David M. Berman, MD, PhD, Ronald Rodriguez, MD, PhD, Robert W. Veltri, PhD The focus of this chapter is on the development, anatomy, histology, and physiology of the prostate and seminal vesicles, the male sex accessory glands that contribute to seminal fluid. It is worth noting that these roles are deduced by anatomic and physiologic observation but that male infertility is infrequently traced to disorders of these organs. Therefore, their physiologic roles in reproduction are either unimportant or, as the authors believe, exceedingly resistant to malfunction. In the specific case of the prostate, its physiologic role is overshadowed by a striking vulnerability toward other types of disease—pathologic prostate growth represents an especially important cause of morbidity and mortality in men. Because pathologic prostate growth in adults involves reactivation of physiologic growth and differentiation pathways that act in embryonic and perinatal development (Marker, 2008; Schaeffer et al, 2008; Pritchard et al, 2009), regulation of these events is a major scientific and clinical question and comprises the main discussion in this chapter. Our understanding of prostate growth control is based on phenotypic evaluation humans with genetic diseases affecting the androgen receptor (AR) signaling axis and on analysis of laboratory animals or cultured cells subjected to genetic, surgical, or pharmacologic manipulation. The experimental data represent the broadest and deepest vein of information on prostate growth control and are the major source for the discussion of this topic. Possible physiologic roles of cellular constituents of the prostate are described in the final section of this chapter, and this discussion may be useful for students of prostate biomarkers. The sex accessory tissues include the epididymis, ampullae, seminal vesicles, prostate, Cowper (bulbourethral) gland, and glands of Littre. All of these glands have reproductive roles, but the seminal vesicles work in tandem with the prostate and provide an important counterpoint to its biology and pathologic processes. The seminal vesicles are two saccular glands that pair with the vasa deferentia to form the ejaculatory ducts that empty into the craniodorsal aspects of the prostate. Together with the prostate the seminal vesicles produce seminal fluid that nurtures, protects, and facilitates sperm transport for mammalian reproduction. The division of labor between the two glands is surprisingly variable. At one of end of the spectrum is the dog, a species in which seminal vesicles are absent and the prostate must therefore carry out the functions that are divided between the two glands in other species. Most mammals, including humans, rats, and mice, occupy the other end of the spectrum, in which the seminal vesicles produce most of the seminal fluid, with the prostate playing a minor role. In species with both glands, physiologic cooperation between the two glands is also appreciable at the molecular level. For example, the major secretory protein product of the seminal vesicles is semenogelin, a 52-kD protein that serves as a substrate for proteolytic enzymes produced by the prostate, including prostate-specific antigen (PSA). Proteolysis of semenogelin yields a variety of peptide byproducts that are believed to serve reproductive and antimicrobial functions in humans (Curry and Atherton, 1990). In mice and rats, seminal vesicle and prostate products cooperate to coagulate the ejaculate into a firm copulatory plug in the vagina during mating. The plug serves as a temporary barrier to further mating by the female, potentially blocking impregnation by competing males. Seminal vesicles develop from the mesonephric (wolffian) ducts shortly before the onset of prostate development. Seminal vesicle development is strictly dependent on an intact AR signaling pathway, including the ligand testosterone (reviewed in Wilson et al, 1981). This requirement contrasts to development of the human prostate (see later), which, in addition to an intact AR pathway, requires conversion of testosterone into the more potent 5α-reduced androgen, dihydrotestosterone (DHT) (Andersson et al, 1991; Mahendroo and Russell, 1999). Thick smooth muscle layers constitute the muscular stroma of the seminal vesicles, which surrounds a short columnar-to-cuboidal epithelium. The epithelium has distinct basal and luminal layers that are notable for unusually variable nuclear size and shape, a feature that is also found in prostate cancer (Epstein and Netto, 2007). Another notable feature of the epithelium of the seminal vesicles, the almost invariable appearance of gold-colored intracytoplasmic pigment, is usually absent in prostate cancer and helps to clarify any confusion between the two entities. Seminal vesical pigment is thought to derive