CHAPTER
11
Disorders of Phosphate Homeostasis—Hypo and Hyperphosphatemia
Recommended Time to Complete: 1 Day
REGULATION
Phosphorus is an important element involved in various vital functions of the body including signal transduction, cell membrane function, and energy exchange. Phosphorus circulates in the bloodstream in 2 forms: an organic fraction made up primarily of phospholipids; and an inorganic fraction. Of these 2 fractions it is the inorganic fraction, which makes up approximately one-third of total plasma phosphorus, that is assayed in the clinical laboratory. The majority (75%) of inorganic phosphorus is free in solution and exists as either divalent (HPO42) or monovalent () phosphate. The relative amounts of each ion depend on the systemic pH. At pH 7.4, 80% is in the divalent form based on the dissociation constant of the reaction. Approximately 1% of body weight in a 70-kg human is phosphorus (600 to 700 g). It is distributed primarily in skeleton (80% to 85%), other organs (14% in skeletal muscle and viscera), 1% in blood and extravascular space, and a small fraction is found in the intracellular compartment where the phosphorus pool is inorganic and available for adenosine triphosphate (ATP) synthesis (Figure 11.1). Fifteen percent of phosphorus is protein bound. A typical Western diet includes 800 to 1500 mg of phosphorus daily. Diurnal variation occurs in normal individuals, with the lowest serum phosphorus concentration in the morning and a gradual rise during the day to peak in the evening. The variability in serum phosphorus concentration may be as high as 1 mg/dL and does not correlate with abundance of renal transporter proteins. This diurnal variation is intact in some patients with hyperparathyroidism but remains unknown in patients with hypophosphatemia from other causes. However, we measure random serum inorganic phosphorus concentration in the clinical setting, the normal range of which is 2.5 to 4.5 mg/dL (0.8 to 1.5 mM). Three major organs work in concert to maintain phosphorus balance: intestinal uptake; retention or release from bone; and regulated renal reabsorption.
FIGURE 11-1. Phosphorus homeostasis. Daily phosphorus fluxes between extracellular fluid (ECF), intestine, kidney, and bone are shown. In the steady state, net intestinal absorption and renal excretion are equal. The majority of phosphorus in the body is in bone. (With permission from Schrier, R.W. (ed.). Manual of Nephrology. Lippincott Williams & Wilkins, Philadelphia, PA, 2000.)
Inorganic phosphate is absorbed along the entire length of small intestine via passive and active transport. The role of colon, at physiologic levels, is insignificant. However, in certain conditions, the colon plays a role in hypophosphatemia as the unregulated secretion (100 to 200 mg/day) is exaggerated with diarrhea. Overall, the small intestine plays a more active role in phosphorus balance than previously thought mediated by a high-affinity Pi transporter, Npt2b (type II sodium-dependent phosphate transporter). Found in the brush border membrane (BBM) of small intestine, Npt2b transports divalent Pi with a stoichiometry of 2:1 across the BBM in an electroneutral fashion. Npt2b activity is regulated by dietary inorganic phosphate (Pi) intake and serum 1,25-dihydroxy-vitamin D3 (1,25[OH]2D3) concentration. On the one hand, low dietary Pi enhances Npt2b activity through a posttranscriptional mechanism, and on the other hand, 1,25[OH]2D3 enhances transporter activity by a transcription-dependent pathway mediated by the vitamin D receptor (VDR). Interestingly, parathyroid hormone (PTH) has no effect on Npt2b. Furthermore, a regulated organ crosstalk between small intestine, kidney, and bone exists, as shown in Npt2b knockout (KO) animal models, where decline in fibroblast growth factor 23 (FGF-23) and elevation in 1,25[OH]2D3 occurs to maintain serum phosphorus and calcium concentration in these animals with upregulation of Npt2a in kidney. Paracellular Pi transport in intestine is not clearly understood.
The kidney plays a key role in Pi homeostasis. It filters 200 mmol (~20 g) of Pi per day. Pi is freely filtered, as only 15% is protein bound, and the majority (85% to 90%) is reabsorbed. The latter occurs primarily in the proximal convoluted tubule (PCT) via sodium-dependent phosphate transporters (70% by Npt2a and ~30% by Npt2c, minimal by PiT-2). Reabsorption saturates and excretion increases in proportion to the filtered load at a serum phosphorus concentration within the normal range. Although, basolateral Pi transport remains poorly understood, with possible sodium independent or concentration dependent pathways, there have been recent advances in the understanding of renal Pi transport across the apical membrane.
