synthesized by at least three forms of PGE synthases, including microsomal PGE synthase 1 (mPGES1), microsomal PGE synthase 2 (mPGES2), and cytosolic PGE synthase (cPGES1). The two membrane associated PGE 2 synthases are 33 kDa and 16 kDa enzymes designated mPGES1 and mPGES2, respectively. Microsomal PGES1 displays a higher catalytic activity relative to other PGE synthases and, like COX2, its expression can be induced by cytokines and inflammatory stimuli. In contrast, the expression of cPGES and mPGES2 do not seem to be inducible and may play housekeeping functions.
Genetic disruption confirms that mPGES1 −/− mice exhibit a marked reduction in inflammatory responses compared with mPGES1 +/+ mice, and indicates that mPGES1 is critical for the induction of inflammatory fever. It has been proposed that mPGES1 couples primarily to the inducible COX2 in inflammatory cells. In contrast, intrarenal expression of mPGES1 maps to cells of the collecting duct that primarily express COX1 with lower expression in medullary interstitial cells and macula densa that express COX2 ( Figure 17.2 ). Thus, in the kidney mPGE1 co-localizes with both cyclooxygenase 1 and 2. The renal phenotype of the mPGE1 knockout mouse is relatively subtle, and is characterized by increased blood pressure sensitivity to high-sodium diet and mineralocorticoids, as well as increased vascular reactivity to angiotensin-II, although not all investigators have seen these effects. These results are consistent with a role for mPGES1-derived PGE 2 in buffering physiologic stresses that tend to increase blood pressure. Notably, the kidneys of mPGES1 −/− mice are normal and do not exhibit the renal dysgenesis observed in COX2 −/− mice. Nor do these mice exhibit perinatal death from patent ductus arteriosus observed with the prostaglandin EP4 receptor knockout mouse, suggesting other sources of PGE2 production are sufficient to provide adequate receptor activation. These sources could include cPGES and mPGES2. Both cPGES and mPGES2 are expressed in the kidney ; however, their intrarenal role(s) have not yet been elucidated. In addition, several cytosolic glutathione-S-transferases have the capacity to convert PGH 2 to PGE 2 ; however, their physiologic role in this process remains uncertain.

E-Prostanoid Receptors
All four E-Prostanoid receptors (EP receptors) are expressed in the kidney ( Figure 17.3 ). Each exhibits a distinct mRNA expression profile along the nephron. The EP4 receptor predominates in the glomerulus, while the EP3 and EP1 receptors are primarily detected in the thick limb and collecting duct. The EP2 receptor is expressed at lower levels in the renal vaculature and stroma. Each receptor plays a distinct role in these regions, mediating many of the well-defined physiologic actions of PGE 2 that have been identified over the past several decades.

EP 1 Receptor
The EP1 receptor was originally identified pharmacologically via its smooth muscle constrictor activity in guinea pig ileum, and its unique profile of response to a series of prostanoid analogs. The EP1 receptor cDNA has been cloned from numerous species, including human, dog, mouse, rat, and rabbit. The human EP1 receptor cDNA encodes a 402 amino acid polypeptide with a predicted molecular mass of 41,858 kDa. This receptor signals via a mechanism linked to increased cell Ca + , and is accompanied by modest increases in IP 3 generation.
Studies of EP1 receptors have taken advantage of several relatively selective antagonists that block their activation, including SC-19220, SC-53122, and ONO-8130. A significant impetus behind the development of clinically active EP1 receptor antagonists derives from evidence that the EP 1 receptor plays an important role in prostaglandin-mediated pain, and that EP1 receptor antagonists have EP1 properties. These antagonists provide useful tools to study EP 1 receptor physiology in vivo .
The EP1 receptor is highly expressed in the kidney, where it primarily localizes to the collecting duct with an increasing mRNA expression gradient from the cortical to the medullary collecting duct. In the collecting duct, activation of the EP1 receptor inhibits Na + and water reabsorption via a Ca 2+ -coupled mechanism. These results suggest that renal EP 1 receptor activation contributes to PGE 2 -dependent natriuresis by inhibiting Na + transport in the collecting duct. Despite this in vitro demonstration, these natriuretic effects have been difficult to demonstrate in vivo .
Genetic disruption of the EP1 receptor does not lead to a significant impairment of sodium excretion; however, EP1 knockout mice do exhibit increased renin and aldosterone levels, consistent with maintenance of normotension at the expense of activation of the renin–angiotensin system. EP1 receptor knockout mice not only exhibit reduced blood pressure on normal chow, but also impaired pressor response to angiotensin II. These studies identified EP1 mRNA expression in small resistance vessels of mice including the afferent arterioles of the glomerulus, and are consistent with more recent studies suggesting Ang II-stimulated vasoconstriction may in part be mediated by activation of vascular EP1 receptors. EP1 receptors have also been identified in glomerular mesangial cells, where they may contribute to mesangial contraction. Inhibition of the EP1 receptor slows the progression of mesangial expansion in experimental models of diabetic nephropathy. EP1 receptor knockout mice are resistant to the pressor effects of angiotensin II, and EP1 receptor antagonists can also block the Ang II pressor activity. It is instructive to consider the role of PGE 2 as a vasoconstrictor through its actions on the EP1 receptor, as opposed to its classically characterized role as a vasodilator/vasodepressor. This underscores the capacity of PGE 2 to serve as a physiological buffer of blood pressure, either in support or reduction of blood pressure (see below).
EP 2 Receptors
In contrast to the smooth muscle constrictor activity of the EP1 receptor, the EP2 receptor was originally defined by its relaxant activity in smooth muscle. The human EP 2 receptor cDNA encodes a 358 amino acid polypeptide, which signals through increased cAMP and is selectively activated by butaprost. The EP 2 receptor may be distinguished from the EP 4 receptor, the other major cAMP stimulating and vasorelaxant EP receptor, by its selective activation by butaprost and relative insensitivity to the EP 4 agonist PGE 1 -OH. Literature prior to 1995 may be confusing regarding the EP 2 receptor, because before the human EP 2 receptor was cloned, the previously cloned EP 4 receptor was classified as the EP 2 receptor.
The physiological processes mediated by the EP2 receptor include important roles in reproduction and blood pressure regulation. The precise tissue distribution of the EP 2 receptor has only been characterized by Northern blot analysis of mRNA distribution. This reveals a major mRNA species of ~3.1 kb that is most abundant in the uterus, lung, and spleen, exhibiting only low levels of expression in the kidney. EP2 knockout mice exhibit a fertility defect and the development of hypertension on a high NaCl diet (these latter effects are significantly influenced by the genetic background of the mouse strain). In the kidney, despite incomplete histological characterization, a preponderance of functional and mRNA expression evidence suggests the EP2 receptor is expressed in stromal cells of the kidney, including renal medullary interstitial cells, vascular pericytes along the vasa recta, and glomerular arterioles where it contributes to afferent arteriolar dilation ( Figure 17.4 ). Evidence suggests that deletion of the EP2 receptor in renal medullary interstitial cells, combined with the absence of its systemic vasodilator activity, contributes to salt-sensitive hypertension in the EP2 knockout mouse.

EP 3 Receptor
In smooth muscle the EP 3 receptor generally acts as a constrictor. This receptor is unique, in that at least seven alternatively spliced variants defined by unique COOH-terminal cytoplasmic tails exist in humans alone, and over 22 unique variants have been observed in rats, rabbits, mice, cows, and humans. These splice variants encode proteins of a predicted molecular mass between 40 and 45 kDa. All the EP 3 splice variants bind PGE 2 and the EP 3 specific prostanoid analogs with similar affinity, and inhibit cAMP generation via a pertussis toxin-sensitive G i -coupled mechanism; however, additional signaling mechanisms may be differentially activated by the different COOH-terminal tails.
Mice with targeted deletion of the EP 3 receptor exhibit an impaired febrile response to lipopolysaccaride and PGE 2 , suggesting that the EP 3 receptor antagonists could be effective antipyretic agents. In the kidney the EP 3 receptor is highly expressed in the cortical and outer medullary collecting duct, where it antagonizes vasopressin-stimulated water absorption via pertussis toxin-sensitive inhibition of cAMP generation. Despite relatively high levels of EP 3 receptor in the kidney collecting duct, mice with targeted disruption of this receptor only display a subtle alteration with altered urinary concentrating ability in mice treated with NSAIDs. These findings raise the possibility that prostaglandin receptors other than the EP 3 receptor exert overlapping effects that also (such as the EP1 and FP receptor) modulate the urinary concentration and dilution by this segment of the nephron.
EP 4 Receptor
The EP 4 receptor can be distinguished from the EP 1 and EP 3 receptors by its insensitivity to sulprostone, and from EP 2 receptors by its insensitivity to butaprost and relatively selective activation by PGE 1 -OH. The human EP 4 receptor cDNA encodes a 488 amino acid polypeptide with a predicted molecular mass of ~53 kDa. Like the EP 2 receptor, EP 4 signals through increased cAMP, but may also engage other signaling mechanisms including PI3K activation. EP 4 receptor mRNA is relatively highly expressed compared with the EP 2 receptor and widely distributed, with a major species of ~3.8 kb detected by Northern analysis in thymus, ileum, lung, spleen, adrenal gland, and kidney. In contrast to other EP receptor knockouts, EP4 receptor knockout mice exhibit a profound perinatal lethal phenotype due to impaired closure of the ductus arteriosus, consistent with its robust expression in this vessel.
In the kidney, EP4 receptor mRNA is predominantly expressed in the glomerulus where it modulates glomerular hemodynamics through opposing direct vasodilator activity, and an indirect vasoconstrictor activity via stimulation of renin release ( Figure 17.4 ). The ability of PGE 2 to increase renin release is well-established and of clinical relevance, since NSAID blockade of prostaglandin synthesis can be associated with hyporeninemic hypoaldosteronism, and COX2 inhibitors can block the hyper-reninemia associated with volume depletion or Bartter’s syndrome. Accumulating evidence supports a role for COX2-mediated PGE 2 production, and subsequent activation of juxtaglomerular EP4 receptor in mediating renin release. As mPGES-specific inhibitors and EP4 receptor antagonists become increasingly available it will be important to determine the relative contribution of PGE2-dependent renin release versus prostacyclin-dependent effects (see below).
In volume depletion, congestive heart failure, and shock, intrarenal PGE 2 production helps maintain glomerular perfusion via afferent arteriolar vasodilator EP2 receptors ( Figure 17.4 ), while simultaneously maintaining systemic blood pressure by stimulating renin release through EP4 receptors. Genetic deletion of the EP4 receptor or EP4 inhibitors impairs renin release in mice following furosemide-induced volume depletion. Inhibition of PGE 2 synthesis using NSAIDs or COX2 inhibition in these settings can actually drop blood pressure by inhibiting renin release, and lead to acute renal failure due to decreased glomerular perfusion.
The EP4 receptor is also abundant in glomerular podocytes. In podocytes EP4 receptor activation may impair their ability to withstand mechanical stress, since podocyte selective overexpression of EP4 receptors accelerates renal injury in a mouse renal ablation model of kidney disease.
Other roles for the EP4 receptor in controlling blood pressure have been suggested, including the ability to stimulate aldosterone release from zona glomerulosa cells. It remains to be determined whether the adrenal EP4 receptor plays any role in hyperkalemia and hyporeninemic hypoaldosteronism associated with NSAID blockade of prostaglandin synthesis or whether this is primarily due to hyporeninemia. Important vasodilator effects of EP 4 receptor activation in venous and arterial beds have been described. Roles for EP4 receptors in immune cell activation and osteoblast function have also been reported.
Prostaglandin F Synthesis
PGF 2α may derive either directly from PGH 2 via a PGF synthase or via a NADPH-dependent 9 ketoreductase, which converts PGE 2 into PGF 2α . This enzymatic activity is typically cytosolic, and may be detected in homogenates from renal cortex, medulla or papilla. Another more obscure pathway for PGF formation is by the action of a PGD 2 ketoreductase, yielding a stereoisomer of PGF 2α , 9α,11ß-PGF 2 (11epi-PGF 2α ). This reaction, and conversion of PGD 2 into the biologically active metabolite (9α,11β-PGF 2α ) has been documented in vivo . This PGF 2α isomer can also ligate and activate the FP receptor. The physiologically relevant enzymes responsible for PGF 2α formation in the kidney remain incompletely characterized.
Prostaglandin 9-Ketoreductase (PG9KR) and PGF 2α Synthesis
Prostaglandin F synthase activity may be mediated via several distinct, and incompletely defined, enzymes. One major synthetic pathway appears to occur via a member of the aldo-ketoreductase 1C family. Renal PGE 2 9-ketoreductase also exhibits 20α-hydroxyl-steroid reductase activity that may be involved in steroid metabolism. PG9KR activity appears to be particularly robust in suspensions from the thick ascending limb of Henle (TALH).
Interestingly, some studies suggest activity of a 9-ketoreductase may be modulated by salt intake and the angiotensin AT2 receptor. This activity may play a role in the development of salt-sensitive hypertension. AT2 receptor knockout mice exhibit salt-sensitive hypertension associated with increased PGE 2 production and reduced production of PGF 2α , consistent with decreased 9-ketoreductase activity. Other studies suggest increased dietary potassium intake may also enhance the activity of conversion from PGE 2 to PGF 2α . The intrarenal sites of expression of this enzymatic activity remain to be characterized.
F-Prostanoid Receptors
Once formed, PGF 2α is available to interact with the intrarenal FP receptors. The human FP receptor mRNA is predicted to encode 359 amino acid residues with a molecular mass of ~40 kDa. In fibroblasts and smooth muscle the FP receptor signals through increased cellular calcium, and its activation is associated with muscle contraction. The FP receptor is highly expressed in the ovarian corpus luteum, and mice lacking the FP receptor exhibit a major reproductive defect due to failure of partuition because of impaired reduction of progesterone at term. FP receptor antagonists have been developed, and their use proposed as a means of delaying pre-term delivery. The FP receptor is highly expressed in the ocular ciliary body and FP selective agonists including travaprost, latanoprost, and bimatoprost are in use for clinical treatment of glaucoma d. In the kidney, the FP receptor is highly expressed in the distal convoluted tubule, connecting tubule, and cortical collecting duct, where it inhibits vasopressin-stimulated renal water transport consistent with recent studies that the FP receptor knockout mice exhibit mild polyuria and polydipsia. Interestingly, in the rabbit cortical collecting duct epithelium the FP receptor appears to signal through a pertussis toxin-sensitive G i -coupled mechanism, rather than the classical Ca 2+ -coupled signaling mechanism observed in smooth muscle cells. Vascular expression of FP receptor in pre-glomerular arterioles and other resistance vessels has also been demonstrated, and studies in FP receptor knockout mice show they are relatively hypotensive. These studies also provide evidence that renal JGA FP receptor activation directly stimulates renin release.
Prostaglandin D 2 is derived from PGH 2 via the action of specific enzymes designated PGD synthases. Two major enzymes are capable of transforming PGH 2 to PGD 2 – a lipocalin type PGD synthase and a hematopoietic type PGDS. RT-PCR showed that L-PGDS is strongly expressed in kidney cortex and outer medulla, including in nearly all segments of the nephron, while H-PGDS mRNA is only detected in microdissected outer medullary collecting duct.
Lipocalin PGD synthase (L-PGDS) is a multifunctional molecule, and on addition to prostaglandin H2 it binds a variety of small lipophilic molecules including bilirubin and biliverdin. Mice lacking the lipocalin D synthase gene exhibit pain sensation. LPGDS-mediated PGD2 synthesis also appears to play an important role in the sleep/wake cycle, but its role in renal PGD2 synthesis has not been studied. Urinary levels of L-PGDS, also known as beta-trace protein, have been increasingly studied as a biomarker of acute and chronic renal injury. LPDGS knockout mice appear to be more susceptible to diabetic nephropathy.