from cellular byproducts of nonviable sperm ingested by the seminal vesical epithelium (spermatophagy). The seminal vesicles are extremely resistant to disease. Given their proximity, shared functions, and similar endocrine requirements to the prostate, it is striking that diseases of the seminal vesicles in humans are vanishingly rare. In contrast, prostate disease, at least in Western cultures, is a nearly universal rite of passage into old age (see subsequent chapters on benign prostatic hyperplasia and prostate cancer). Accordingly, contrasting gene expression between seminal vesicles and prostate has been used as a strategy to discover the molecular basis of prostate cancer risk (Thompson et al, 2008). The prostate is a derivative of the primitive endoderm (gut tube). Regional differentiation of the primitive gut tube into foregut, midgut, and hindgut is followed by a swelling at the caudal end that creates the cloaca (see Chapter 111). The cloaca, a Latin term meaning “sewer,” receives output from both the urinary and intestinal tracts and represents the fully differentiated state in birds, reptiles, amphibians, marsupials, and monotrenes. In placental mammals, however, the cloaca is divided by the urorectal septum during embryogenesis to create separate urinary and digestive outlets. The ventral urinary compartment is called the primitive urogenital sinus, which further segments into the urinary bladder at its cranial end and the urethra at its caudal terminus. In males, the prostate develops just caudal to the bladder neck via the proliferation of epithelial buds extending out from the urogenital sinus epithelium. Prostate buds invade at stereotyped locations that pattern the future development of distinct prostate lobes in the rodent and, potentially, zones in the human. These regions prepare for epithelial bud invasion by “mesenchymal condensation,” a process in which urogenital sinus mesenchymal cells (cells constituting loose connective tissue that will differentiate into stromal elements) become closely packed together (reviewed in Thomson, 2008). This condensation occurs in both males and females and is therefore androgen independent. In contrast, epithelial budding is strictly androgen-dependent and represents the first events in prostate development that are identifiable at the light microscopic level. Prostate budding requires intricate epithelial-mesenchymal interactions (Fig. 90–1). In humans, prostate budding occurs during the 10th week of gestation. In mice, prostate budding occurs on the 17th gestational day, 2 days before birth. Importantly, androgen exposure is not only necessary but also sufficient to drive prostatic differentiation and growth in the embryo. This fact, along with the ability to easily manipulate androgen levels in experimental animals, makes the prostate a particularly appealing subject for the study of epithelial cell fate determination (Cunha et al, 1987; Schaeffer et al, 2008). Prostate buds initially grow as solid epithelial cords that subsequently (postnatal days 1 to 14 in mice) branch and canalize (Sugimura et al, 1986) as part of a sophisticated branching morphogenesis program. In the mouse, the urogenital sinus epithelium begins as a homogeneous cell compartment that differentiates (after birth in mice) into distinct basal (adjacent to stroma) and luminal layers (Wang et al, 2001). Intervening epithelial cells, called “intermediate cells,” are present that have features of both basal and luminal cells. A fourth cell type, the neuroendocrine cell, is present in large numbers before prostate epithelial budding and decreases during embryonic development (Aumuller et al, 2001). The development of this cell type during mouse embryogenesis has not been well characterized, and the source of these cells has been variously proposed to be neural crest or urogenital sinus endoderm (Aumuller et al, 2001; Goldstein et al, 2008), illustrating the need to further delineate lineage commitment events in prostate epithelium. Although AR signaling through DHT is the primary motivating force behind prostate development, it specifies only the timing of events not their location. The AR signaling machinery is present diffusely throughout the lower genitourinary tract (Takeda et al, 1985; Berman et al, 1995). Prostate epithelial buds form at precise locations through mechanisms that are not understood. This spatial control may involve paralogous homeobox (Hox) genes, which are transcriptional regulators that govern differential gene expression along the craniocaudal (head to tail) and proximodistal (e.g., shoulder to fingertip) axes in a variety of tissues, including the genitourinary tract (reviewed in Beck et al, 2000; Kmita and Duboule, 2003). In vertebrates, the paralogous Hox genes exist as four similar clusters (clusters A, B, C, and