Three types of sodium-phosphate cotransporters are expressed in kidney (Npt1, Npt2, and Npt3). Npt1 is a nonspecific anionic carrier and its physiologic role is unknown. It may play a role in urate transport. Npt2 is further subdivided into 3 isoforms: a, b, and c. Npt2b is found in the small intestine and lung. Human mutations in Npt2b present with lung calcification and normal serum calcium and Pi concentrations. Npt2a and Npt2c are found in the PCT. PiT-1 and PiT-2 belong to the Npt3 family. PiT-1 and -2 are widely expressed and likely help supply Pi to cells rather than controlling Pi balance. Table 11.1 illustrates the properties of Npt transporter isoforms. Overall, renal phosphate transport maximum (Tm) is regulated by a variety of stimuli.
TABLE 11-1. Sodium Phosphate Cotransporter Isoforms
PTH and phosphatonins lower the Tm. PTH induces the removal of Npt2a from the apical surface of PCT via PTH1 receptors expressed on the apical and basolateral membranes. FGF-23 and Klotho are not necessary for the phosphaturic action of PTH. It is mediated by endocytic retrieval of Npt2a from the BBM. These vesicles are shuttled to lysosomes and degraded. There is little to no recycling back to the proximal tubular cell membrane once transporters are endocytosed. New transporters must then be resynthesized and routed to the apical membrane via a subapical compartment. Acute regulation involves changes in endocytic rates. Endocytosis occurs between microvilli at intermicrovillar clefts and involves the actin cytoskeleton. Megalin may also play a role. It is mediated by a variety of protein kinases. Figure 11.2 summarizes this process.
FIGURE 11-2. Cellular model of proximal tubular phosphate transport. Sodium phosphate cotransporters (Npt2a) are distributed along the luminal membrane (dark circles). In response to PTH, transporters localize to the intermicrovillar region where they are endocytosed and degraded in lysosomes. This appears to be a unidirectional process. New transporters must be resynthesized and routed to the apical membrane via a subapical compartment (SAC). PTH binds to receptors in both the luminal and basolateral membrane. Parathyroid hormone receptormediated signaling pathways (protein kinase A [PKA] and protein kinase C [PKC]) differ at the basolateral and luminal membranes.
Phosphatonins, circulating phosphaturic peptides, decrease BBM abundance of Npt2a/Npt2c in vivo and suppress 1α-hydroxylase activity in kidney. Amongst the phosphatonins such as FGF-23, secreted frizzled related protein-4 (sFRP-4), matric extracellular phosphoglycoprotein (MEPE) and dentin matrix protein-1, FGF-23 is the most studied molecule requiring a cofactor Klotho to activate its receptor, fibroblast growth factor (FGF) receptor-1, in PCT. FGF-23 is a 251-amino acid protein produced by bone cells (osteocytes and osteoblasts) in response to high Pi diet, high serum Pi or 1,25[OH]2D3 concentration. Furthermore, the Klotho gene encodes a 1014-amino acid protein with a long extracellular NH2 terminal, a single transmembrane domain, and short intracellular carboxyterminus. Klotho exists in 2 forms: secreted; and transmembrane. It is expressed in kidney, brain, pituitary, parathyroid, ovary, testis, skeletal muscle, duodenum, and pancreas. It is highly expressed in distal tubules, and is also expressed in PCT. How bone senses changes in serum Pi concentration and alters FGF-23 secretion is unknown. Furthermore, intestinal mediators may regulate FGF-23 secretion. This hypothesis arises from the observation that hyperphosphatemia induced by dietary load increases FGF-23 levels, whereas nondietary interventions, like potassium phosphate infusion, do not.
FGF-23 when injected into experimental animals reduces calcitriol concentration within 3 hours. This occurs as a result of decreased calcitriol synthesis (decreased expression of 1 α-hydroxylase) and increased degradation (increased expression of 24-hydroxylase). Serum phosphorus concentration and NaPi-2a fall after 9 to 13 hours. This effect occurs in parathyroidectomized animals indicating that it is PTH-independent. It is likely that only a part of the phosphaturic effect of FGF-23 is related to decreased calcitriol concentration. Calcitriol injection into mice results in an increase in FGF-23 concentration and FGF-23 KO mice have high serum calcitriol concentrations. Taken together, these studies indicate that FGF-23 plays a central role in feedback regulation of calcitriol concentration. It also plays a significant role in inhibiting PTH secretion from the parathyroid gland.