PGD 2 is the major prostanoid released from mast cells following challenge with IgE, and this synthesis appears to be mediated by the hematopoietic form of PGDS. The precise role of hematopoietic PGDS in the kidney remains uncertain, as does the significance of its reported localization in the outer medullary collecting duct.
D-Prostanoid Receptors
Once synthesized, PGD 2 is available to interact with either the DP1 or DP2 (originally identified as CRTH2) receptors or undergo further metabolism to a PGF 2 -like compound that can interact with the FP receptor (see above). The human DP1 receptor is a cAMP-coupled GPCR with a predicted molecular mass similar to other prostanoid receptors (~40 kDa). It exhibits a relatively selective tissue distribution, with particularly high expression in retina and small intestine, where it appears to be highly expressed in mucus secreting goblet cells. The DP2 receptor is a GPCR that is also activated by PGD2, but it is unrelated in sequence to the classic prostanoid receptor family, being more closely related to members of the N -formyl peptide receptor (FPR) subfamily. DP2 is selectively expressed in Th2 cells, cytotoxic T-cells, eosinophils, and basophils. DP and DP2 receptors are intimately involved in the immune allergic response. Neither DP1 receptors nor DP2 receptors appear to be highly expressed in the kidney, and while infusion of a DP1 selective agonist lowers blood pressure these effects appear to be primarily due to peripheral vasodilation and not by directly affecting renal blood flow.
Prostacyclin (PGI 2 )
The biological effects of prostacyclin are numerous and include nocioception, anti-thrombosis, and vasodilator actions, which have been targeted therapeutically to treat pulmonary hypertension. Prostacyclin (PGI 2 ) is derived from the enzymatic conversion of PGH 2 via prostacyclin synthase (PGIS) to PGI 2 . The PGIS cDNA is comprised of a 1500 bp open reading frame that encodes a 500 amino acid protein of approximately 56 kDa. Northern blot analysis shows prostacyclin synthase mRNA is widely expressed in human tissues and is particularly abundant in ovary, heart, skeletal muscle, lung, and prostate. PGI synthase expression exhibits segmental expression in the kidney, especially in kidney inner medulla tubules and interstitial cells.
PGI 2 synthase-null mice exhibit a profound renal phenotype with nephrosclerosis and areas of renal infarction. PGI 2 levels in the plasma, kidneys, and lungs were reduced, documenting the role of this enzyme as an in vivo source of PGI 2 . Blood pressure and blood urea nitrogen and creatinine in the PGIS knockout mice were significantly increased, and renal pathological findings included surface irregularity, fibrosis, cysts, arterial sclerosis, and hypertrophy of vessel walls. Thickening of the thoracic aortic media and adventitia were observed in aged PGIS-null mice. Interestingly, this is a phenotype different from that reported for the IP receptor knockout mouse, which failed to exhibit abnormal kidney morphology. These differences suggests the presence of additional IP receptor independent PGI 2 activated signaling pathways – possibly through nuclear receptors such as PPARdelta. Regardless, these findings demonstrate the importance of PGI 2 in the maintenance of blood vessels and to the kidney.
IP Receptor
The IP receptor mRNA is highly expressed in the afferent arteriole, where it may combine with the effects of EP2 and EP4 receptors to dilate renal arterioles and stimulate renin release. IP receptor deficient mice exhibit reduced renin and are resistant to the development of hypertension following unilateral renal artery stenosis, consistent with an impaired IP receptor-mediated renin release. COX2-derived prostacyclin from the endothelium also appears to serve an atheroprotective role in mice, and its loss could contribute to the cardiovascular risks associated with the use of COX2 inhibitors. Conversely, thromboxane receptors may counteract the effects of these protective effects of prostacyclin and accelerate atherosclerotic lesions, as well as increasing vascular resistance.
Thromboxane A2
Thromboxane A 2 (TxA 2 ) is produced from PGH 2 by thromboxane synthase (TxAS), a microsomal protein of 533 amino acids with a predicted molecular weight of ~60 kDa. The human gene is located on chromosome 7q, and the enzyme exhibits homology to the cytochrome P450s and is now classified as CYP5A1. TxAS mRNA is highly expressed in hematopoietic cells, including platelets, macrophages, and leukocytes, as well as thymus, kidney, lung, spleen, prostate, and placenta. Immunolocalization of TxA synthase demonstrates high expression in the dendritic cells of the interstitium, with lower expression in glomerular podocytes of human kidney. In the kidney, thromboxane synthase is mainly detected in the glomeruli. TxA 2 synthase expression is regulated by dietary salt intake. Furthermore, experimental use of ridogrel, a specific thromboxane synthase inhibitor, reduced blood pressure in spontaneously hypertensive rats. The clinical use of TxA 2 synthase inhibitors is complicated by the fact that its endoperoxide precursors (PGG 2 /PGH 2 ) are also capable of activating its downstream target, the TP receptor, so thromboxane receptor antagonists would be expected to more definitively interfere with this pathway.
TP Receptor
The thromboxane receptor (TP) was the first member of the prostanoid receptor family cloned. This GPCR has a predicted molecular mass ~37.5 kDa, and is highly expressed in platelets and vascular tissue where its activation increases intracellular calcium. In the kidney TP receptor mRNA is predominantly expressed in the glomerulus and vascular tissue, where it contributes to vasoconstrictor activity following Ang II infusion and following renal injury.
Prostaglandin Transport
One of the major areas of uncertainty in eicosanoid research is precisely how prostanoids, synthesized inside the cell, transit the cell membrane to become available to interact with cell surface receptors. Recent studies have identified several transmembrane proteins capable of facilitated transport of prostaglandins across cell membranes. Schuster’s group identified a lactate/PGE 2 exchanger they designated prostaglandin transporter or PGT. The importance of PGT in mediating the in vivo effects of PGE 2 has been substantially bolstered by studies showing genetic disruption of the PGT gene is associated with perinatal death due to persistence of a patent ductus arteriosus recapitulating the phenotype of the EP4 receptor knockout. Although this prostaglandin transporter appears poised primarily to provide a PGE2 re-uptake mechanism, it is notable that the transporter is expressed in renal cells that are major sites of prostaglandin synthesis, rather than those renal cells involved in PGE 2 inactivation. These findings are consistent with the possibility that PGT plays an important role in directing vectorial PGE 2 release. Nevertheless, it seems unlikely that this protein comprises the pathway mediating PGE 2 release. More recent evidence supports a role for the multi-drug resistance protein 4 (MRP4) as a potential PGE 2 efflux mechanism. MRP4 knockout mice exhibit low levels of circulating PGE 2 and reduced inflammatory pain, consistent with a role in PGE2 extrusion.
Prostaglandins and the Pathogenesis of Kidney Disease
Renal Inflammation
Increased glomerular COX1 or COX2 expression has been reported in patients with nephritis, and in animal models of nephritis. Glomerular expression of COX2 is upregulated in patients with active lupus nephritis and in lupus nephritis animal models. COX2 inhibition has shown beneficial effects on passive Heymann nephritis (PHN), a model of membranous nephropathy. Cell culture studies show that thromboxane A2 contributes to complement-induced cytotoxicity of glomerular epithelial cells. In contrast, in anti-Thy1.1 glomerulonephritis model, an animal model of mesangioproliferative glomerulonephritis (MPGN) that is characterized by endothelial injury, COX2 inhibition is associated with increased mesangiolysis, albuminuria, and delayed recovery from glomerular injury. Further studies suggest that healing of injured glomerular capillary endothelium in this model may depend on COX2-derived prostanoids, and COX2 inhibition may lead to impaired capillary endothelium healing. The role of COX-derived prostanoids in autoimmune and inflammatory disease has been well-documented. COX2 expression and prostanoid biosynthesis can be induced in macrophages and dendritic cells by inflammatory agents, such as LPS, IL-1ß, and interferon. PGDS expression or activity has been described in dendritic cells, macrophage, eosinophil, neutrophil, and mast cells. Prostanoids, particularly PGE2 and PGI2, have been shown to enhance inflammatory reactions. Conversely prostanoids also exert anti-inflammatory effects. The pro- or anti-inflammatory effect of prostanoids is dependent on specific prostanoid, receptor subtype, cell population, and context of activation. Additional studies are required to define the precise roles of prostanoids in different forms of glomerulonephritis.
Renal COX-Derived Prostanoids and Diabetic Nephropathy
Diabetic nephropathy is characterized by microalbuminuria, glomerular hypertrophy, mesangial expansion with glomerular basement membrane thickening, arteriolar hyalinosis, and global glomerular sclerosis, which ultimately lead to the progression to proteinuria and renal failure. Supra-normal GFR (hyperfiltration) typifies the early stages of diabetic nephropathy. Studies in diabetic humans and streptozotocin (STZ)-induced type I diabetic rats show increased renal PGE2, PGI2, and TxB2 levels. COX2 expression is increased in the thick ascending limb and macula densa in diabetic humans and rodents. Selective COX2 inhibition reduces glomerular hyperfiltration in streptozotocin-induced diabetic rats, consistent with COX2-derived prostanoids increasing renal blood flow in the diabetic kidney. The identity of the specific COX2-derived prostanoids and their cognate receptors involved in pathogenesis of diabetic hyperfiltration has not been completely characterized. EP1 receptor antagonist treatment ameliorates renal and glomerular hypertrophy, and decreases mesangial expansion. A thromboxane receptor (TP) antagonist has also been reported to attenuate proteinuria and ameliorate histological changes of diabetic nephropathy in diabetic apolipoprotein E-deficient mice. It has not been determined whether similar beneficial effects might be observed in human diabetic nephropathy.
Prostaglandins and Progression of Kidney Disease
COX-derived prostanoids may also modify renal function and glomerular damage in non-diabetic chronic renal disease. After subtotal renal ablation, renal cortical COX2 expression is increased, predominantly in the macula densa and surrounding cTAL. Selective COX2 inhibition decreases proteinuria and inhibits the development of glomerular sclerosis. These studies are consistent with a role of COX2-derived prostanoids in the pathogenesis of structural and functional deterioration of kidney in chronic kidney disease.
Lipoxygenase Derived Eicosanoids: 5-, 12-, and 15-HETEs and Leukotrienes
Arachidonic acid may be metabolized to form leukotrienes and hydroxyeicosatetraenoic acids (HETEs) by a family of enzymes designated lipoxygenases ( Figure 17.5 ). Leukotriene arachidonic acid metabolites play a major role in inflammatory disease, especially asthma and other inflammatory pulmonary diseases where cysteinyl leukotriene receptor antagonists have found a place as a standard of care. In contrast to the prostaglandins, it has been difficult to demonstrate a major role for leukotrienes in the normal kidney.

Lipoxygenases (LOX) are comprised of non-heme iron containing enzymes that insert molecular oxygen into polyunsaturated fatty acids such as arachidonic acid and linoleic acid. Six functional human lipoxygenases have been cloned: 5-lipoxygenase (gene name: ALOX5); platelet-type 12-lipoxygenase (gene name: ALOX12); 12/15-lipoxygenase (leukocyte-type 12-LO for mice, 15-LO type 1 for human, gene name: ALOX15); epidermal-type 12-lipoxgenase (gene name: ALOXE3); 12(R)-lipoxygenase (gene name: ALOX12B); and 15-lipoxygenase type 2 (gene name: ALOX15B, 8-lipoxygenase in mice).
5-Lipoxygenase is the key enzyme in leukotriene biosynthesis. In the presence of 5-LO-activating protein (FLAP) 5-lipoxygenase catalyzes the generation of leukotriene A4 (LTA4). LTA4, in turn, is converted by LTA4 hydrolase to LTB4, capable of activating LTB4 receptors. LTB4 is a potent chemotactic substance, and increases polymorphonuclear leukocytes (PMN) aggregation and adhesion to the endothelium. Alternatively, LTA4 can be converted to cysteinyl (cys) leukotrienes (LTC4, LTD4, and LTE4) through leukotriene C4 synthase. LTC4 and LTD4 contract vascular smooth muscle cells and increase vascular permeability. These leukotrienes are usually released locally by leukocytes. Mice with 5-LO gene disruption exhibit a reduced inflammatory reaction, supporting the pro-inflammatory action of 5-LO-derived metabolites. Recently, increased expression of leukotriene C4 synthase and formation of cysteinyl-leukotrienes have been reported in human abdominal aortic aneurysm.
As might be predicted from its expression in inflammatory cells, 5-LO-derived products appear to play an important role in glomerular immune injury. 5-LO mRNA and 5-LOX-activating protein (FLAP) mRNA are detected in the glomeruli and vasa recta. Both leukotriene receptor B4 and the cysteinyl leukotriene receptor type 1 are selectively expressed in the glomerulus, suggesting 5-LO products are involved in glomerular function. Glomerular synthesis of LTB4 and LTC4/LTD4 is markedly enhanced in both human disease and experimental glomerular immune injury. LTD4 may contribute to the reduction of GFR in the acute phase of injury by virtue of its potent vasoconstrictor action and contraction of mesangial cells. LTD4 may also increase intraglomerular pressure, contributing to proteinuria. LTB4, a potent promoter of PMN attraction, participates in glomerular damage by amplifying PMN-dependent mechanisms of injury.
The biological functions of the other members of the lipoxygenase family are more obscure. The 12-LO, catalyzes the formation of oxidized lipids 12(S)-hydroxyeicosatetraenoic acid [12(S)-HETE]. Human 15-LO type 1 shares high homology with rodent leukocyte-type 12-LO; both can mediate the formation of 12(S)-HETE and 15(S)-HETE from arachidonic acid, and are thus classified as 12/15-LO. Some evidence suggests that 12(S)-HETE and 15(S)-HETE play important roles in systemic homeostasis and renal–cardiovascular pathology ; however, specific receptors for these products have not yet been identified. A recent study implicates ALOX15 as playing a role in bone mineralization. 12/15-Lipoxygenase products also appear to be involved in the pathogenesis of atherosclerosis.
12/15-Lipoxygenase-derived products may contribute to the pathogenesis of diabetic complications, including diabetic nephropathy. 12/15-LO is detected in renal microvessels, glomeruli, and mesangial cells. 12/15-LO levels are increased in the glomeruli of experimental diabetic animals. The 12/15 LO pathway has been shown to be a critical mediator of TGFβ and angiotensin II (ANG II)-induced mesangial cell hypertrophy and extracellular matrix accumulation. These studies also suggest that ANG II-induced mesangial cell hypertrophy and extracellular matrix synthesis in cultured rat mesangial cells can be blocked by an LO inhibitor or targeted 12/15 LO gene deletion.
Cytochrome P450 Monooxygenase-Derived Eicosanoids: 20-HETE and EETs
Free arachidonic acid can also be metabolized by the cytochrome P450 monooxygenases (CYP450) to produce hydroxy- and epoxy-arachidonic acid derivatives. The major CYP450-catalyzed reactions in most tissues are mediated by epoxygenase and ω-hydroxylase activities of the CYP450 family, which are responsible for biosynthesis of epoxyeicosatrienoic acids (EETs) and 20-hydroxyeicosatetraenoic acid (20-HETE), respectively ( Figure 17.6 ). These metabolites have been shown to possess biological activity, but the mechanisms mediating these biological effects remain obscure since specific receptors have not been identified.