D), each of which resides on a separate chromosome and encodes genes whose chromosomal position from 3′ to 5′ mirrors their expression pattern in the embryo. The paralogous genes are distinct from other more distantly related transcription factor families that also contain DNA-binding homeobox motifs, such as the NK family whose members are expressed in a more discrete, organ-specific manner (e.g., see Nkx3.1, later). Paralagous Hox genes are sequentially numbered from 1 to 13, with the higher numbers in the 5′ position showing the most distal or caudal expression patterns. Accordingly, Hoxa13, Hoxb13, and Hoxd13 are paralogues on chromosomes 7, 17, and 2, respectively, that have overlapping expression patterns and functions in distal genitourinary tract development. Hoxb13 regulatory elements have been characterized that restrict its function to the caudal end of the genitourinary and digestive tracts and can be used to engineer androgen-independent prostatic expression of genes of interest (McMullin et al, 2009). Homozygous mutations in individual Hox genes result in subtle changes in prostatic branching patterns (Podlasek et al, 1997) and/or defective epithelial maturation (Economides and Capecchi, 2003). Mutations involving more than one of these genes results in significantly more severe urogenital phenotypes, such as significant prostate hypoplasia in Hoxd13/Hoxb13 compound mutant mice or failure of separate urinary and gastrointestinal tract outlets to form in Hoxa13/Hoxd13 compound mutants (Kondo et al, 1997; Warot et al, 1997). These multigene experiments are technically challenging and have not yet revealed the effects of complete loss of Hox gene function in the prostate. Mesenchymal condensation occurs in both males and females, so it is not sufficient to drive prostate development but may be necessary. Condensation of the ventral mesenchymal pad is defective in mice lacking the gene for Noggin (see TGF-β family section later for further discussion of the roles of Noggin in prostate development), which antagonizes binding of bone morphogenetic protein (BMP) ligands to their receptors (Cook et al, 2007). This observation suggests that BMP signaling enhances mesenchymal condensation, either by direct action on mesenchyme or through regulation of epithelial-derived factors important in this process. Condensed mesenchyme is highly enriched for expression of fibroblast growth factors (FGFs) that are essential for epithelial bud outgrowth. For example, mice with engineered mutations of the mesenchyme-specific growth factor Fgf10 gene generate small abortive epithelial buds and fail to grow prostates (Donjacour et al, 2003). Epithelial expression of the NK homeobox transcription family member Nkx3.1 is the earliest indicator of prostate development at the molecular level. This transcription factor influences the degree of branching in the mature mouse prostate, where it can act as a tumor suppressor (Bieberich et al, 1996; Bhatia-Gaur et al, 1999; Abate-Shen et al, 2008). Nkx3.1 (Bhatia-Gaur et al, 1999), Sox9 (Lupien et al, 2008; Schaeffer et al, 2008; Thomsen et al, 2008), and sonic hedgehog (Podlasek et al, 1999) are expressed in emerging prostate buds, but prostate buds can emerge in the absence of these factors (see later). Mutations in the transcriptional regulator p63 (TP63) (Signoretti et al, 2000) or in the AR signaling axis (reviewed in Cunha et al, 1987) can completely abolish prostate induction. Noggin mutations selectively impair budding of the ventral lobes of the prostate, leaving anterior and dorsolateral budding unimpaired (Cook et al, 2007). Overall, however, the process appears to be a very robust one, with evidence of prostate epithelial bud formation persisting in the presence of a variety of genetic mutations that affect future steps in prostate ductal morphogenesis, particularly branching morphogenesis. TP63 has transcriptional repressor and activator activities that balance differentiation and stem/progenitor cell functions in epithelia (McKeon, 2004). The transcriptional targets of TP63 in prostate epithelial cells remain to be elucidated (Grisanzio and Signoretti, 2008). One of the most striking aspects of the induction of prostate epithelial budding is the finding by Cunha and Lung (1978) that AR signaling is required in the mesenchyme but dispensable in the epithelium. Thus the action of androgens in this process appears to be indirect. This has led to the hypothesis that mesenchymal cells secrete inductive factors in response to androgens called “andromedins” (Yan et al, 1992). Andromedins have proven to be elusive quarry. Indeed, androgen-regulated mesenchymal factors have been difficult to identify much less characterize functionally (reviewed in Thomson, 2008), leading to alternative hypotheses involving androgen-mediated