Pi depletion and 1,25[OH]2D3 increase Pi Tm. With the discovery of novel molecules such as NHERF (sodium proton exchanger regulatory factor), we know that animals adapt to dietary Pi changes that are mediated by synchronous changes in trafficking Npt2a/Npt2c to the apical membrane in kidney. These changes are absent in NHERF null mice as they fail to correct phosphaturia and fail to increase expression of Npt2a with dietary Pi depletion. So far, the direct effect of 1,25[OH]2D3 on renal Pi transport is controversial. It may alter Pi reabsorption by changing serum calcium and PTH concentrations.
Other factors that regulate renal phosphate reabsorption in humans are worth mentioning. Estrogen increases serum FGF-23 concentration and decreases Npt2a messenger ribonucleic acid (mRNA) levels, thyroid hormone increases Npt2a gene expression and glucocorticoid excess inhibits Pi reabsorption by decreasing Npt2a mRNA and protein abundance in BBM. Metabolic acidosis directly inhibits Npt2a, causing phosphaturia, in an attempt to increase net acid excretion.
Hence, Pi homeostasis is a highly regulated process involving several organs and phosphatonins play a major role in orchestrating the bone-kidney-endocrine axis.
KEY POINTS
HYPERPHOSPHATEMIA
Etiology
Hyperphosphatemia most commonly results from decreased renal phosphate excretion. Chronic kidney disease (CKD) is the cause in greater than 90% of cases. Other causes involve abnormally increased proximal tubular phosphate reabsorption. Furthermore, an acute phosphorus load from either exogenous or endogenous sources can also cause hyperphosphatemia. Table 11.2 lists the etiologies of hyperphosphatemia, grouped by pathophysiologic categories.
TABLE 11-2. Etiologies of Hyperphosphatemia
As glomerular filtration rate (GFR) declines below 60 mL/min/1.73 m2 renal phosphate excretion increases. Once GFR falls below 30 mL/min/1.73 m2, however, phosphate reabsorption is maximally inhibited and renal excretion cannot increase further. At this point, dietary intake will exceed renal excretion and serum phosphorus concentration must increase. A new steady state is established at a higher serum phosphorus concentration. Approximately 15% of patients with a GFR of 15 to 30 mL/min/1.73 m2 and 50% of those with a GFR less than 15 mL/min/1.73 m2 have a serum phosphorus concentration greater than 4.5 mg/dL.
FGF-23 levels are augmented in early CKD stages long before hyperphosphatemia manifests in such patients. CKD patients exhibit poor response to the phosphaturic effects of FGF-23 because of decreased nephron mass or FGF-23 resistance. Furthermore, high FGF-23 and low calcitriol levels suppress Klotho expression in kidney and parathyroid gland. Decrease in Klotho provides FGF-23 resistance and perpetuates this vicious cycle of abnormal Pi metabolism in CKD patients. A progressive decline in urinarysecreted Klotho occurs during CKD progression, which is evident in early CKD stages. Inhibition of Npt2a and augmentation of TRPV5 (transient receptor potential vanilloid-5) activity by secreted Klotho is mitigated with CKD, which may explain increased Pi reabsorption in these patients. We now know from FGF-23 KO mice that exhibit hyperphosphatemia, high 1,25[OH]2D3 levels, vascular calcification, and early mortality, that reducing serum Pi concentration in these animals by either ablating the VDR or administering a low Pi diet rescues their phenotype. FGF-23 and Klotho KO models exhibit markers of oxidative stress, hypogonadism and generalized tissue atrophy. There are several overlapping aging-like phenotypes, including hypogonadism, skin atrophy, osteopenia, vascular calcification, and cognitive impairment, that present in CKD patients. Phosphate toxicity may play a pivotal role in these toxic effects.
CKD patients suffer disproportionately from cardiovascular mortality. In fact, compared to CKD patients with a serum Pi concentration less than 6.5 mg/dL, those with equal to or greater than 6.5 mg/dL levels have higher mortality. Multiple observational studies show a linear relationship between high Pi levels and high allcause and cardiovascular mortality. There seems to be a dose–response effect in regards to serum Pi concentration and mortality in patients with CKD (all stages), end-stage renal disease (ESRD), and those with normal renal function. Based on observational data, each 1 mg/dL rise in serum Pi concentration increased allcause mortality by 10% in patients with CKD stages 3 to 4. The REIN (Ramipril Efficacy in Nephropathy) trial showed that each 1 mg/dL rise in serum Pi concentration was associated with 85% excess risk of progression to ESRD that was consistent with the Chronic Renal Impairment in Birmingham (CRIB) study cohort. CKD patients have accelerated atherosclerosis and medial calcification. Some reports suggest that each 1 mg/dL rise in serum phosphorus concentration is associated with 21% and 25% greater prevalence of coronary artery and aortic calcification. Calcification in large arteries reduces compliance, increases pulse pressure and subsequently afterload, favoring left ventricular hypertrophy (LVH)-compromised coronary perfusion. Moreover, Pi interacts with several autocrine, paracrine, and endocrine factors to exhibit toxic effects on vascular smooth muscle cells. Vascular calcification is a complex ectopic biomineralization process where imbalance in inhibitors of mineralization, rate of cell death (providing nucleus for apatite crystals), presence of circulating nucleating factors (from increased bone turnover), and pathologic expression of bone proteins occurs in vascular smooth muscle cells.