Members of the P450 CYP2C , and 2J subfamilies have been identified as functionally relevant epoxygenases, while members of 4A and 4F are ω-hydroxylases, respectively. 20-HETE is a potent vasoconstrictor. EETs are produced in the vascular endothelium, and are potent vasodilators. EETs are also produced in tubules, including the proximal tubule and collecting ducts in the rodent kidney. EETs have been shown to inhibit ENaC activity, which may contribute to their natriuretic effect. EETs have also been shown to mediate the natriuretic effect of angiotensin II. Studies of knockout mice have supported a role for CYP450 metabolism in the regulation of blood pressure, but the mechanisms of these effects remain incompletely understood. Genetic disruption of CYP2J8 was associated with hypertension in females, but it is uncertain whether this effect is related to its arachidonate epoxygenase activity in mice or an effect on estrogen metabolism. Genetic disruption of cyp4a10 and 4a14 are also associated with hypertension, adding to uncertainty regarding the distinct roles of epoxygenase and ω-hydroxylase activity of arachidonic acid in regulating vascular tone and blood pressure.
Role of Renal CYP450-Derived Arachidonate Metabolites in Renal Damage
Increased EET formation has been reported in the kidney of rats with liver cirrhosis. While it is well-documented that renal vasoconstriction leading to impaired renal function occurs during cirrhosis, this result suggests increased EET synthesis may be a homeostatic response to help preserve renal perfusion. Reduced CYP arachidonate hydroxyglase activity and 20-HETE levels have been reported in the kidney following ischemia and reperfusion. Reduced CYP4A protein expression and enzyme activity in ischemia/reperfusion was suggested as an adaptive mechanism to preserve renal vasculature from excessive vasoconstriction. Other studies suggest that the CYP hydroxylase-derived product 20-HETE plays an important role in the maintenance of the glomerular protein permeability barrier. An in vitro glomerular albumin permeability study using isolated rat glomeruli shows that puromycin aminonucleoside (PAN) significantly increases glomerular albumin permeability. 20-HETE treatment blocks PAN-induced increase in albumin permeability.
Summary
Eicosanoids exert diverse and sometimes self-opposing functions. The specific effect of each eicosanoid depends on sequential enzymatic machinery in a specific cell, yielding a specific eicosanoid, exerting its distinct function. The biosynthesis of each eicosanoid is regulated at multiple levels from phospholipase A2 that catalyzes the release of arachidonic acid to specific enzymes that catalyze the formation of bioactive eicosanoids. Arachidonate-derived eicosanoids including prostanoids, leukotrienes, 12/15-HETEs, EETs, and HETEs, and sphingomyelin-derived ceramide play important roles in maintaining normal renal function. They are also involved in the pathophysiology of diabetic nephropathy, and inflammatory or toxic glomerular injury. Those signaling pathways should provide a fruitful area to identify targets for intervention in the pharmacologic treatment of renal disease.
References
- [1]. Page J., and Henry D.: Consumption of NSAIDs and the development of congestive heart failure in elderly patients: an underrecognized public health problem. Arch Intern Med 2000; 160: pp. 777-784
- [2]. White W.B.: Cardiovascular risk, hypertension, and NSAIDs. Curr Rheumatol Rep 2007; 9: pp. 36-43
- [3]. Breyer M.D., and Harris R.C.: Cyclooxygenase 2 and the kidney. Curr Opin Nephrol Hypertens 2001; 10: pp. 89-98
- [4]. Eras J., and Perazella M.A.: NSAIDs and the kidney revisited: are selective cyclooxygenase-2 inhibitors safe? Am J Med Sci 2001; 321: pp. 181-190
- [5]. Winkelmayer W.C., Waikar S.S., Mogun H., and Solomon D.H.: Nonselective and cyclooxygenase-2-selective NSAIDs and acute kidney injury. Am J Med 2008; 121: pp. 1092-1098
- [6]. Hansen A.E., and Burr G.O.: Essential fatty acids and human nutrition. J Am Med Assoc 1946; 132: pp. 855-859
- [7]. De Caterina R.: n-3 Fatty acids in cardiovascular disease. N Engl J Med 2011; 364: pp. 2439-2450
- [8]. Burr G.O., and Burr M.M.: Nutrition classics from The Journal of Biological Chemistry 82:345-67, 192[9] A new deficiency disease produced by the rigid exclusion of fat from the diet. Nutr Rev 1973; 31: pp. 248-249
- [9]. Lands W.E.: The biosynthesis and metabolism of prostaglandins. Annu Rev Physiol 1979; 41: pp. 633-652
- [10]. Dunham E.W., Balasingam M., Privett O.S., and Nickell E.C.: Effects of essential fatty acid deficiency on prostaglandin synthesis and fatty acid composition in rat renal medulla. Lipids 1978; 13: pp. 892-897
- [11]. Moussa M., Garcia J., Ghisolfi J., Periquet B., and Thouvenot J.P.: Dietary essential fatty acid deficiency differentially affects tissues of rats. J Nutr 1996; 126: pp. 3040-3045
- [12]. Soares A.F., Santiago R.C., Alessio M.L., Descomps B., and de Castro-Chaves C.: Biochemical, functional, and histochemical effects of essential fatty acid deficiency in rat kidney. Lipids 2005; 40: pp. 1125-1133
- [13]. Boyanovsky B.B., and Webb N.R.: Biology of secretory phospholipase A2. Cardiovasc Drugs Ther 2009; 23: pp. 61-72
- [14]. Burke J.E., and Dennis E.A.: Phospholipase A2 structure/function, mechanism, and signaling. J Lipid Res 2009; 50: pp. S237-S242
- [15]. Boulven I., Palmier B., Robin P., Vacher M., Harbon S., and Leiber D.: Platelet-derived growth factor stimulates phospholipase C-gamma 1, extracellular signal-regulated kinase, and arachidonic acid release in rat myometrial cells: contribution to cyclic 3′,5′-adenosine monophosphate production and effect on cell proliferation. Biol Reprod 2001; 65: pp. 496-506
- [16]. Fujishima H., Sanchez Mejia R.O., Bingham C.O., Lam B.K., Sapirstein A., Bonventre J.V., et al: Cytosolic phospholipase A2 is essential for both the immediate and the delayed phases of eicosanoid generation in mouse bone marrow-derived mast cells. Proc Natl Acad Sci USA 1999; 96: pp. 4803-4807
- [17]. Samad T.A., Moore K.A., Sapirstein A., Billet S., Allchorne A., Poole S., et al: Interleukin-1beta-mediated induction of Cox-2 in the CNS contributes to inflammatory pain hypersensitivity. Nature 2001; 410: pp. 471-475
- [18]. Sapirstein A., and Bonventre J.V.: Specific physiological roles of cytosolic phospholipase A(2) as defined by gene knockouts. Biochim Biophys Acta 2000; 1488: pp. 139-148
- [19]. Kudo I., and Murakami M.: Phospholipase A2 enzymes. Prostag Oth Lipid M 2002; 68-69: pp. 3-58
- [20]. Mangat H., Peterson L.N., and Burns K.D.: Hypercalcemia stimulates expression of intrarenal phospholipase A2 and prostaglandin H synthase-2 in rats. Role of angiotensin II AT1 receptors. J Clin Invest 1997; 100: pp. 1941-1950
- [21]. Pavoine C., Behforouz N., Gauthier C., Le Gouvello S., Roudot-Thoraval F., Martin C.R., et al: beta2-Adrenergic signaling in human heart: shift from the cyclic AMP to the arachidonic acid pathway. Mol Pharmacol 2003; 64: pp. 1117-1125
- [22]. Xing M., and Insel P.A.: Protein kinase C-dependent activation of cytosolic phospholipase A2 and mitogen-activated protein kinase by alpha 1-adrenergic receptors in Madin–Darby canine kidney cells. J Clin Invest 1996; 97: pp. 1302-1310
- [23]. Jiao H., Cui X.L., Torti M., Chang C.H., Alexander L.D., Lapetina E.G., et al: Arachidonic acid mediates angiotensin II effects on p21ras in renal proximal tubular cells via the tyrosine kinase-Shc-Grb2-Sos pathway. Proc Natl Acad Sci USA 1998; 95: pp. 7417-7421
- [24]. Marin Castano M.E., Schanstra J.P., Hirtz C., Pesquero J.B., Pecher C., Girolami J.P., et al: B2 kinin receptor upregulation by cAMP is associated with BK-induced PGE2 production in rat mesangial cells. Am J Physiol 1998; 274: pp. F532-F540
- [25]. Murakami M., Kambe T., Shimbara S., and Kudo I.: Functional coupling between various phospholipase A2s and cyclooxygenases in immediate and delayed prostanoid biosynthetic pathways. J Biol Chem 1999; 274: pp. 3103-3115
- [26]. Jacobs L.S., and Douglas J.G.: Angiotensin II type 2 receptor subtype mediates phospholipase A2-dependent signaling in rabbit proximal tubular epithelial cells. Hypertension 1996; 28: pp. 663-668
- [27]. Slivka S.R., and Insel P.A.: Alpha 1-adrenergic receptor-mediated phosphoinositide hydrolysis and prostaglandin E2 formation in Madin–Darby canine kidney cells. Possible parallel activation of phospholipase C and phospholipase A2. J Biol Chem 1987; 262: pp. 4200-4207
- [28]. Choi K.H., Edelstein C.L., Gengaro P., Schrier R.W., and Nemenoff R.A.: Hypoxia induces changes in phospholipase A2 in rat proximal tubules: evidence for multiple forms. Am J Physiol 1995; 269: pp. F846-F853
- [29]. Osada-Oka M., Takahashi M., Akiba S., and Sato T.: Involvement of Ca . Eur J Pharmacol 2006; 549: pp. 58-62
- [30]. Petry C., Huwiler A., Eberhardt W., Kaszkin M., and Pfeilschifter J.: Hypoxia increases group IIA phospholipase A(2) expression under inflammatory conditions in rat renal mesangial cells. J Am Soc Nephrol 2005; 16: pp. 2897-2905
- [31]. Goto S., Nakamura H., Morooka H., Terao Y., Shibata O., and Sumikawa K.: Role of reactive oxygen in phospholipase A2 activation by ischemia/reperfusion of the rat kidney. J Anesth 1999; 13: pp. 90-93
- [32]. Alexander L.D., Alagarsamy S., and Douglas J.G.: Cyclic stretch-induced cPLA2 mediates ERK 1/2 signaling in rabbit proximal tubule cells. Kidney Int 2004; 65: pp. 551-563
- [33]. Bonventre J.V.: The 85-kD cytosolic phospholipase A2 knockout mouse: a new tool for physiology and cell biology. J Am Soc Nephrol 1999; 10: pp. 404-412
- [34]. DeRubertis F.R., and Craven P.A.: Eicosanoids in the pathogenesis of the functional and structural alterations of the kidney in diabetes. Am J Kidney Dis 1993; 22: pp. 727-735
- [35]. Beck S., Beck G., Ostendorf T., Floege J., Lambeau G., Nevalainen T., et al: Upregulation of group IB secreted phospholipase A(2) and its M-type receptor in rat ANTI-THY-1 glomerulonephritis. Kidney Int 2006; 70: pp. 1251-1260
- [36]. Zager R.A., Schimpf B.A., Gmur D.J., and Burke T.J.: Phospholipase A2 activity can protect renal tubules from oxygen deprivation injury. Proc Natl Acad Sci USA 1993; 90: pp. 8297-8301
- [37]. dos Reis M.A., Costa R.S., Coimbra T.M., and Teixeira V.P.: Acute renal failure in experimental envenomation with Africanized bee venom. Ren Fail 1998; 20: pp. 39-51
- [38]. Takasaki J., Kawauchi Y., Urasaki T., Tanaka H., Usuda S., and Masuho Y.: Antibodies against type II phospholipase A2 prevent renal injury due to ischemia and reperfusion in rats. FEBS Lett 1998; 440: pp. 377-381
- [39]. Beck L.H., Bonegio R.G., Lambeau G., Beck D.M., Powell D.W., Cummins T.D., et al: M-type phospholipase A2 receptor as target antigen in idiopathic membranous nephropathy. N Engl J Med 2009; 361: pp. 11-21
- [40]. Lambeau G., and Lazdunski M.: Receptors for a growing family of secreted phospholipases A2. Trends Pharmacol Sci 1999; 20: pp. 162-170
- [41]. Rouault M., Le Calvez C., Boilard E., Surrel F., Singer A., Ghomashchi F., et al: Recombinant production and properties of binding of the full set of mouse secreted phospholipases A2 to the mouse M-type receptor. Biochemistry 2007; 46: pp. 1647-1662
- [42]. Augert A., Payre C., de Launoit Y., Gil J., Lambeau G., and Bernard D.: The M-type receptor PLA2R regulates senescence through the p53 pathway. EMBO Rep 2009; 10: pp. 271-277
- [43]. Fitzpatrick F.A., and Soberman R.: Regulated formation of eicosanoids. J Clin Invest 2001; 107: pp. 1347-1351
- [44]. FitzGerald G.A., and Loll P.: COX in a crystal ball: current status and future promise of prostaglandin research. J Clin Invest 2001; 107: pp. 1335-1337
- [45]. Smith W.L., DeWitt D.L., and Garavito R.M.: Cyclooxygenases: structural, cellular, and molecular biology. Annu Rev Biochem 2000; 69: pp. 145-182
- [46]. Funk C.D.: Prostaglandins and leukotrienes: advances in eicosanoid biology. Science 2001; 294: pp. 1871-1875
- [47]. Murphy R.C., and Gijon M.A.: Biosynthesis and metabolism of leukotrienes. Biochem J 2007; 405: pp. 379-395
- [48]. Capdevila J.H.: Regulation of ion transport and blood pressure by cytochrome p450 monooxygenases. Curr Opin Nephrol Hypertens 2007; 16: pp. 465-470
- [49]. Fleming I.: Epoxyeicosatrienoic acids, cell signaling and angiogenesis. Prostaglandins Other Lipid Mediat 2007; 82: pp. 60-67
- [50]. Zeldin D.C.: Epoxygenase pathways of arachidonic acid metabolism. J Biol Chem 2001; 276: pp. 36059-36062
- [51]. Hansen R.A., Ogilvie G.K., Davenport D.J., Gross K.L., Walton J.A., Richardson K.L., et al: Duration of effects of dietary fish oil supplementation on serum eicosapentaenoic acid and docosahexaenoic acid concentrations in dogs. Am J Vet Res 1998; 59: pp. 864-868
- [52]. Smith W.L.: Nutritionally essential fatty acids and biologically indispensable cyclooxygenases. Trends Biochem Sci 2008; 33: pp. 27-37
- [53]. De Caterina R., Caprioli R., Giannessi D., Sicari R., Galli C., Lazzerini G., et al: n-3 Fatty acids reduce proteinuria in patients with chronic glomerular disease. Kidney Int 1993; 44: pp. 843-850
- [54]. Grande J.P., and Donadio J.V.: Dietary fish oil supplementation in IgA nephropathy: a therapy in search of a mechanism? Nutrition 1998; 14: pp. 240-242
- [55]. Garavito M.R., Malkowski M.G., and DeWitt D.L.: The structures of prostaglandin endoperoxide H synthases-1 and -2. Prostag Oth Lipid M 2002; 68-69: pp. 129-152
- [56]. Kiefer J.R., Pawlitz J.L., Moreland K.T., Stegeman R.A., Hood W.F., Gierse J.K., et al: Structural insights into the stereochemistry of the cyclooxygenase reaction. Nature 2000; 405: pp. 97-101
- [57]. Picot D., Loll P.J., and Garavito R.M.: The X-ray crystal structure of the membrane protein prostaglandin H2 synthase-1. Nature 1994; 367: pp. 243-249
- [58]. Yuan C., Sidhu R.S., Kuklev D.V., Kado Y., Wada M., Song I., et al: Cyclooxygenase allosterism, fatty acid-mediated cross-talk between monomers of cyclooxygenase homodimers. J Biol Chem 2009; 284: pp. 10046-10055
- [59]. Yuan C., Rieke C.J., Rimon G., Wingerd B.A., and Smith W.L.: Partnering between monomers of cyclooxygenase-2 homodimers. PNAS 2006; 103: pp. 6142-6147
- [60]. Mbonye U.R., Wada M., Rieke C.J., Tang H.Y., Dewitt D.L., and Smith W.L.: The 19-amino acid cassette of cyclooxygenase-2 mediates entry of the protein into the endoplasmic reticulum-associated degradation system. J Biol Chem 2006; 281: pp. 35770-35778
- [61]. Sidhu, R. S., Lee, J. Y., Yuan, C., and Smith, W. L. Comparison of cyclooxygenase-1 crystal structures: cross-talk between monomers comprising cyclooxygenase-1 homodimers. Biochemistry 49:7069–7079.