suppression of soluble factors (Tenniswood, 1986) or of smooth muscle cell barriers (Thomson et al, 2002) that inhibit induction of prostate epithelial budding. Studies to unravel the mechanism by which AR can indirectly induce prostate lineage commitment and growth are ongoing and are likely to yield important insights into the basic underpinnings of both physiologic and pathologic prostate growth. Once set in motion, prostate growth and homeostasis continues to require androgens throughout life and this requirement appears to continue to be indirect, through mesenchymal or stromal AR signaling. Epithelial branching morphogenesis occurs through signaling cascades that inhibit further outgrowth along the long axis of an extending epithelial bud while stimulating lateral growth at its tip (Hogan, 1999). Through engineered deletion of genes in transgenic mice, several individual genes and components of classic morphogenetic pathways have been shown to be required for branching morphogenesis. Indeed, morphologic aberrations seen on interruption of a cellular pathway may be the most sensitive measure of a role for that pathway in regulation of prostate growth. As such, a wide variety of genes and pathways have been strongly implicated in prostate branching morphogenesis, only a few of which are covered here. For a more comprehensive perspective, including additional pathways such as those centered around Notch and Forkhead proteins, the reader is referred to recent reviews (Leong and Gao, 2008; Matusik et al, 2008). The transcription factor Nkx3.1 (see earlier) helps determine the branching pattern of the prostate, as demonstrated by the reduced numbers of duct tips seen in mice with engineered Nkx3.1 deletion (Bhatia-Gaur et al, 1999). This relatively subtle phenotypic change may be important, however, as indicated by a dramatic decrease in the ability of Nkx3.1-mutant prostates to manufacture mature secretory proteins (Bhatia-Gaur et al, 1999). The regulatory machinery that promotes Nkx3.1 expression early in prostate induction has been used to drive expression of Cre recombinase to specifically inactivate other genes in prostate epithelial cells (Lin et al, 2007; Thomsen et al, 2008; Zhang et al, 2008). Nkx3.1-Cre–mediated deletion was used to show that the sex-determining region Y–box 9 (Sox9) transcription factor is required for the development of the ventral lobe of the mouse prostate. Ventral lobe buds appeared at the normal embryonic age but failed to grow out and branch. The specificity of this proliferative defect for the ventral lobe, rather than other lobes, could be due to either a lobe-specific role for Sox9 or to a delay in Cre-mediated Sox9 excision found in the other prostate lobes. The latter possibility would be consistent with a transient requirement for the protein in ductal outgrowth (Thomsen et al, 2008). The fibroblast growth factor (FGF) family of related secreted peptides promote growth in recipient cells by binding to cell surface receptors and activating intracellular second messenger cascades. Epithelial branching morphogenesis, be it in the lung, salivary gland, mammary gland, or prostate, requires such signals to proceed. Of the FGFs, Fgf-7 (keratinocyte growth factor) and Fgf-10 have been studied most extensively in prostate development. Both of these ligands preferentially bind to Fgfr-2 over the three other family members (FGFRs 1, 3, and 4) (reviewed in Thomson, 2001, 2008). Ligand binding activates the intracellular microtubule-associated protein kinase (MAPK) pathway, leading to enhanced activity of growth-promoting transcription factors and increased proliferation. Fgfr-2 is expressed on developing prostate epithelial cells where it can interact with its coreceptor Frs-2α. Fgf-7 and Fgf-10, in contrast, are secreted by prostate mesenchyme. This arrangement, along with androgen-independent growth of prostate organ cultures exposed to these ligands, have led to the proposal that they act as andromedins (Yan et al, 1992; Lu et al, 1999). However, Thomson and associates (2002) make a compelling argument to the contrary, pointing out that Fgf-7 and Fgf-10 expression patterns are equivalent in male and female rodent embryos and are therefore unlikely to mediate AR signaling from mesenchyme to epithelium. Instead, FGF signaling appears to have a critical role in epithelial outgrowth that is downstream of as yet undetermined prostate-inductive events. This role was demonstrated most dramatically by almost complete failure of prostate development in Fgf-10–deficient mice (Donjacour et al, 2003) and further supported by prostate hypoplasia and decreased epithelial branching in mice with prostate-targeted deletion of the genes encoding Fgfr-2 or Frs-2α. Across a variety of organs, elaboration of secreted hedgehog ligands (Sonic hedgehog, Indian