There are other potential but uncommon causes of hyperphosphatemia. First, increased renal phosphate reabsorption is an uncommon pathophysiologic mechanism for the development of hyperphosphatemia. It occurs in hypoparathyroidism as a result of decreased PTH concentration. In acromegaly, insulin-like growth factor-1 stimulates phosphate transport. Bisphosphonates directly increase renal phosphate reabsorption, but this effect is usually offset by secondary hyperparathyroidism that results from a decrease in serum calcium concentration. Tumoral calcinosis is an autosomal recessive disease associated with hyperphosphatemia and softtissue calcium deposition. This condition arises either from: mutations in the GALNT3 gene that encodes a glycosyltransferase that is involved in O-linked glycosylation of FGF-23; mutations in FGF-23 that increase its cleavage by proteases; and defects in Klotho, thereby rendering FGF-23 and FGFR-1 interaction abnormal. It is the intact FGF-23 molecule that is biologically active. This results in increased FGF-23 processing and diminished phosphaturic effects.
Second, serum phosphorus concentration also increases as a result of an acute large phosphorus load. Phosphorus can be released from an endogenous source (within cells), as in tumor lysis syndrome, hemolysis, or rhabdomyolysis. Exogenous phosphorus sources reported to cause hyperphosphatemia include phosphorus-containing laxatives and enemas, high-dose liposomal amphotericin (contains phosphatidylcholine and phosphatidylserine), and solvent-detergent-treated fresh-frozen plasma (contained improper amounts of dihydrogen phosphate used as a buffer in the purification process). Oral sodium phosphate solution was commonly used as a bowel preparation agent for colonoscopy. It can be given in a small volume (45 mL 18 and 6 hours before the procedure) and is less expensive than polyethylene glycol-based solutions. These 90 mL contain 43.2 g of monobasic sodium phosphate and 16.2 g of dibasic sodium phosphate. A variety of rare renal complications occur with its use. Fatal hyperphosphatemia was reported in a renal transplantation patient, serum phosphorus concentration 17.8 mg/dL, who received a single oral dose of 90 mL and suffered a cardiorespiratory arrest 6 hours later. A case series of 5 patients was reported with acute kidney injury (mean serum creatinine concentration 4.9 mg/dL) secondary to acute calcium phosphate precipitation in distal tubules and collecting ducts and severe tubular damage. Four were prescribed angiotensin-converting enzyme inhibitors or angiotensin receptor blockers, and 2 were taking diuretics. At 6 weeks renal function was unchanged in 4 of the 5 patients. When given to normal volunteers ages 21 to 41 years with normal renal function, oral phosphosoda caused a rise in serum phosphorus concentration to 7.6 mg/dL and a fall in serum calcium concentration to 8.4 mg/dL. There were no adverse clinical effects as a result of these changes. As many as 37% of patients with a creatinine clearance greater than 70 mL/min have an increase in serum phosphorus concentration to greater than 8.0 mg/dL. Taken together, these studies indicate that oral sodium phosphate solution should be used with caution in those older than age 55 years, those with decreased gastrointestinal (GI) motility, and in the presence of volume depletion. It should not be used at all in patients with CKD stages 3 to 5.
Fourth, spurious hyperphosphatemia occurs as a result of interference in the assay to measure serum Pi concentration. Hyperlipidemia, hyperbilirubinemia, hemolysis, paraproteinemia, and contamination of blood samples with heparin can cause spuriously elevated serum Pi levels.