- [62]. Rimon, G., Sidhu, R. S., Lauver, D. A., Lee, J. Y., Sharma, N. P., Yuan, C., et al. Coxibs interfere with the action of aspirin by binding tightly to one monomer of cyclooxygenase-1. Proc Natl Acad Sci USA 107:28–33.
- [63]. Dong L., Vecchio A.J., Sharma N.P., Jurban B.J., Malkowski M.G., and Smith W.L.: Human cyclooxygenase-2 is a sequence homodimer that functions as a conformational heterodimer. J Biol Chem 2011; 286: pp. 19035-19046
- [64]. Kobayashi T., and Narumiya S.: Function of prostanoid receptors: studies on knockout mice. Prostaglandins Other Lipid Mediat 2002; 68-69: pp. 557-573
- [65]. Narumiya S., Sugimoto Y., and Ushikubi F.: Prostanoid receptors: structures, properties, and functions. Physiol Rev 1999; 79: pp. 1193-1226
- [66]. Kliewer S., Lenhard J., Wilson T., Patel I., Morris D., and Lehmann J.: A prostaglandin J . Cell 1995; 83: pp. 813-819
- [67]. Narumiya S., Ohno K., Fujiwara M., and Fukushima M.: Site and mechanism of growth inhibition by prostaglandins. II. Temperature-dependent transfer of a cyclopentenone prostaglandin to nuclei. J Pharmacol Exp Ther 1986; 239: pp. 506-511
- [68]. Reginato M.J., Krakow S.L., Bailey S.T., and Lazar M.A.: Prostaglandins promote and block adipogenesis through opposing effects on peroxisome proliferator-activated receptor gamma. J Biol Chem 1998; 273: pp. 1855-1858
- [69]. Hla T., and Neilson K.: Human cyclooxygenase-2 cDNA. Proc Natl Acad Sci USA 1992; 89: pp. 7384-7388
- [70]. Kujubu D.A., Fletcher B.S., Varnum B.C., Lim R.W., and Herschman H.R.: TIS10, a phorbol ester tumor promoter-inducible mRNA from Swiss 3T3 cells, encodes a novel prostaglandin synthase/cyclooxygenase homologue. J Biol Chem 1991; 266: pp. 12866-12872
- [71]. Kujubu D.A., and Herschman H.R.: Dexamethasone inhibits mitogen induction of the TIS10 prostaglandin synthase/cyclooxygenase gene. J Biol Chem 1992; 267: pp. 7991-7994
- [72]. Tanabe T., and Tohnai N.: Cyclooxygenase isozymes and their gene structures and expression. Prostaglandins Other Lipid Mediat 2002; 68-69: pp. 95-114
- [73]. Cheng Y., Wang M., Yu Y., Lawson J., Funk C.D., and Fitzgerald G.A.: Cyclooxygenases, microsomal prostaglandin E synthase-1, and cardiovascular function. J Clin Invest 2006; 116: pp. 1391-1399
- [74]. Hla T., Bishop-Bailey D., Liu C.H., Schaefers H.J., and Trifan O.C.: Cyclooxygenase-1 and -2 isoenzymes. Int J Biochem Cell Biol 1999; 31: pp. 551-557
- [75]. Herschman H.R.: Prostaglandin synthase 2. Bba-Lipid Lipid Metab 1996; 1299: pp. 125-140
- [76]. Smith W.L.: Prostanoid biosynthesis and mechanisms of action. Am J Physiol 1992; 263: pp. F181-F191
- [77]. Smith W.L., and Langenbach R.: Why there are two cyclooxygenase isozymes? J Clin Invest 2001; 107: pp. 1491-1495
- [78]. Dinchuk J.E., Car B.D., Focht R.J., Johnston J.J., Jaffee B.D., Covington M.B., et al: Renal abnormalities and an altered inflammatory response in mice lacking cyclooxygenase II. Nature 1995; 378: pp. 406-409
- [79]. Komhoff M., Wang J.L., Cheng H.F., Langenbach R., McKanna J.A., Harris R.C., et al: Cyclooxygenase-2-selective inhibitors impair glomerulogenesis and renal cortical development. Kidney Int 2000; 57: pp. 414-422
- [80]. Morham S.G., Langenbach R., Loftin C.D., Tiano H.F., Vouloumanos N., Jennette J.C., et al: Prostaglandin synthase 2 gene disruption causes severe renal pathology in the mouse. Cell 1995; 83: pp. 473-482
- [81]. Harris R.C., McKanna J.A., Akai Y., Jacobson H.R., Dubois R.N., and Breyer M.D.: Cyclooxygenase-2 is associated with the macula densa of rat kidney and increases with salt restriction. J Clin Invest 1994; 94: pp. 2504-2510
- [82]. Komhoff M., Jeck N.D., Seyberth H.W., Grone H.J., Nusing R.M., and Breyer M.D.: Cyclooxygenase-2 expression is associated with the renal macula densa of patients with Bartter-like syndrome. Kidney Int 2000; 58: pp. 2420-2424
- [83]. Vio C.P., An S.J., Cespedes C., McGiff J.C., and Ferreri N.R.: Induction of cyclooxygenase-2 in thick ascending limb cells by adrenalectomy. J Am Soc Nephrol 2001; 12: pp. 649-658
- [84]. Campean V., Theilig F., Paliege A., Breyer M., and Bachmann S.: Key enzymes for renal prostaglandin synthesis: site-specific expression in rodent kidney (rat, mouse). Am J Physiol Renal Physiol 2003; 285: pp. F19-F32
- [85]. Smith W.L., and Bell T.G.: Immunohistochemical localization of the prostaglandin-forming cyclooxygenase in renal cortex. Am J Physiol 1978; 235: pp. F451-F457
- [86]. Yang T., Singh I., Pham H., Sun D., Smart A., Schnermann J.B., et al: Regulation of cyclooxygenase expression in the kidney by dietary salt intake. Am J Physiol 1998; 274: pp. F481-F489
- [87]. Castrop H., Schweda F., Schumacher K., Wolf K., and Kurtz A.: Role of renocortical cyclooxygenase-2 for renal vascular resistance and macula densa control of renin secretion. J Am Soc Nephrol 2001; 12: pp. 867-874
- [88]. Guan Y., Chang M., Cho W., Zhang Y., Redha R., Davis L., et al: Cloning, expression, and regulation of rabbit cyclooxygenase-2 in renal medullary interstitial cells. Am J Physiol 1997; 273: pp. F18-F26
- [89]. Hao C.M., Komhoff M., Guan Y., Redha R., and Breyer M.D.: Selective targeting of cyclooxygenase-2 reveals its role in renal medullary interstitial cell survival. Am J Physiol 1999; 277: pp. F352-F359
- [90]. Bresalier R.S., Sandler R.S., Quan H., Bolognese J.A., Oxenius B., Horgan K., et al: Cardiovascular events associated with rofecoxib in a colorectal adenoma chemoprevention trial. N Engl J Med 2005; 352: pp. 1092-1102
- [91]. Zewde T., and Mattson D.L.: Inhibition of cyclooxygenase-2 in the rat renal medulla leads to sodium-sensitive hypertension. Hypertension 2004; 44: pp. 424-428
- [92]. Zhang M.Z., Yao B., Cheng H.F., Wang S.W., Inagami T., and Harris R.C.: Renal cortical cyclooxygenase 2 expression is differentially regulated by angiotensin II AT(1) and AT(2) receptors. Proc Natl Acad Sci USA 2006; 103: pp. 16045-16050
- [93]. Peti-Peterdi J., Komlosi P., Fuson A.L., Guan Y., Schneider A., Qi Z., et al: Luminal NaCl delivery regulates basolateral PGE2 release from macula densa cells. J Clin Invest 2003; 112: pp. 76-82
- [94]. Helliwell R.J., Adams L.F., and Mitchell M.D.: Prostaglandin synthases: recent developments and a novel hypothesis. Prostaglandins Leukot Essent Fatty Acids 2004; 70: pp. 101-113
- [95]. Urade Y., Watanabe K., and Hayaishi O.: Prostaglandin D, E, and F synthases. J Lipid Mediat Cell Signal 1995; 12: pp. 257-273
- [96]. Breyer M.D., and Breyer R.M.: Prostaglandin E receptors and the kidney. Am J Physiol Renal Physiol 2000; 279: pp. F12-F23
- [97]. Narumiya S., and FitzGerald G.A.: Genetic and pharmacological analysis of prostanoid receptor function. J Clin Invest 2001; 108: pp. 25-30
- [98]. Qi Z., Cai H., Morrow J.D., and Breyer M.D.: Differentiation of cyclooxygenase 1- and 2-derived prostanoids in mouse kidney and aorta. Hypertension 2006; 48: pp. 323-328
- [99]. Murphey L.J., Williams M.K., Sanchez S.C., Byrne L.M., Csiki I., Oates J.A., et al: Quantification of the major urinary metabolite of PGE2 by a liquid chromatographic/mass spectrometric assay: Determination of cyclooxygenase-specific PGE2 synthesis in healthy humans and those with lung cancer. Anal Biochem 2004; 334: pp. 266-275
- [100]. Whelton A., Schulman G., Wallemark C., Drower E.J., Isakson P.C., Verburg K.M., et al: Effects of celecoxib and naproxen on renal function in the elderly. Arch Intern Med 2000; 160: pp. 1465-1470
- [101]. Kammerl M.C., Nusing R.M., Schweda F., Endemann D., Stubanus M., Kees F., et al: Low sodium and furosemide-induced stimulation of the renin system in man is mediated by cyclooxygenase 2. Clin Pharmacol Ther 2001; 70: pp. 468-474
- [102]. Breyer R.M., Kennedy C.R., Zhang Y., and Breyer M.D.: Structure–function analyses of eicosanoid receptors. Physiologic and therapeutic implications. Ann NY Acad Sci 2000; 905: pp. 221-231
- [103]. Sugimoto Y., and Narumiya S.: Prostaglandin E receptors. J Biol Chem 2007; 282: pp. 11613-11617
- [104]. Hebert R.L., Carmosino M., Saito O., Yang G., Jackson C.M., Qi Z., et al: Characterization of a rabbit PGF2alpha (FP) receptor exhibiting Gi-restricted signaling and that inhibits water absorption in renal collecting duct. J Biol Chem 2005; 280: pp. 35028-35037
- [105]. Breyer M.D., Davis L., Jacobson H.R., and Breyer R.M.: Differential localization of prostaglandin E receptor subtypes in human kidney. Am J Physiol 1996; 270: pp. F912-F918
- [106]. Tilley S.L., Coffman T.M., and Koller B.H.: Mixed messages: modulation of inflammation and immune responses by prostaglandins and thromboxanes. J Clin Invest 2001; 108: pp. 15-23
- [107]. Cronstein B.N., and Weissmann G.: Targets for antiinflammatory drugs. Annu Rev Pharmacol Toxicol 1995; 35: pp. 449-462
- [108]. Honda T., Tokura Y., Miyachi Y., and Kabashima K.: Prostanoid receptors as possible targets for anti-allergic drugs: recent advances in prostanoids on allergy and immunology. Curr Drug Targets 2010; 11: pp. 1605-1613
- [109]. Jakobsson P.J., Thoren S., Morgenstern R., and Samuelsson B.: Identification of human prostaglandin E synthase: a microsomal, glutathione-dependent, inducible enzyme, constituting a potential novel drug target. Proc Natl Acad Sci USA 1999; 96: pp. 7220-7225
- [110]. Tanioka T., Nakatani Y., Semmyo N., Murakami M., and Kudo I.: Molecular identification of cytosolic prostaglandin E2 synthase that is functionally coupled with cyclooxygenase-1 in immediate prostaglandin E2 biosynthesis. J Biol Chem 2000; 275: pp. 32775-32782
- [111]. Tanikawa N., Ohmiya Y., Ohkubo H., Hashimoto K., Kangawa K., Kojima M., et al: Identification and characterization of a novel type of membrane-associated prostaglandin E synthase. Biochem Biophys Res Commun 2002; 291: pp. 884-889
- [112]. Trebino C.E., Stock J.L., Gibbons C.P., Naiman B.M., Wachtmann T.S., Umland J.P., et al: Impaired inflammatory and pain responses in mice lacking an inducible prostaglandin E synthase. Proc Natl Acad Sci USA 2003; 100: pp. 9044-9049
- [113]. Engblom D., Saha S., Engstrom L., Westman M., Audoly L.P., Jakobsson P. -J., et al: Microsomal prostaglandin E synthase-1 is the central switch during immune-induced pyresis. Nat Neurosci 2003; 6: pp. 1137-1138
- [114]. Uematsu S., Matsumoto M., Takeda K., and Akira S.: Lipopolysaccharide-dependent prostaglandin E(2) production is regulated by the glutathione-dependent prostaglandin E(2) synthase gene induced by the Toll-like receptor 4/MyD88/NF-IL6 pathway. J Immunol 2002; 168: pp. 5811-5816
- [115]. Schneider A., Zhang Y., Zhang M., Lu W.J., Rao R., Fan X., et al: Membrane-associated PGE synthase-1 (mPGES-1) is coexpressed with both COX-1 and COX-2 in the kidney. Kidney Int 2004; 65: pp. 1205-1213
- [116]. Guan Y., Zhang Y., Schneider A., Riendeau D., Mancini J.A., Davis L., et al: Urogenital distribution of a mouse membrane-associated prostaglandin E(2) synthase. Am J Physiol Renal Physiol 2001; 281: pp. F1173-F1177