hedgehog, and Desert hedgehog) by epithelial cells and reception in adjacent mesenchyme coordinates the activities of the Gli family proteins in regulating hedgehog pathway target genes. In the mesenchyme of developing prostate, several Hh target genes have been identified (Yu et al, 2009), including the cytokine Cxcl14, the insulin-like growth factor–binding protein Igfbp3, and the delta/notch-like epidermal growth factor–related receptor Dner. The roles of these particular genes in prostate development have yet to be ascertained, but, as a whole, Hh pathway target genes have been implicated in placement of prostate epithelial buds and in subsequent ductal branching and outgrowth. In particular, buds form in the absence of the dominant Hh ligand in the prostate (Berman et al, 2004) but are mislocalized in prostates of mice bearing mutations of downstream effectors of the pathway, Gli proteins (Doles et al, 2006). Later on in development, Hh ligands enhance epithelial outgrowth and branching (Freestone et al, 2003), which proceed abnormally in prostate organ cultures treated with Hh pathway antagonists (Lamm et al, 2002; Freestone et al, 2003; Berman et al, 2004). In adult animals the pathway may play a role in homeostasis, as indicated in a failure of prostates to regenerate after castration of animals treated with antibodies or small molecules that block Hh signaling (Berman et al, 2004). Taken together, these data indicate a growth-promoting role for the pathway in prostate epithelium, one that may have clinical relevance in pathologic prostate growth (reviewed in Shaw and Bushman, 2007). TGF-β superfamily members include TGF-β itself, as well as members of the growth and differentiation factors (GDFs) and the bone morphogenetic proteins (BMPs). These factors act through transmembrane receptors and the SMAD family of intracellular signal transducing proteins (Schmierer and Hill, 2007). Little is known about GDFs in the prostate, but both TGFs and BMPs are likely to play important roles. In organogenesis this superfamily is best known as a mesenchymal mediator of epithelial growth suppression, but (less frequently) they can also stimulate growth and/or be produced by epithelial cells. TGF-β1 inhibits net growth of the prostate but can stimulate growth in certain regions of the gland, particularly in the distal tips of the ventral prostate (Tomlinson et al, 2004a). Although the mechanism for growth promotion by TGF-β1 is unclear, the growth suppressive effect could quite reasonably relate to its ability to suppress levels of another mesenchymal growth factor FGF-10 (Tomlinson et al, 2004b) (see FGF section, earlier). A similar mechanism could remain in place in mature males, where TGF-β signaling in proximal ducts is believed to help to maintain prostate epithelial stem cells in a quiescent (growth-suppressed) state (Salm et al, 2005). BMP4 and BMP7 exert important and highly localized growth-suppressive activities in prostate development that help guide branching morphogenesis and prevent overproduction and disorganized epithelial growth. Like TGF-β, BMPs are most active during epithelial budding and subsequent prostate branching (Lamm et al, 2001; Tomlinson et al, 2004a; Grishina et al, 2005) (embryonic day 17 through postnatal day 5 in the mouse). Activation of BMP signaling suppresses branching morphogenesis, as indicated by experimental addition of exogenous BMP4 or BMP7 protein to prostate organ cultures (Lamm et al, 2002; Grishina et al, 2005) or by genetic deletion of the BMP inhibitor Noggin (Cook et al, 2007). BMP inactivation can show the opposite effect, as epithelial overgrowth of prostates with genetic deletion of BMP7 (Grishina et al, 2005). The rodent prostate is divided into paired anterior, dorsolateral, and ventral lobes. Each empties into to the urethra separately at its proximal extreme, with the distal end floating freely in the pelvic cavity. In contrast, the human prostate, like that of most primates and canine species, grows as a single organ encircling the urethra. In man and in dog, the latter arrangement is coupled with a propensity to grow in old age (benign prostatic hyperplasia), making males vulnerable to urinary obstruction (see Chapter 91). In rodents, the anterior, ventral, and dorsolateral lobes are named for the distinct locations of the urethra from which they originate. Each lobe also has a different branching pattern with a distinctive histologic appearance. These differences reviewed by Timms (2008) have been likened to different zones of the human prostate, histologically (Price, 1963), molecularly (Berquin et al, 2005; Thielen et al, 2007), and in terms of propensities to be affected by disease. In mice, mRNA transcripts for spermine-binding protein, probasin, and renin-1 are specific for ventral, dorsolateral, and