KEY POINTS
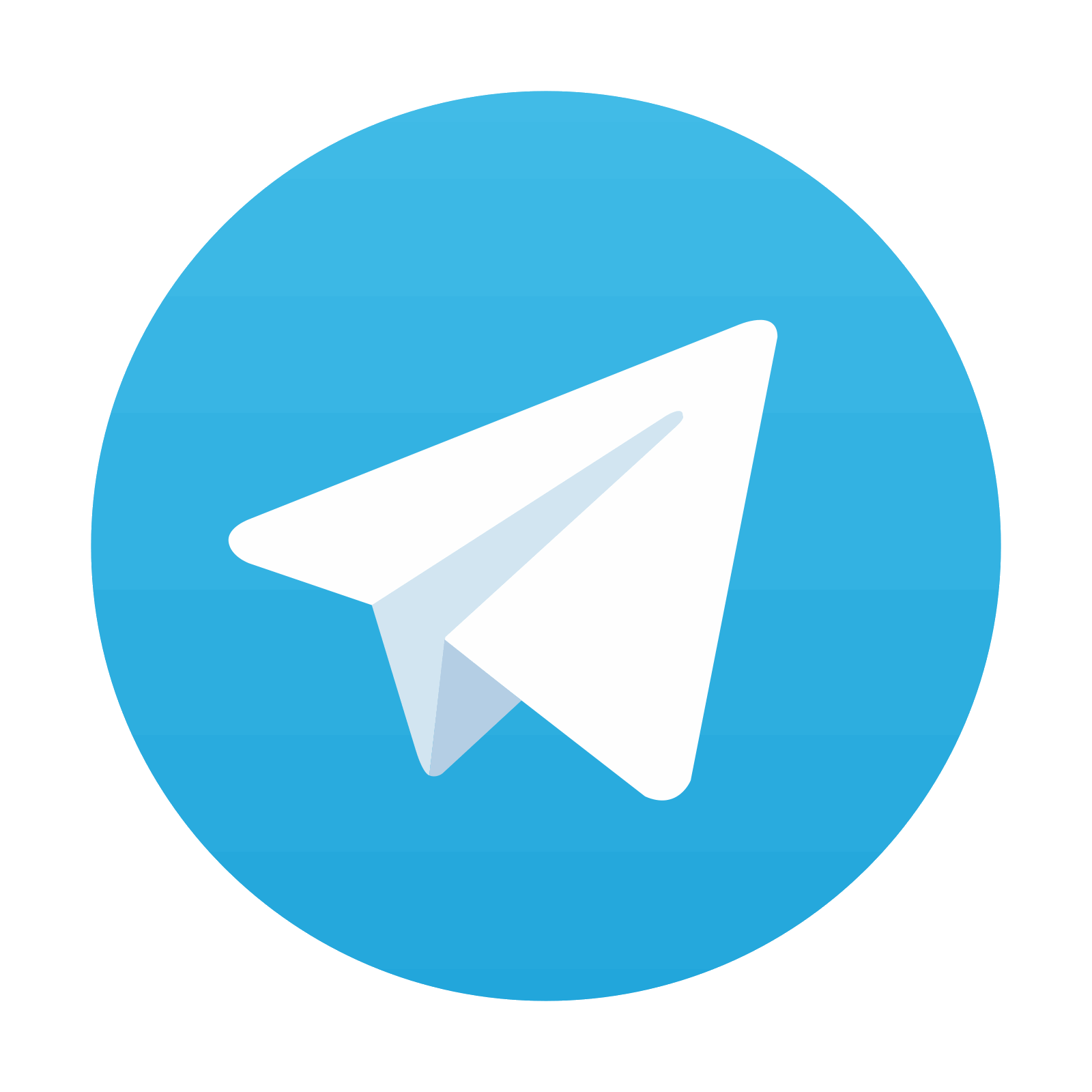
Stay updated, free articles. Join our Telegram channel
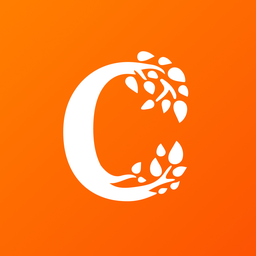
Full access? Get Clinical Tree
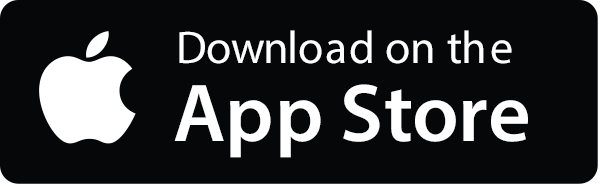
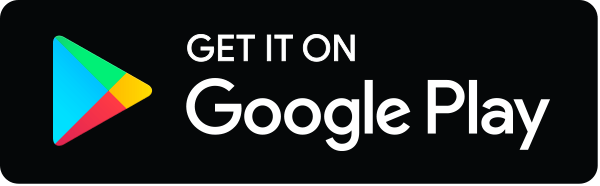