- [117]. Ouellet M., Falgueyret J.P., Hien Ear P., Pen A., Mancini J.A., Riendeau D., et al: Purification and characterization of recombinant microsomal prostaglandin E synthase-1. Protein Expr Purif 2002; 26: pp. 489-495
- [118]. Jia Z., Aoyagi T., and Yang T.: mPGES-1 protects against DOCA-salt hypertension via inhibition of oxidative stress or stimulation of NO/cGMP. Hypertension 2010; 55: pp. 539-546
- [119]. Jia Z., Guo X., Zhang H., Wang M.H., Dong Z., and Yang T.: Microsomal prostaglandin synthase-1-derived prostaglandin E2 protects against angiotensin II-induced hypertension via inhibition of oxidative stress. Hypertension 2008; 52: pp. 952-959
- [120]. Francois H., Facemire C., Kumar A., Audoly L., Koller B., and Coffman T.: Role of microsomal prostaglandin E synthase 1 in the kidney. J Am Soc Nephrol 2007; 18: pp. 1466-1475
- [121]. Nguyen M., Camenisch T., Snouwaert J.N., Hicks E., Coffman T.M., Anderson P.A., et al: The prostaglandin receptor EP4 triggers remodelling of the cardiovascular system at birth. Nature 1997; 390: pp. 78-81
- [122]. Murakami M., Nakatani Y., Tanioka T., and Kudo I.: Prostaglandin E synthase. Prostaglandins Other Lipid Mediat 2002; 68-69: pp. 383-399
- [123]. Zhang Y., Schneider A., Rao R., Lu W.J., Fan X., Davis L., et al: Genomic structure and genitourinary expression of mouse cytosolic prostaglandin E(2) synthase gene. Biochim Biophys Acta 2003; 1634: pp. 15-23
- [124]. Breyer R.M., Davis L.S., Nian C., Redha R., Stillman B., Jacobson H.R., et al: Cloning and expression of the rabbit prostaglandin EP4 receptor. Am J Physiol 1996; 270: pp. F485-F493
- [125]. Guan Y., Stillman B.A., Zhang Y., Schneider A., Saito O., Davis L.S., et al: Cloning and expression of the rabbit prostaglandin EP2 receptor. BMC Pharmacol 2002; 2: pp. 14
- [126]. Jensen B.L., Stubbe J., Hansen P.B., Andreasen D., and Skott O.: Localization of prostaglandin E(2) EP2 and EP4 receptors in the rat kidney. Am J Physiol Renal Physiol 2001; 280: pp. F1001-F1009
- [127]. Sheldrick R., Coleman R., and Lumley P.: Iloprost-A potent EP1- and IP- receptor agonist. Br J Pharmacol 1987; undefined:
- [128]. Coleman R.A., Kennedy I., Humphrey P.P.A., Bunce K., and Lumley P.: Prostanoids and their Receptors. In Emmet J.C. (eds): Comprehensive Medicinal Chemistry. Oxford: Pergammon Press, 1990. pp. 643-714
- [129]. Coleman R.A., Smith W.L., and Narumiya S.: VIII. International union of pharmacology classification of prostanoid receptors: properties, distribution, and structure of the receptors and their subtypes. Pharmacol Rev 1994; 46: pp. 205-229
- [130]. Lawrence R.A., Jones R.L., and Wilson N.H.: Characterization of receptors involved in the direct and indirect actions of prostaglandins E and I on the guinea-pig ileum. Br J Pharmacol 1992; 105: pp. 271-278
- [131]. Funk C., Furchi L., FitzGerald G., Grygorczyk R., Rochette C., Bayne M.A., et al: Cloning and expression of a cDNA for the human prostaglandin E receptor EP . J Biol Chem 1993; 268: pp. 26767-26772
- [132]. Guan Y., Zhang Y., Breyer R.M., Fowler B., Davis L., Hebert R.L., et al: Prostaglandin E2 inhibits renal collecting duct Na . J Clin Invest 1998; 102: pp. 194-201
- [133]. Hibbs T.A., Lu B., Smock S.L., Vestergaard P., Pan L.C., and Owen T.A.: Molecular cloning and characterization of the canine prostaglandin E receptor EP2 subtype. Prostaglandins Other Lipid Mediat 1999; 57: pp. 133-147
- [134]. Watabe A., Sugimoto Y., Honda A., Irie A., Namba T., Negishi M., et al: Cloning and expression of cDNA for a mouse EP1 subtype of prostaglandin E receptor. J Biol Chem 1993; 268: pp. 20175-20178
- [135]. Boie Y., Stocco R., Sawyer N., Slipetz D.M., Ungrin M.D., Neuschafer-Rube F., et al: Molecular cloning and characterization of the four rat prostaglandin E2 prostanoid receptor subtypes. Eur J Pharmacol 1997; 340: pp. 227-241
- [136]. Hallinan E.A., Hagen T.J., Tsymbalov S., Husa R.K., Lee A.C., Stapelfeld A., et al: Aminoacetyl moiety as a potential surrogate for diacylhydrazine group of SC-51089, a potent PGE2 antagonist, and its analogs. J Med Chem 1996; 39: pp. 609-613
- [137]. Hallinan E.A., Hagen T.J., Tsymbalov S., Stapelfeld A., and Savage M.A.: 2,4-Disubstituted oxazoles and thiazoles as latent pharmacophores for diacylhydrazine of SC-51089, a potent PGE2 antagonist. Bioorg Med Chem 2001; 9: pp. 1-6
- [138]. Hall A., Atkinson S., Brown S.H., Chessell I.P., Chowdhury A., Giblin G.M., et al: Discovery of novel, non-acidic 1,5-biaryl pyrrole EP1 receptor antagonists. Bioorg Med Chem Lett 2007; 17: pp. 1200-1205
- [139]. Makino H., Tanaka I., Mukoyama M., Sugawara A., Mori K., Muro S., et al: Prevention of diabetic nephropathy in rats by prostaglandin E receptor EP1-selective antagonist. J Am Soc Nephrol 2002; 13: pp. 1757-1765
- [140]. Miki T., Matsunami M., Nakamura S., Okada H., Matsuya H., and Kawabata A.: ONO-8130, a selective prostanoid EP1 receptor antagonist, relieves bladder pain in mice with cyclophosphamide-induced cystitis. Pain 2011; 152: pp. 1373-1381
- [141]. Naganawa A., Matsui T., Ima M., Saito T., Murota M., Aratani Y., et al: Further optimization of sulfonamide analogs as EP1 receptor antagonists: synthesis and evaluation of bioisosteres for the carboxylic acid group. Bioorg Med Chem 2006; 14: pp. 7121-7137
- [142]. Johansson T., Narumiya S., and Zeilhofer H.U.: Contribution of peripheral versus central EP1 prostaglandin receptors to inflammatory pain. Neurosci Lett 2011; 495: pp. 98-101
- [143]. Stock J.L., Shinjo K., Burkhardt J., Roach M., Taniguchi K., Ishikawa T., et al: The prostaglandin E2 EP1 receptor mediates pain perception and regulates blood pressure. J Clin Invest 2001; 107: pp. 325-331
- [144]. Biswas S., Bhattacherjee P., Paterson C.A., Maruyama T., and Narumiya S.: Modulation of ocular inflammatory responses by EP1 receptors in mice. Exp Eye Res 2007; 84: pp. 39-43
- [145]. Guan Y., Zhang Y., Wu J., Qi Z., Yang G., Dou D., et al: Antihypertensive effects of selective prostaglandin E2 receptor subtype 1 targeting. J Clin Invest 2007; 117: pp. 2496-2505
- [146]. Sugimoto Y., Namba T., Shigemoto R., Negishi M., Ichikawa A., and Narumiya S.: Distinct cellular localization of mRNAs for three subtypes of prostaglandin E receptor in kidney. Am J Physiol 1994; 266: pp. F823-F828
- [147]. Capone C., Faraco G., Anrather J., Zhou P., and Iadecola C.: Cyclooxygenase 1-derived prostaglandin E2 and EP1 receptors are required for the cerebrovascular dysfunction induced by angiotensin II. Hypertension 2010; 55: pp. 911-917
- [148]. Pena-Silva R.A., and Heistad D.D.: EP1c times for angiotensin: EP1 receptors facilitate angiotensin II-induced vascular dysfunction. Hypertension 2010; 55: pp. 846-848
- [149]. Rutkai I., Feher A., Erdei N., Henrion D., Papp Z., Edes I., et al: Activation of prostaglandin E2 EP1 receptor increases arteriolar tone and blood pressure in mice with type 2 diabetes. Cardiovascular Research 2009; 83: pp. 148-154
- [150]. Regan J.W., Bailey T.J., Pepperl D.J., Pierce K.L., Bogardus A.M., Donello J.E., et al: Cloning of a novel human prostaglandin receptor with characteristics of the pharmacologically defined EP2 subtype. Mol Pharmacol 1994; 46: pp. 213-220
- [151]. Nishigaki N., Negishi M., Honda A., Sugimoto Y., Namba T., Narumiya S., et al: Identification of prostaglandin E receptor ‘EP2 cloned from mastocytoma cells as EP4 subtype. FEBS Letters 1995; 364: pp. 339-341
- [152]. Katsuyama M., Nishigaki N., Sugimoto Y., Morimoto K., Negishi M., Narumiya S., et al: The mouse prostaglandin E receptor EP2 subtype: cloning, expression, and Northern blot analysis. FEBS Lett 1995; 372: pp. 151-156
- [153]. Kennedy C.R., Zhang Y., Brandon S., Guan Y., Coffee K., Funk C.D., et al: Salt-sensitive hypertension and reduced fertility in mice lacking the prostaglandin EP2 receptor. Nat Med 1999; 5: pp. 217-220
- [154]. Tilley S.L., Audoly L.P., Hicks E.H., Kim H.S., Flannery P.J., Coffman T.M., et al: Reproductive failure and reduced blood pressure in mice lacking the EP2 prostaglandin E2 receptor. J Clin Invest 1999; 103: pp. 1539-1545
- [155]. Pallone T.: Vasoconstriction of outer medullary vasa recta by angiotensin II is modulated by prostaglandin E2. Am J Physiol 1994; 266: pp. F850-F857
- [156]. Pallone T.L., and Silldorff E.P.: Pericyte regulation of renal medullary blood flow. Exp Nephrol 2001; 9: pp. 165-170
- [157]. Imig J.D., Breyer M.D., and Breyer R.M.: Contribution of prostaglandin EP(2) receptors to renal microvascular reactivity in mice. Am J Physiol Renal Physiol 2002; 283: pp. F415-F422
- [158]. Chen J., Zhao M., He W., Milne G.L., Howard J.R., Morrow J., et al: Increased dietary NaCl induces renal medullary PGE2 production and natriuresis via the EP2 receptor. Am J Physiol Renal Physiol 2008; 295: pp. F818-F825
- [159]. Savage M.A., Moummi C., Karabatsos P.J., and Lanthorn T.H.: SC-46275: a potent and highly selective agonist at the EP3 receptor. Prostaglandins Leukot Essent Fatty Acids 1993; 49: pp. 939-943
- [160]. van Rodijnen W.F., Korstjens I.J., Legerstee N., Ter Wee P.M., and Tangelder G.J.: Direct vasoconstrictor effect of prostaglandin E2 on renal interlobular arteries: role of the EP3 receptor. Am J Physiol Renal Physiol 2007; 292: pp. F1094-F1101
- [161]. Breyer R.M., Emeson R.B., Tarng J.L., Breyer M.D., Davis L.S., Abromson R.M., et al: Alternative splicing generates multiple isoforms of a rabbit prostaglandin E2 receptor. J Biol Chem 1994; 269: pp. 6163-6169
- [162]. Irie A., Segi E., Sugimoto Y., Ichikawa A., and Negishi M.: Mouse prostaglandin E receptor EP3 subtype mediates calcium signals via Gi in cDNA-transfected Chinese hamster ovary cells. Biochem Biophys Res Commun 1994; 204: pp. 303-309
- [163]. Pierce K.L., and Regan J.W.: Prostanoid receptor heterogeneity through alternative mRNA splicing. Life Sci 1998; 62: pp. 1479-1483
- [164]. Schmid A., Thierauch K.H., Schleuning W.D., and Dinter H.: Splice variants of the human EP3 receptor for prostaglandin E2. Eur J Biochem 1995; 228: pp. 23-30
- [165]. Namba T., Sugimoto Y., Negishi M., Irie A., Ushikubi F., Kakizuka A., et al: Alternative splicing of C-terminal tail of prostaglandin E receptor subtype EP3 determines G-protein specificity. Nature 1993; 365: pp. 166-170
- [166]. Ushikubi F., Segi E., Sugimoto Y., Murata T., Matsuoka T., Kobayashi T., et al: Impaired febrile response in mice lacking the prostaglandin E receptor subtype EP3. Nature 1998; 395: pp. 281-284
- [167]. Hébert, R, Jacobson, H, Breyer, M. PGE2 inhibits AVP induced water flow in cortical collecting ducts by protein kinase C activation. Am J Physiol 1990;259:F318–5.
- [168]. Sonnenburg, WK, Zhu, J, Smith, WL. A prostglandin E receptor coupled to a pertussis toxin-sensitive guanine nucleotide regulatory protein in rabbit cortical collecting tubule cells. J Biol Chem 1990;265:8479–83.