anterior lobes, respectively (Cook et al, 2007), whereas human zone specific gene expression has not been as well characterized. Of particular interest, the mouse dorsolateral prostate shares molecular and histologic similarities to the peripheral zone of the human prostate, the zone most prone to the development of cancer. The prostatic epithelium in the human is composed of two major cellular compartments: epithelial cells and stromal cells (Table 90–1). The prostate epithelial compartment consists of basal epithelial cells, intermediate cells, neuroendocrine cells, and luminal secretory epithelial cells (reviewed by De Marzo et al, 1998a). The stromal compartment architecturally serves as structural support and consists predominantly of connective tissue, smooth muscle cells, and fibroblasts. Most prostate cell types have been characterized in vitro (Peehl, 2005). Table 90–1 Summary of the Anatomy and Cell Biology of the Prostate Gland In most glands with renewing cell populations there is a steady-state flow of cells from mostly quiescent stem cells to a more rapidly dividing pool of transient proliferating cells. This proliferating population finally reaches terminal differentiation, characterized by metabolically active secretory epithelium. In the prostate, cell lineage has not been rigorously determined but has been inferred from a variety of sources. A hypothetical differentiation scheme for prostate epithelium is presented in Figure 90–2. As in most multilayered epithelia, stem cells reside in the basal compartment and appear to give rise to all of the other epithelial cell types, as well as neuroendocrine cells. These include fully differentiated secretory cells that line glandular lumina (luminal cells), neuroendocrine cells that secrete bioactive peptides, and intermediate cells that show phenotypic features that are intermediate between basal cells and luminal cells. (Modified from Wang Y, Hayward S, et al. Cell differentiation lineage in the prostate. Differentiation 2001;68[4-5]:270–9.) The luminal epithelial cell is the “workhorse” of the prostate gland, responsible for epithelial barrier integrity and production of prostatic secretion. Luminal cells constitute most of the prostate epithelium. These tall (10 to 20 µm) columnar secretory epithelial cells are terminally differentiated and have a low proliferative index (De Marzo et al, 1998a); they are easily distinguished by their morphologic features and abundant secretory granules and enzymes. Secretory cells produce a variety of proteins that characterize prostatic differentiation, including PSA, acid phosphatase, AR, leucine amino peptidase, and 15-lipoxygenase-2 (Shappell et al, 1999; Bhatia et al, 2003). They are also rich in keratin filaments (subtypes 8 and 18) (van Leenders and Schalken, 2003). These tall, columnar secretory cells appear in rows like a picket fence with each cell connected to the next by cell adhesion molecules; the apical aspect of these cells projects into the lumen, with the base attached to a basement membrane through integrin receptors (Knox et al, 1994). The nucleus is at the base just below a clear zone (2 to 8 µm) of abundant Golgi apparatus, and the upper cellular periphery is rich in secretory granules and enzymes. The apical plasma membrane facing the lumen possesses microvilli, and secretions move into the open collecting spaces of the acinus. These epithelial cells ring the periphery of the acinus and produce secretions into the acini that drain into ducts connected to the urethra. Neuroendocrine cells are cells that release hormones in response to neural stimulation. In the prostate, neuroendocrine cells reside among the more abundant secretory epithelial cells in the normal prostate gland as well as in the urothelium of the prostatic urethra (Aumuller et al, 2001). There are two types of neuroendocrine cells: the first is open and possesses specialized microvilli that protrude into the lumen; the second is closed with long dendrite-like processes that extend to nearby epithelial cells and basal cells close to afferent and efferent nerves (diSant-Agnese and deMesy-Jensen, 1984; diSant-Agnese et al, 1985; Abrahamsson, 1999; Vashchenko and Abrahamsson, 2005). Thinking on the origin of prostatic neuroendocrine cells has evolved. Aumuller and associates (2001) demonstrated that neuroendocrine cells are readily identified in male and female urogenital sinus epithelium before human prostate development, suggesting that these might represent a separate lineage that is independent of prostate epithelium. More recently, Goldstein and coworkers (2008) showed that neuroendocrine, basal, and secretory luminal cells can all originate from a common pluripotent Trop2-expressing prostate epithelial stem cell precursor. Current evidence suggests that neuroendocrine cells can