- [169]. Fleming E., Athirakul K., Oliverio M., Key M., Goulet J., Koller B., et al: Urinary concentrating function in mice lacking the EP3 receptors for prostaglandin E2. Am J Physiol 1998; 275: pp. F955-F961
- [170]. Abramovitz M., Adam M., Boie Y., Carriere M., Denis D., Godbout C., et al: The utilization of recombinant prostanoid receptors to determine the affinities and selectivities of prostaglandins and related analogs. Biochim Biophys Acta 2000; 1483: pp. 285-293
- [171]. Bastien L., Sawyer N., Grygorczyk R., Metters K.M., and Adam M.: Cloning, functional expression, and characterization of the human prostaglandin E2 receptor EP2 subtype. J Biol Chem 1994; 269: pp. 11873-11877
- [172]. Fujino H., Salvi S., and Regan J.W.: Differential regulation of phosphorylation of the cAMP response element-binding protein after activation of EP2 and EP4 prostanoid receptors by prostaglandin E2. Mol Pharmacol 2005; 68: pp. 251-259
- [173]. Fujino H., West K.A., and Regan J.W.: Phosphorylation of glycogen synthase kinase-3 and stimulation of T-cell factor signaling following activation of EP2 and EP4 prostanoid receptors by prostaglandin E2. J Biol Chem 2002; 277: pp. 2614-2619
- [174]. Bastien L., Sawyer N., Grygorczyk R., Metters K., and Adam M.: Cloning, functional expression, and characterization of the human prostaglandin E . J Biol Chem 1994; 269: pp. 11873-11877
- [175]. Narko K., Saukkonen K., Ketola I., Butzow R., Heikinheimo M., and Ristimaki A.: Regulated expression of prostaglandin E(2) receptors EP2 and EP4 in human ovarian granulosa-luteal cells. J Clin Endocrinol Metab 2001; 86: pp. 1765-1768
- [176]. Segi E., Sugimoto Y., Yamasaki A., Aze Y., Oida H., Nishimura T., et al: Patent ductus arteriosus and neonatal death in prostaglandin receptor EP4-deficient mice. Biochem Biophys Res Commun 1998; 246: pp. 7-12
- [177]. Lawrence R.A., and Jones R.L.: Investigation of the prostaglandin E (EP-) receptor subtype mediating relaxation of the rabbit jugular vein. Br J Pharmacol 1992; 105: pp. 817-824
- [178]. Schweda F., Klar J., Narumiya S., Nusing R.M., and Kurtz A.: Stimulation of renin release by prostaglandin E2 is mediated by EP2 and EP4 receptors in mouse kidneys. Am J Physiol Renal Physiol 2004; 287: pp. F427-F433
- [179]. Bugge J.F., Stokke E.S., Vikse A., and Kiil F.: Stimulation of renin release by PGE2 and PGI2 infusion in the dog: enhancing effect of ureteral occlusion or administration of ethacrynic acid. Acta Physiol Scand 1990; 138: pp. 193-201
- [180]. Ito S., Carretero O.A., Abe K., Beierwaltes W.H., and Yoshinaga K.: Effect of prostanoids on renin release from rabbit afferent arterioles with and without macula densa. Kidney Int 1989; 35: pp. 1138-1144
- [181]. Nusing R.M., Treude A., Weissenberger C., Jensen B., Bek M., Wagner C., et al: Dominant role of prostaglandin E2 EP4 receptor in furosemide-induced salt-losing tubulopathy: a model for hyperprostaglandin E syndrome/antenatal Bartter syndrome. J Am Soc Nephrol 2005; 16: pp. 2354-2362
- [182]. Tan S.Y., Shapiro R., Franco R., Stockard H., and Mulrow P.J.: Indomethacin-induced prostaglandin inhibition with hyperkalemia. A reversible cause of hyporeninemic hypoaldosteronism. Ann Intern Med 1979; 90: pp. 783-785
- [183]. Kammerl M.C., Nusing R.M., Richthammer W., Kramer B.K., and Kurtz A.: Inhibition of COX-2 counteracts the effects of diuretics in rats. Kidney Int 2001; 60: pp. 1684-1691
- [184]. Reinalter S.C., Jeck N., Brochhausen C., Watzer B., Nusing R.M., Seyberth H.W., et al: Role of cyclooxygenase-2 in hyperprostaglandin E syndrome/antenatal Bartter syndrome. Kidney Int 2002; 62: pp. 253-260
- [185]. Harding P., Sigmon D.H., Alfie M.E., Huang P.L., Fishman M.C., Beierwaltes W.H., et al: Cyclooxygenase-2 mediates increased renal renin content induced by low-sodium diet. Hypertension 1997; 29: pp. 297-302
- [186]. Blackshear J.L., Davidman M., and Stillman M.T.: Identification of risk for renal insufficiency from nonsteroidal anti-inflammatory drugs. Arch Intern Med 1983; 143: pp. 1130-1134
- [187]. Hockel G., and Cowley A.: Prostaglandin E2-induced hypertension in conscious dogs. Am J Physiol 1979; 237: pp. H449-H454
- [188]. Oliver J.A., Pinto J., Sciacca R.R., and Cannon P.J.: Increased renal secretion of norepinephrine and prostaglandin E2 during sodium depletion in the dog. J Clin Invest 1980; 66: pp. 748-756
- [189]. Hartner A., Cordasic N., Goppelt-Struebe M., Veelken R., and Hilgers K.F.: Role of macula densa cyclooxygenase-2 in renovascular hypertension. Am J Physiol Renal Physiol 2002; undefined:
- [190]. Ahmad S.R., Kortepeter C., Brinker A., Chen M., and Beitz J.: Renal failure associated with the use of celecoxib and rofecoxib. Drug Saf 2002; 25: pp. 537-544
- [191]. Perneger T.V., Whelton P.K., and Klag M.J.: Risk of kidney failure associated with the use of acetaminophen, aspirin, and nonsteroidal antiinflammatory drugs. N Engl J Med 1994; 331: pp. 1675-1679
- [192]. Yussim E., Schwartz E., Sidi Y., and Ehrenfeld M.: Acute renal failure precipitated by non-steroidal anti-inflammatory drugs (NSAIDs) in multiple myeloma. Am J Hematol 1998; 58: pp. 142-144
- [193]. Bek M.J., Wahle S., Muller B., Benzing T., Huber T.B., Kretzler M., et al: Stra13, a prostaglandin E2-induced gene, regulates the cellular redox state of podocytes. Faseb J 2003; 17: pp. 682-684
- [194]. Martineau L.C., McVeigh L.I., Jasmin B.J., and Kennedy C.R.: p38 MAP kinase mediates mechanically induced COX-2 and PG EP4 receptor expression in podocytes: implications for the actin cytoskeleton. Am J Physiol Renal Physiol 2004; 286: pp. F693-F701
- [195]. Stitt-Cavanagh E.M., Faour W.H., Takami K., Carter A., Vanderhyden B., Guan Y., et al: A maladaptive role for EP4 receptors in podocytes. J Am Soc Nephrol 2010; 21: pp. 1678-1690
- [196]. Muro S., Tanaka I., Usui T., Kotani M., Koide S., Mukoyama M., et al: Expression of prostaglandin E receptor EP4 subtype in rat adrenal zona glomerulosa: involvement in aldosterone release. Endocr J 2000; 47: pp. 429-436
- [197]. Galler M., Folkert V.W., and Schlondorff D.: Reversible acute renal insufficiency and hyperkalemia following indomethacin therapy. JAMA 1981; 246: pp. 154-155
- [198]. Miller K.P., Lazar E.J., and Fotino S.: Severe hyperkalemia during piroxicam therapy. Arch Intern Med 1984; 144: pp. 2414-2415
- [199]. Inada M., Iwasaki K., Imai C., and Hashimoto S.: Hyperpotassemia and bradycardia in a bedridden elderly woman with selective hypoaldosteronism associated with low renin activity. Intern Med 2010; 49: pp. 307-313
- [200]. Baxter G.S., Clayton J.K., Coleman R.A., Marshall K., Sangha R., and Senior J.: Characterization of the prostanoid receptors mediating constriction and relaxation of human isolated uterine artery. Br J Pharmacol 1995; 116: pp. 1692-1696
- [201]. Kabashima K., Sakata D., Nagamachi M., Miyachi Y., Inaba K., and Narumiya S.: Prostaglandin E(2)-EP4 signaling initiates skin immune responses by promoting migration and maturation of Langerhans cells. Nat Med 2003; 9: pp. 744-749
- [202]. Miyaura C., Inada M., Suzawa T., Sugimoto Y., Ushikubi F., Ichikawa A., et al: Impaired bone resorption to prostaglandin E2 in prostaglandin E receptor EP4-knockout mice. J Biol Chem 2000; 275: pp. 19819-19823
- [203]. Yoshida K., Oida H., Kobayashi T., Maruyama T., Tanaka M., Katayama T., et al: Stimulation of bone formation and prevention of bone loss by prostaglandin E EP4 receptor activation. Proc Natl Acad Sci USA 2002; 99: pp. 4580-4585
- [204]. Watanabe K.: Prostaglandin F synthase. Prostaglandins Other Lipid Mediat 2002; 68-69: pp. 401-407
- [205]. Lee S.C., and Levine L.: Purification and regulatory properties of chicken heart prostaglandin E 9-ketoreductase. J Biol Chem 1975; 250: pp. 4549-4555
- [206]. Roberts L.J., Seibert K., Liston T.E., Tantengco M.V., and Robertson R.M.: PGD2 is transformed by human coronary arteries to 9 alpha, 11 beta-PGF2, which contracts human coronary artery rings. Adv Prostaglandin Thromboxane Leukot Res 1987; 17A: pp. 427-429
- [207]. Sharif N.A., Xu S.X., Williams G.W., Crider J.Y., Griffin B.W., and Davis T.L.: Pharmacology of [3H]prostaglandin E1/[3H]prostaglandin E2 and [3H]prostaglandin F2alpha binding to EP3 and FP prostaglandin receptor binding sites in bovine corpus luteum: characterization and correlation with functional data. J Pharmacol Exp Ther 1998; 286: pp. 1094-1102
- [208]. Wallner E.I., Wada J., Tramonti G., Lin S., Srivastava S.K., and Kanwar Y.S.: Relevance of aldo-keto reductase family members to the pathobiology of diabetic nephropathy and renal development. Ren Fail 2001; 23: pp. 311-320
- [209]. Siragy H.M., Senbonmatsu T., Ichiki T., Inagami T., and Carey R.M.: Increased renal vasodilator prostanoids prevent hypertension in mice lacking the angiotensin subtype-2 receptor. J Clin Invest 1999; 104: pp. 181-188
- [210]. Siragy H.M., Inagami T., Ichiki T., and Carey R.M.: Sustained hypersensitivity to angiotensin II and its mechanism in mice lacking the subtype-2 (AT2) angiotensin receptor. Proc Natl Acad Sci USA 1999; 96: pp. 6506-6510
- [211]. Siragy H.M., and Carey R.M.: The subtype 2 angiotensin receptor regulates renal prostaglandin F2 alpha formation in conscious rats. Am J Physiol 1997; 273: pp. R1103-R1107
- [212]. Abramovitz M., Boie Y., Nguyen T., Rushmore T.H., Bayne M.A., Metters K.M., et al: Cloning and expression of a cDNA for the human prostanoid FP receptor. J Biol Chem 1994; 269: pp. 2632-2636
- [213]. Griffin B.W., Williams G.W., Crider J.Y., and Sharif N.A.: FP prostaglandin receptors mediating inositol phosphates generation and calcium mobilization in Swiss 3T3 cells: a pharmacological study. J Pharmacol Exp Ther 1997; 281: pp. 845-854
- [214]. Kelly C.R., Williams G.W., and Sharif N.A.: Real-time intracellular Ca . J Pharmacol Exp Ther 2003; 304: pp. 238-245
- [215]. Woodward D.F., and Lawrence R.A.: Identification of a single (FP) receptor associated with prostanoid-induced Ca . Biochem Pharmacol 1994; 47: pp. 1567-1574
- [216]. Sugimoto Y., Yamasaki A., Segi E., Tsuboi K., Aze Y., Nishimura T., et al: Failure of parturition in mice lacking the prostaglandin F receptor. Science 1997; 277: pp. 681-683
- [217]. Chollet A., Tos E.G., and Cirillo R.: Tocolytic effect of a selective FP receptor antagonist in rodent models reveals an innovative approach to the treatment of preterm labor. BMC Pregnancy Childbirth 2007; 7: pp. S16
- [218]. Griffin B.W., Klimko P., Crider J.Y., and Sharif N.A.: AL-8810: a novel prostaglandin F2 alpha analog with selective antagonist effects at the prostaglandin F2 alpha (FP) receptor. J Pharmacol Exp Ther 1999; 290: pp. 1278-1284
- [219]. Sharif N.A., Kelly C.R., and Crider J.Y.: Agonist activity of bimatoprost, travoprost, latanoprost, unoprostone isopropyl ester and other prostaglandin analogs at the cloned human ciliary body FP prostaglandin receptor. J Ocul Pharmacol Ther 2002; 18: pp. 313-324
- [220]. Saito O., Guan Y., Qi Z., Davis L.S., Komhoff M., Sugimoto Y., et al: Expression of the prostaglandin F receptor (FP) gene along the mouse genitourinary tract. Am J Physiol Renal Physiol 2003; 284: pp. F1164-F1170
- [221]. Yu Y., Lucitt M.B., Stubbe J., Cheng Y., Friis U.G., Hansen P.B., et al: Prostaglandin F2alpha elevates blood pressure and promotes atherosclerosis. Proc Natl Acad Sci USA 2009; 106: pp. 7985-7990
- [222]. Urade Y., and Eguchi N.: Lipocalin-type and hematopoietic prostaglandin D synthases as a novel example of functional convergence. Prostaglandins & Other Lipid Mediators 2002; 68-69: pp. 375-382
- [223]. Shimura C., Satoh T., Igawa K., Aritake K., Urade Y., Nakamura M., et al: Dendritic cells express hematopoietic prostaglandin D synthase and function as a source of prostaglandin D2 in the skin. Am J Pathol 2010; 176: pp. 227-237
- [224]. Vitzthum H., Abt I., Einhellig S., and Kurtz A.: Gene expression of prostanoid forming enzymes along the rat nephron. Kidney Int 2002; 62: pp. 1570-1581
- [225]. Qu W.M., Huang Z.L., Xu X.H., Aritake K., Eguchi N., Nambu F., et al: Lipocalin-type prostaglandin D synthase produces prostaglandin D2 involved in regulation of physiological sleep. Proc Natl Acad Sci USA 2006; 103: pp. 17949-17954
- [226]. Eguchi N., Minami T., Shirafuji N., Kanaoka Y., Tanaka T., Nagata A., et al: Lack of tactile pain (allodynia) in lipocalin-type prostaglandin D synthase-deficient mice. Proc Natl Acad Sci USA 1999; 96: pp. 726-730
- [227]. Nakayama H., Echizen H., Gomi T., Shibuya Y., Nakamura Y., Nakano K., et al: Urinary lipocalin-type prostaglandin D synthase: a potential marker for early gentamicin-induced renal damage? Ther Drug Monit 2009; 31: pp. 126-130
- [228]. Uehara Y., Makino H., Seiki K., and Urade Y.: Urinary excretions of lipocalin-type prostaglandin D synthase predict renal injury in type-2 diabetes: a cross-sectional and prospective multicentre study. Nephrol Dial Transplant 2009; 24: pp. 475-482
- [229]. Ogawa M., Hirawa N., Tsuchida T., Eguchi N., Kawabata Y., Numabe A., et al: Urinary excretions of lipocalin-type prostaglandin D2 synthase predict the development of proteinuria and renal injury in OLETF rats. Nephrol Dial Transplant 2006; 21: pp. 924-934
- [230]. Tsuchida T., Eguchi N., Eguchi Y., Numabe A., Nakajima H., Oda H., et al: Lipocalin-type prostaglandin D synthase in urine in adriamycin-induced nephropathy of mice. Nephron Physiol 2004; 96: pp. p42-p51
- [231]. Ragolia L., Palaia T., Hall C.E., Maesaka J.K., Eguchi N., and Urade Y.: Accelerated glucose intolerance, nephropathy, and atherosclerosis in prostaglandin D2 synthase knock-out mice. J Biol Chem 2005; 280: pp. 29946-29955
- [232]. Kanaoka Y., Ago H., Inagaki E., Nanayama T., Miyano M., Kikuno R., et al: Cloning and crystal structure of hematopoietic prostaglandin D synthase. Cell 1997; 90: pp. 1085-1095
- [233]. Kanaoka Y., and Urade Y.: Hematopoietic prostaglandin D synthase. Prostaglandins Leukot Essent Fatty Acids 2003; 69: pp. 163-167
- [234]. Boie Y., Sawyer N., Slipetz D.M., Metters K.M., and Abramovitz M.: Molecular cloning and characterization of the human prostanoid DP receptor. J Biol Chem 1995; 270: pp. 18910-18916
- [235]. Wright D.H., Nantel F., Metters K.M., and Ford-Hutchinson A.W.: A novel biological role for prostaglandin D2 is suggested by distribution studies of the rat DP prostanoid receptor. Eur J Pharmacol 1999; 377: pp. 101-115
- [236]. Hirai H., Tanaka K., Yoshie O., Ogawa K., Kenmotsu K., Takamori Y., et al: Prostaglandin D2 selectively induces chemotaxis in T helper type 2 cells, eosinophils, and basophils via seven-transmembrane receptor CRTH2. J Exp Med 2001; 193: pp. 255-262
- [237]. Toh H., Ichikawa A., and Narumiya S.: Molecular evolution of receptors for eicosanoids. FEBS Letters 1995; 361: pp. 17-21
- [238]. Kabashima K., and Narumiya S.: The DP receptor, allergic inflammation and asthma. Prostaglandins Leukot Essent Fatty Acids 2003; 69: pp. 187-194
- [239]. Koch K.A., Wessale J.L., Moreland R., Reinhart G.A., and Cox B.F.: Effects of BW245C, a prostaglandin dp receptor agonist, on systemic and regional haemodynamics in the anaesthetized rat. Clin Exp Pharmacol Physiol 2005; 32: pp. 931-935
- [240]. Hoeper M.M., Schwarze M., Ehlerding S., Adler-Schuermeyer A., Spiekerkoetter E., Niedermeyer J., et al: Long-term treatment of primary pulmonary hypertension with aerosolized iloprost, a prostacyclin analogue. N Engl J Med 2000; 342: pp. 1866-1870
- [241]. Yokoyama C., Yabuki T., Inoue H., Tone Y., Hara S., Hatae T., et al: Human gene encoding prostacyclin synthase (PTGIS): Genomic organization, chromosomal localization, and promoter activity. Genomics 1996; 36: pp. 296-304
- [242]. Tone Y., Inoue H., Hara S., Yokoyama C., Hatae T., Oida H., et al: The regional distribution and cellular localization of mRNA encoding rat prostacyclin synthase. Eur J Cell Biol 1997; 72: pp. 268-277
- [243]. Yokoyama C., Yabuki T., Shimonishi M., Wada M., Hatae T., Ohkawara S., et al: Prostacyclin-deficient mice develop ischemic renal disorders, including nephrosclerosis and renal infarction. Circulation 2002; 106: pp. 2397-2403
- [244]. Murata T., Ushikubi F., Matsuoka T., Hirata M., Yamasaki A., Sugimoto Y., et al: Altered pain perception and inflammatory response in mice lacking prostacyclin receptor. Nature 1997; 388: pp. 678-682
- [245]. Hao C.M., Redha R., Morrow J., and Breyer M.D.: Peroxisome proliferator-activated receptor delta activation promotes cell survival following hypertonic stress. J Biol Chem 2002; 277: pp. 21341-21345
- [246]. Lim H., Gupta R.A., Ma W.G., Paria B.C., Moller D.E., Morrow J.D., et al: Cyclo-oxygenase-2-derived prostacyclin mediates embryo implantation in the mouse via PPARdelta. Genes Dev 1999; 13: pp. 1561-1574
- [247]. Namba T., Oida H., Sugimoto Y., Kakizuka A., Negishi M., Ichikawa A., et al: cDNA cloning of a mouse prostacyclin receptor: multiple signaling pathways and expression in thymic medulla. J Biol Chem 1994; 269: pp. 9986-9992
- [248]. Oida H., Namba T., Sugimoto Y., Ushikubi F., Ohishi H., Ichikawa A., et al: In situ hybridization studies on prostacyclin receptor mRNA expression in various mouse organs. Br J Pharmacol 1995; 116: pp. 2828-2837
- [249]. Fujino T., Nakagawa N., Yuhki K., Hara A., Yamada T., Takayama K., et al: Decreased susceptibility to renovascular hypertension in mice lacking the prostaglandin I2 receptor IP. J Clin Invest 2004; 114: pp. 805-812
- [250]. Egan K.M., Lawson J.A., Fries S., Koller B., Rader D.J., Smyth E.M., et al: COX-2-derived prostacyclin confers atheroprotection on female mice. Science 2004; 306: pp. 1954-1957
- [251]. Chevalier D., Lo-Guidice J.M., Sergent E., Allorge D., Debuysere H., Ferrari N., et al: Identification of genetic variants in the human thromboxane synthase gene (CYP5A1). Mutat Res 2001; 432: pp. 61-67
- [252]. Miyata A., Yokoyama C., Ihara H., Bandoh S., Takeda O., Takahashi E., et al: Characterization of the human gene (TBXAS1) encoding thromboxane synthase. Eur J Biochem 1994; 224: pp. 273-279
- [253]. Zhang L.Q., Chase M.B., and Shen R.F.: Molecular cloning and expression of murine thromboxane synthase. Biochem Biophys Res Commun 1993; 194: pp. 741-748
- [254]. Nusing R., Fehr P.M., Gudat F., Kemeny E., Mihatsch M.J., and Ullrich V.: The localization of thromboxane synthase in normal and pathological human kidney tissue using a monoclonal antibody Tu 300. Virchows Arch 1994; 424: pp. 69-74
- [255]. Wilcox C.S., and Welch W.J.: Thromboxane synthase and TP receptor mRNA in rat kidney and brain: effects of salt intake and ANG II. Am J Physiol Renal Physiol 2003; 284: pp. F525-F531
- [256]. Quest D.W., and Wilson T.W.: Effects of ridogrel, a thromboxane synthase inhibitor and receptor antagonist, on blood pressure in the spontaneously hypertensive rat. Jpn J Pharmacol 1998; 78: pp. 479-486
- [257]. Vezza R., Mezzasoma A.M., Venditti G., and Gresele P.: Prostaglandin endoperoxides and thromboxane A2 activate the same receptor isoforms in human platelets. Thromb Haemost 2002; 87: pp. 114-121
- [258]. Hirata M., Hayashi Y., Ushikubi F., Yokota Y., Kageyama R., Nakanishi S., et al: Cloning and expression of cDNA for a human thromboxane A2 receptor. Nature 1991; 349: pp. 617-620
- [259]. Abe T., Takeuchi K., Takahashi N., Tsutsumi E., Taniyama Y., and Abe K.: Rat kidney thromboxane receptor: molecular cloning, signal transduction, and intrarenal expression localization. J Clin Invest 1995; 96: pp. 657-664
- [260]. Francois H., Athirakul K., Mao L., Rockman H., and Coffman T.M.: Role for thromboxane receptors in angiotensin-II-induced hypertension. Hypertension 2004; 43: pp. 364-369
- [261]. Boffa J.J., Just A., Coffman T.M., and Arendshorst W.J.: Thromboxane receptor mediates renal vasoconstriction and contributes to acute renal failure in endotoxemic mice. J Am Soc Nephrol 2004; 15: pp. 2358-2365
- [262]. Schnermann J., Traynor T., Pohl H., Thomas D.W., Coffman T.M., and Briggs J.P.: Vasoconstrictor responses in thromboxane receptor knockout mice: Tubuloglomerular feedback and ureteral obstruction. Acta Physiol Scand 2000; 168: pp. 201-207
- [263]. Snoeijs M.G., Hoogland P.R., Boonen B., Coffman T.M., Peutz-Kootstra C.J., Buurman W.A., et al: Thromboxane receptor signalling in renal ischemia reperfusion injury. Free Radic Res 2011; 45: pp. 699-706
- [264]. Schuster V.L.: Prostaglandin transport. Prostaglandins Other Lipid Mediat 2002; 68-69: pp. 633-647
- [265]. Kanai N., Lu R., Satriano J.A., Bao Y., Wolkoff A.W., and Schuster V.L.: Identification and characterization of a prostaglandin transporter. Science 1995; 268: pp. 866-869
- [266]. Chang H.Y., Locker J., Lu R., and Schuster V.L.: Failure of postnatal ductus arteriosus closure in prostaglandin transporter-deficient mice. Circulation 2010; 121: pp. 529-536
- [267]. Bao Y., Pucci M.L., Chan B.S., Lu R., Ito S., and Schuster V.L.: Prostaglandin transporter PGT is expressed in cell types that synthesize and release prostanoids. Am J Physiol Renal Physiol 2002; 282: pp. F1103-F1110
- [268]. Reid G., Wielinga P., Zelcer N., van der Heijden I., Kuil A., de Haas M., et al: The human multidrug resistance protein MRP4 functions as a prostaglandin efflux transporter and is inhibited by nonsteroidal antiinflammatory drugs. Proceedings of the National Academy of Sciences 2003; 100: pp. 9244-9249
- [269]. Lin Z.P., Zhu Y. -L., Johnson D.R., Rice K.P., Nottoli T., Hains B.C., et al: Disruption of cAMP and prostaglandin E2 transport by multidrug resistance protein 4 deficiency alters cAMP-mediated signaling and nociceptive response. Mol Pharmacol 2008; 73: pp. 243-251
- [270]. Tomasoni S., Zappella S., Gotti E., Casiraghi F., Bonazzola S., Benigni A., et al: Upregulation of renal and systemic cyclooxygenase-2 in patients with active lupus nephritis. J Am Soc Nephrol 1998; 9: pp. 1202-1212
- [271]. Schneider A., Harendza S., Zahner G., Jocks T., Wenzel U., Wolf G., et al: Cyclooxygenase metabolites mediate glomerular monocyte chemoattractant protein-1 formation and monocyte recruitment in experimental glomerulonephritis [see comments]. Kidney Int 1999; 55: pp. 430-441
- [272]. Zoja C., Benigni A., Verroust P., Ronco P., Bertani T., and Remuzzi G.: Indomethacin reduces proteinuria in passive Heymann nephritis in rats. Kidney Int 1987; 31: pp. 1335-1343
- [273]. Takano T., Cybulsky A.V., Cupples W.A., Ajikobi D.O., Papillon J., and Aoudjit L.: Inhibition of cyclooxygenases reduces complement-induced glomerular epithelial cell injury and proteinuria in passive Heymann nephritis. J Pharmacol Exp Ther 2003; 305: pp. 240-249
- [274]. Kitahara M., Eitner F., Ostendorf T., Kunter U., Janssen U., Westenfeld R., et al: Selective cyclooxygenase-2 inhibition impairs glomerular capillary healing in experimental glomerulonephritis. J Am Soc Nephrol 2002; 13: pp. 1261-1270
- [275]. Anderson G.D., Hauser S.D., McGarity K.L., Bremer M.E., Isakson P.C., and Gregory S.A.: Selective inhibition of cyclooxygenase (COX)-2 reverses inflammation and expression of COX-2 and interleukin 6 in rat adjuvant arthritis. J Clin Invest 1996; 97: pp. 2672-2679
- [276]. Simon L.S.: Role and regulation of cyclooxygenase-2 during inflammation. Am J Med 1999; 106: pp. 37S-42S
- [277]. Mauer S.M., Steffes M.W., Ellis E.N., Sutherland D.E., Brown D.M., and Goetz F.C.: Structural–functional relationships in diabetic nephropathy. J Clin Invest 1984; 74: pp. 1143-1155
- [278]. Najafian B., Alpers C.E., and Fogo A.B.: Pathology of human diabetic nephropathy. Contrib Nephrol 2011; 170: pp. 36-47
- [279]. Ciavarella A., Galuppi V., Forlani G., and Vannini P.: The prevalence of glomerular hyperfiltration in type 1 (insulin-dependent) diabetes mellitus. Diabete Metab 1988; 14: pp. 73-74
- [280]. Levine D.Z., Iacovitti M., Robertson S.J., and Mokhtar G.A.: Modulation of single-nephron GFR in the db/db mouse model of type 2 diabetes mellitus. Am J Physiol Regul Integr Comp Physiol 2006; 290: pp. R975-R981
- [281]. Qi Z., Fujita H., Jin J., Davis L.S., Wang Y., Fogo A.B., et al: Characterization of susceptibility of inbred mouse strains to diabetic nephropathy. Diabetes 2005; 54: pp. 2628-2637
- [282]. Viberti G.C., Benigni A., Bognetti E., Remuzzi G., and Wiseman M.J.: Glomerular hyperfiltration and urinary prostaglandins in type 1 diabetes mellitus. Diabet Med 1989; 6: pp. 219-223
- [283]. Craven P.A., Caines M.A., and DeRubertis F.R.: Sequential alterations in glomerular prostaglandin and thromboxane synthesis in diabetic rats: relationship to the hyperfiltration of early diabetes. Metabolism 1987; 36: pp. 95-103
- [284]. Mathiesen E.R., Hommel E., Olsen U.B., and Parving H.H.: Elevated urinary prostaglandin excretion and the effect of indomethacin on renal function in incipient diabetic nephropathy. Diabet Med 1988; 5: pp. 145-149
- [285]. Moel D.I., Safirstein R.L., McEvoy R.C., and Hsueh W.: Effect of aspirin on experimental diabetic nephropathy. J Lab Clin Med 1987; 110: pp. 300-307
- [286]. Cheng H.F., Wang C.J., Moeckel G.W., Zhang M.Z., McKanna J.A., and Harris R.C.: Cyclooxygenase-2 inhibitor blocks expression of mediators of renal injury in a model of diabetes and hypertension. Kidney Int 2002; 62: pp. 929-939