influence growth, differentiation, and secretory activity of the prostate epithelium through paracrine and autocrine mechanisms (Abrahamsson, 1999; Vashchenko and Abrahamsson, 2005). Neuroendocrine cells bring about their regulatory activity by the secretion of hormonal polypeptides or biogenic amines such as serotonin. High-pressure liquid chromatography measurements have shown that normal human prostate tissue contains approximately 1400 ng of serotonin per gram of tissue, and this would certainly emphasize the importance of these cells (Davis, 1987). Higgins and Gosling (1989) have studied the structure and intrinsic innervations of the normal human prostate and have observed acetylcholinesterase-containing nerves associated with smooth muscle in both the peripheral and the central parts of the prostate. In addition, they have shown that the majority of the acini in the peripheral and central regions possess a rich plexus of autonomic nerves and that vasoactive intestinal peptide-positive nerve fibers are found in relation to the epithelial lining acini in the central and peripheral regions of the gland. Lepor and Kuhar (1984) characterized and studied the location of the muscarinic cholinergic receptor in human prostate tissue and localized it to the epithelial cells. This is consistent with the neuropharmacology of muscarinic cholinergic agonist, which has a marked effect on increasing prostatic secretion. However, the α1-adrenergic receptor has its effect in the human prostatic stromal compartment. This is of clinical importance because of the use of selective α1-adrenergic antagonists to alleviate bladder outlet obstruction secondary to benign prostatic hyperplasia (Lepor, 1993). Recent work has demonstrated three subtypes of the α1-adrenergic receptor (α1A, α1B, and α1D). Of these, the α1A receptor appears to be linked to smooth muscle contraction of the prostate (Lepor et al, 1993). Neuroendocrine cells are terminally differentiated (i.e., nonproliferating) and do not express detectable AR, PSA, or Bcl-2. These cells release peptide hormones or prohormones by fusion of intracellular granules with the cell membrane and exocytosis of their contents. In addition to serotonin, neuroendocrine cells produce numerous bioactive macromolecules (bombesin, neuron-specific enolase, calcitonin gene family members, thyroid-stimulating hormone–like peptide, somatostatin, synaptophysin, and parathyroid hormone–like peptide are major examples). Neuroendocrine factors appear likely to influence the growth, differentiation, and secretion of epithelium of the prostate in both normal and malignant conditions (Vashchenko and Abrahamsson, 2005). Basal cells (reviewed by De Marzo et al, 1998a) are the smallest of epithelial cells. They have a low mitotic index and are a minor population, accounting for less than 10% of the total cell number. Basal cells express a distinct keratin subtype profile (subtypes 5 and 14) compared with the columnar epithelial cells (subtypes 8 and 18). These cells are typically pyramid shaped with relatively little cytoplasm and condensed chromatin. Basal cells rest on the basement membrane wedged between the bases of adjacent, tall, columnar epithelial cells. The basal cell compartment has long been considered the likely source of the epithelial stem cells of the prostate because they are relatively undifferentiated with a low proliferative index (~1%) and almost devoid of secretory products, such as PSA and prostatic acid phosphatase (see Fig. 90–2). Androgen deprivation does not affect stem cells but rather enriches them, because the more fully differentiated cells initiate apoptosis. Experiments in rodents have shown after castration that testosterone supplementation can restore prostate growth by stimulating stem cell proliferation. When mice are castrated after implantation with human prostate primary xenografts and then restimulated with testosterone, the basal cell population is highly overrepresented, consistent with the concept that the human basal compartment also contains prostate epithelial stem cells (Huss et al, 2004). Recent experimental work in the mouse has provided powerful functional evidence for stem cell populations in the prostate, localizing them to the basal compartment, particularly in the proximal portions of prostatic ducts. These experiments used in vivo grafting assays to demonstrate critical stem cell characteristics including the ability of long-lived cells to proliferate indefinitely and to give rise to more differentiated phenotypes. Tsujimura and colleagues demonstrated DNA label-retaining prostate epithelial cells with long-term proliferative potential that were preferentially localized to the proximal segments of prostatic ducts of adult males (Tsujimura et al, 2002). Further studies have mapped the stem cell properties