- [287]. Khan K.N., Stanfield K.M., Harris R.K., and Baron D.A.: Expression of cyclooxygenase-2 in the macula densa of human kidney in hypertension, congestive heart failure, and diabetic nephropathy. Ren Fail 2001; 23: pp. 321-330
- [288]. Komers R., Lindsley J.N., Oyama T.T., Schutzer W.E., Reed J.F., Mader S.L., et al: Immunohistochemical and functional correlations of renal cyclooxygenase-2 in experimental diabetes. J Clin Invest 2001; 107: pp. 889-898
- [289]. Xu S., Jiang B., Maitland K.A., Bayat H., Gu J., Nadler J.L., et al: The thromboxane receptor antagonist S18886 attenuates renal oxidant stress and proteinuria in diabetic apolipoprotein E-deficient mice. Diabetes 2006; 55: pp. 110-119
- [290]. Pelayo J.C., and Shanley P.F.: Glomerular and tubular adaptive responses to acute nephron loss in the rat. Effect of prostaglandin synthesis inhibition. J Clin Invest 1990; 85: pp. 1761-1769
- [291]. Wang J.L., Cheng H.F., Shappell S., and Harris R.C.: A selective cyclooxygenase-2 inhibitor decreases proteinuria and retards progressive renal injury in rats. Kidney Int 2000; 57: pp. 2334-2342
- [292]. Cheng H., Zhang M., Moeckel G.W., Zhao Y., Wang S., Qi Z., et al: Expression of mediators of renal injury in the remnant kidney of ROP mice is attenuated by cyclooxygenase-2 inhibition. Nephron Exp Nephrol 2005; 101: pp. e75-e85
- [293]. Funk C.D., Chen X. -S., Johnson E.N., and Zhao L.: Lipoxygenase genes and their targeted disruption. Prostaglandins & Other Lipid Mediators 2002; 68-69: pp. 303-312
- [294]. Brash A.R.: Lipoxygenases: occurrence, functions, catalysis, and acquisition of substrate. J Biol Chem 1999; 274: pp. 23679-23682
- [295]. Duroudier N.P., Tulah A.S., and Sayers I.: Leukotriene pathway genetics and pharmacogenetics in allergy. Allergy 2009; 64: pp. 823-839
- [296]. Peters-Golden M., and Henderson W.R.: Leukotrienes. N Engl J Med 2007; 357: pp. 1841-1854
- [297]. Rinaldo-Matthis A., and Haeggstrom J.Z.: Structures and mechanisms of enzymes in the leukotriene cascade. Biochimie 2010; 92: pp. 676-681
- [298]. Jawien J., Gajda M., Rudling M., Mateuszuk L., Olszanecki R., Guzik T.J., et al: Inhibition of five lipoxygenase activating protein (FLAP) by MK-886 decreases atherosclerosis in apoE/LDLR-double knockout mice. Eur J Clin Invest 2006; 36: pp. 141-146
- [299]. Samuelsson B., and Funk C.D.: Enzymes involved in the biosynthesis of leukotriene B4. J Biol Chem 1989; 264: pp. 19469-19472
- [300]. Shimizu T., Yokomizo T., and Izumi T.: Leukotriene-B4 receptor and signal transduction. Ernst Schering Res Found Workshop 2000; undefined: pp. 125-141
- [301]. Kolaczkowska E., Shahzidi S., Seljelid R., van Rooijen N., and Plytycz B.: Early vascular permeability in murine experimental peritonitis is co-mediated by resident peritoneal macrophages and mast cells: crucial involvement of macrophage-derived cysteinyl-leukotrienes. Inflammation 2002; 26: pp. 61-71
- [302]. Porreca E., Di Febbo C., Di Sciullo A., Angelucci D., Nasuti M., Vitullo P., et al: Cysteinyl leukotriene D4 induced vascular smooth muscle cell proliferation: a possible role in myointimal hyperplasia. Thromb Haemost 1996; 76: pp. 99-104
- [303]. Farrukh I.S., Sciuto A.M., Spannhake E.W., Gurtner G.H., and Michael J.R.: Leukotriene D4 increases pulmonary vascular permeability and pressure by different mechanisms in the rabbit. Am Rev Respir Dis 1986; 134: pp. 229-232
- [304. Fiedler V.B., Mardin M., and Abram T.S.: Leukotriene D4-induced vasoconstriction of coronary arteries in anaesthetized dogs. Eur Heart J 1984; 5: pp. 253-260
- [305]. Collin M., Rossi A., Cuzzocrea S., Patel N.S., Di Paola R., Hadley J., et al: Reduction of the multiple organ injury and dysfunction caused by endotoxemia in 5-lipoxygenase knockout mice and by the 5-lipoxygenase inhibitor zileuton. J Leukoc Biol 2004; 76: pp. 961-970
- [306]. Patel N.S., Cuzzocrea S., Chatterjee P.K., Di Paola R., Sautebin L., Britti D., et al: Reduction of renal ischemia-reperfusion injury in 5-lipoxygenase knockout mice and by the 5-lipoxygenase inhibitor zileuton. Mol Pharmacol 2004; 66: pp. 220-227
- [307]. Kitagawa K., Matsumoto M., and Hori M.: Cerebral ischemia in 5-lipoxygenase knockout mice. Brain Res 2004; 1004: pp. 198-202
- [308]. Di Gennaro A., Wågsäter D., Mäyränpää M.I., Gabrielsen A., Swedenborg J., Hamsten A., et al: Increased expression of leukotriene C4 synthase and predominant formation of cysteinyl-leukotrienes in human abdominal aortic aneurysm. Proc Natl Acad Sci 2010; 107: pp. 21093-21097
- [309]. Ardaillou R., Baud L., and Sraer J.: Leukotrienes and other lipoxygenase products of arachidonic acid synthesized in the kidney. Am J Med 1986; 81: pp. 12-22
- [310]. Badr K.F.: 15-Lipoxygenase products as leukotriene antagonists: Therapeutic potential in glomerulonephritis. Kidney Int Suppl 1992; 38: pp. S101-S108
- [311]. Kawasaki Y., Tanji M., Takano K., Fukuda Y., Isome M., Nozawa R., et al: The leukotriene B4 receptor antagonist ONO-4057 inhibits mesangioproliferative changes in anti-Thy-1 nephritis. Nephrology Dialysis Transplantation 2005; 20: pp. 2697-2703
- [312]. Reinhold S.W., Vitzthum H., Filbeck T., Wolf K., Lattas C., Riegger G.A., et al: Gene expression of 5-, 12-, and 15-lipoxygenases and leukotriene receptors along the rat nephron. Am J Physiol Renal Physiol 2006; 290: pp. F864-F872
- [313]. Menegatti E., Roccatello D., Fadden K., Piccoli G., De Rosa G., Sena L.M., et al: Gene expression of 5-lipoxygenase and LTA4 hydrolase in renal tissue of nephrotic syndrome patients. Clin Exp Immunol 1999; 116: pp. 347-353
- [314]. Badr K.F.: Five-lipoxygenase products in glomerular immune injury. J Am Soc Nephrol 1992; 3: pp. 907-915
- [315]. Katoh T., Lianos E.A., Fukunaga M., Takahashi K., and Badr K.F.: Leukotriene D4 is a mediator of proteinuria and glomerular hemodynamic abnormalities in passive Heymann nephritis. J Clin Invest 1993; 91: pp. 1507-1515
- [316]. Natarajan R., and Nadler J.L.: Lipid inflammatory mediators in diabetic vascular disease. Arterioscler Thromb Vasc Biol 2004; 24: pp. 1542-1548
- [317]. Dailey L.A., and Imming P.: 12-Lipoxygenase: classification, possible therapeutic benefits from inhibition, and inhibitors. Curr Med Chem 1999; 6: pp. 389-398
- [318]. Zhao L., Moos M.P., Grabner R., Pedrono F., Fan J., Kaiser B., et al: The 5-lipoxygenase pathway promotes pathogenesis of hyperlipidemia-dependent aortic aneurysm. Nat Med 2004; 10: pp. 966-973
- [319]. Klein R.F., Allard J., Avnur Z., Nikolcheva T., Rotstein D., Carlos A.S., et al: Regulation of bone mass in mice by the lipoxygenase gene Alox15. Science 2004; 303: pp. 229-232
- [320]. Poeckel D., and Funk C.D.: The 5-lipoxygenase/leukotriene pathway in preclinical models of cardiovascular disease. Cardiovasc Res 2010; 86: pp. 243-253
- [321]. Kang S.W., Natarajan R., Shahed A., Nast C.C., LaPage J., Mundel P., et al: Role of 12-lipoxygenase in the stimulation of p38 mitogen-activated protein kinase and collagen alpha5(IV) in experimental diabetic nephropathy and in glucose-stimulated podocytes. J Am Soc Nephrol 2003; 14: pp. 3178-3187
- [322]. Kim Y.S., Xu Z.G., Reddy M.A., Li S.L., Lanting L., Sharma K., et al: Novel interactions between TGF-{beta}1 actions and the 12/15-lipoxygenase pathway in mesangial cells. J Am Soc Nephrol 2005; 16: pp. 352-362
- [323]. Xu Z.G., Li S.L., Lanting L., Kim Y.S., Shanmugam N., Reddy M.A., et al: Relationship between 12/15-lipoxygenase and COX-2 in mesangial cells: potential role in diabetic nephropathy. Kidney Int 2006; 69: pp. 512-519
- [324]. Gonzalez-Nunez D., Sole M., Natarajan R., and Poch E.: 12-Lipoxygenase metabolism in mouse distal convoluted tubule cells. Kidney Int 2005; 67: pp. 178-186
- [325]. Kang S.W., Adler S.G., Nast C.C., LaPage J., Gu J.L., Nadler J.L., et al: 12-lipoxygenase is increased in glucose-stimulated mesangial cells and in experimental diabetic nephropathy. Kidney Int 2001; 59: pp. 1354-1362
- [326]. Guo Q.Y., Miao L.N., Li B., Ma F.Z., Liu N., Cai L., et al: Role of 12-lipoxygenase in decreasing P-cadherin and increasing angiotensin II type 1 receptor expression according to glomerular size in type 2 diabetic rats. Am J Physiol Endocrinol Metab 2011; 300: pp. E708-E716
- [327]. Xu Z.G., Miao L.N., Cui Y.C., Jia Y., Yuan H., and Wu M.: Angiotensin II type 1 receptor expression is increased via 12-lipoxygenase in high glucose-stimulated glomerular cells and type 2 diabetic glomeruli. Nephrol Dial Transplant 2009; 24: pp. 1744-1752
- [328]. Capdevila J.H., Harris R.C., and Falck J.R.: Microsomal cytochrome P450 and eicosanoid metabolism. Cell Mol Life Sci 2002; 59: pp. 780-789
- [329]. McGiff J.C.: Cytochrome P-450 metabolism of arachidonic acid. Annu Rev Pharmacol Toxicol 1991; 31: pp. 339-369
- [330]. Camara N.O., Martins J.O., Landgraf R.G., and Jancar S.: Emerging roles for eicosanoids in renal diseases. Curr Opin Nephrol Hypertens 2009; 18: pp. 21-27
- [331]. Spector A.A.: Arachidonic acid cytochrome P450 epoxygenase pathway. J Lipid Res 2009; 50: pp. S52-S56
- [332]. Kroetz D.L., and Zeldin D.C.: Cytochrome P450 pathways of arachidonic acid metabolism. Curr Opin Lipidol 2002; 13: pp. 273-283
- [333]. Scarborough P.E., Ma J., Qu W., and Zeldin D.C.: P450 subfamily CYP2J and their role in the bioactivation of arachidonic acid in extrahepatic tissues. Drug Metab Rev 1999; 31: pp. 205-234
- [334]. Lasker J.M., Chen W.B., Wolf I., Bloswick B.P., Wilson P.D., and Powell P.K.: Formation of 20-hydroxyeicosatetraenoic acid, a vasoactive and natriuretic eicosanoid, in human kidney. Role of Cyp4F2 and Cyp4A11. J Biol Chem 2000; 275: pp. 4118-4126
- [335]. Roman R.J.: P-450 Metabolites of arachidonic acid in the control of cardiovascular function. Physiol Rev 2002; 82: pp. 131-185
- [336]. Imig J., Gebremedhin D., Zou A., Stec D., Harder D., Falck J., et al: Formation and actions of 20-hydroxyeicosatetraenoic acid in the renal microcirculation. Am J Physiol 1996; 270: pp. R217-R227
- [337]. Larsen B.T., Gutterman D.D., and Hatoum O.A.: Emerging role of epoxyeicosatrienoic acids in coronary vascular function. Eur J Clin Invest 2006; 36: pp. 293-300
- [338]. Oltman C.L., Weintraub N.L., VanRollins M., and Dellsperger K.C.: Epoxyeicosatrienoic acids and dihydroxyeicosatrienoic acids are potent vasodilators in the canine coronary microcirculation. Circ Res 1998; 83: pp. 932-939
- [339]. Wei Y., Lin D.H., Kemp R., Yaddanapudi G.S., Nasjletti A., Falck J.R., et al: Arachidonic acid inhibits epithelial Na channel via cytochrome P450 (CYP) epoxygenase-dependent metabolic pathways. J Gen Physiol 2004; 124: pp. 719-727
- [340]. Wang S., Meng F., Xu J., and Gu Y.: Effects of lipids on ENaC activity in cultured mouse cortical collecting duct cells. J Membr Biol 2009; 227: pp. 77-85
- [341]. Sun P., Lin D.H., Yue P., Jiang H., Gotlinger K.H., Schwartzman M.L., et al: High potassium intake enhances the inhibitory effect of 11,12-EET on ENaC. J Am Soc Nephrol 2010; 21: pp. 1667-1677
- [342]. Athirakul K., Bradbury J.A., Graves J.P., DeGraff L.M., Ma J., Zhao Y., et al: Increased blood pressure in mice lacking cytochrome P450 2J5. FASEB J 2008; 22: pp. 4096-4108
- [343]. Holla V.R., Adas F., Imig J.D., Zhao X., Price E., Olsen N., et al: Alterations in the regulation of androgen-sensitive Cyp 4a monooxygenases cause hypertension. Proc Natl Acad Sci USA 2001; 98: pp. 5211-5216
- [344]. Nakagawa K., Holla V.R., Wei Y., Wang W.H., Gatica A., Wei S., et al: Salt-sensitive hypertension is associated with dysfunctional Cyp4a10 gene and kidney epithelial sodium channel. J Clin Invest 2006; 116: pp. 1696-1702
- [345]. Miyazono M., Zhu D., Nemenoff R., Jacobs E.R., and Carter E.P.: Increased epoxyeicosatrienoic acid formation in the rat kidney during liver cirrhosis. J Am Soc Nephrol 2003; 14: pp. 1766-1775
- [346]. Hercule H., and Oyekan A.: Renal cytochrome p450 oxygenases and preglomerular vascular response to arachidonic acid and endothelin-1 following ischemia/reperfusion. J Pharmacol Exp Ther 2002; 302: pp. 717-724
- [347]. McCarthy E.T., Sharma R., and Sharma M.: Protective effect of 20-hydroxyeicosatetraenoic acid (20-HETE) on glomerular protein permeability barrier. Kidney Int 2005; 67: pp. 152-156
- [348]. Yuhki K. -I., Kojima F., Kashiwagi H., Kawabe J. -I., Fujino T., Narumiya S., et al: Roles of prostanoids in the pathogenesis of cardiovascular diseases: novel insights from knockout mouse studies. Pharm Therap 2011; 129: pp. 195-205
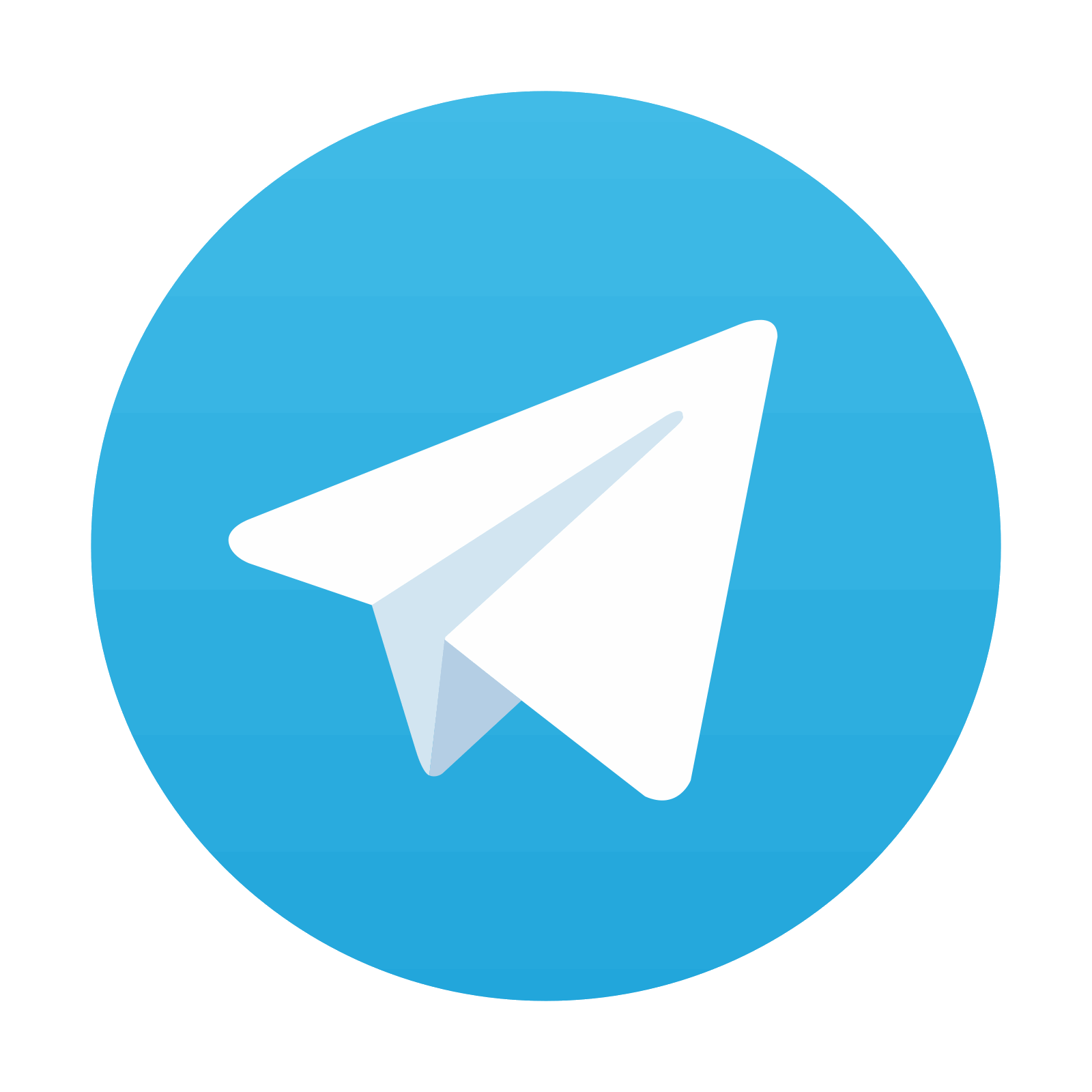
Stay updated, free articles. Join our Telegram channel
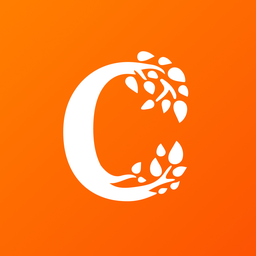
Full access? Get Clinical Tree
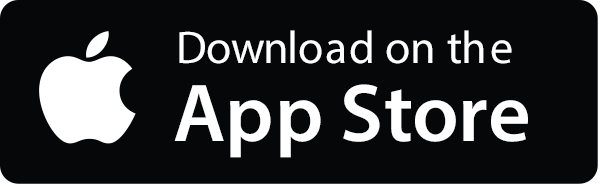
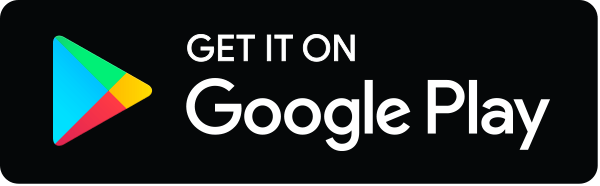