of proximal duct cells to those expressing the mouse stem cell antigen Sca1, the basal cell integrin α6 (Itga6 or CD49f), the tumor-associated calcium signal transducer Tacstd2 (also known as Trop2), and the stem cell factor receptor, c-kit (Burger et al, 2005; Xin et al, 2005; Lawson et al, 2007; Goldstein et al, 2008; Leong and Gao, 2008). Intermediate cells are so named because they possess phenotypic characteristics intermediate between basal and luminal cells. Similarities of these cells to prostate cancer cells have marked them as hypothetical substrates for neoplastic transformation (Verhagen et al, 1992; De Marzo et al, 1998b), although their susceptibility to carcinogenesis is unknown. These investigators proposed that intermediate cells fulfill a transient amplifying function, providing a short-term amplification function for the long-term proliferative capabilities of basal stem cells. Intermediate cells produce basal cell keratins (5 and 14) and the secretory cell keratins 8 and 18 (De Marzo et al, 1998b; Schalken and van Leenders, 2003). Uzgare and colleagues (2004) reported transient amplifying properties of human intermediate cells in culture: a high proliferative fraction with the ability to proliferate for a limited number of generations. Survival of terminally differentiated secretory luminal cells and proliferation of intermediate cells require androgens potentially acting indirectly through the secretion of androgen-regulated growth factors by the stromal compartment (andromedins) (Uzgare et al, 2004). Key Points: Prostate Epithelial Cell Types The noncellular stroma and connective tissue of the prostate make up what is termed the ground substance and the extracellular matrix in what was first suggested by Arcadi (1954) to play an important role in prostate function and disease. The extracellular matrix has long been recognized as one of the important inductive components during normal development of many different types of cells (Cunha, 1976; Hay, 1981; Bissell et al, 1982; Getzenberg et al, 1990; Risbridger et al, 2005). Classic tissue recombination experiments by Cunha and colleagues (1987) have clearly shown the direct importance of the isolated embryonic mesenchyme to the induction of differentiation of normal prostatic epithelial cells (see earlier discussion). The epithelial cells rest on the basement lamina or membrane, which is a complex structure containing, in part, collagen types IV and V, glycosaminoglycans, complex polysaccharides, and glycolipids. This layer forms an interface to the stromal compartment that provides structural support for the basal cells and their progeny. It consists of an extracellular matrix, ground substance, and a variety of stromal cells, including the fibroblasts, capillary and lymphatic endothelial cells, smooth muscle cells, neuroendocrine cells, and axons (reviewed in Taylor and Risbridger, 2008). The cytomatrix (cytoplasmic skeleton) terminates in the center of the cell by direct attachment to the nuclear matrix (Fig. 90–3). The prostatic epithelial cell therefore has direct structural linkage via the matrix system from the DNA to the plasma membrane. The cytomatrix then makes direct contact with the basement membrane, extracellular matrix, and ground substance of the stroma. This entire interlocking tissue scaffolding or superstructure is termed the tissue matrix and may have dynamic properties in ordering and controlling biologic processes as well as in the transport of secretions from the sex accessory tissues (Getzenberg et al, 1990; Konety and Getzenberg, 1999; Etienne-Manneville, 2004; Miner and Yurchenco, 2004; Hallmann et al, 2005). (From Getzenberg RH, Pienta KJ, Coffey DS. The tissue matrix: cell dynamics and hormone action. Endocr Rev 1990;11:399–416.) Understanding the biologic components of the tissue matrix system within sex accessory tissues is of paramount importance to understanding its physiology. The laminin proteins are glycoproteins of the extracellular matrix that mediate attachment of cells to the type IV collagen of the basement membrane (Miner and Yurchenco, 2004; Yurchenco et al, 2004; Hallmann et al, 2005). Laminin is produced by epithelial cells but not by fibroblasts; it is a large molecule (approximately 800 kD) with molecular domains that interact with the type IV collagen of the basement membrane and with integrin-type receptors within the cell surface glycocalyx of the epithelial cell (Aumailley et al, 2005). Laminins are the major anchor filaments in the basement membranes of epithelial cells that stabilize attachment of hemidesmosomes via α6β4 integrin (Brar et al, 2003; Miner and Yurchenco, 2004). The key functional properties of the laminins are cell adhesion, proliferation, differentiation, growth, and migration. Laminin surrounds the basement membrane of prostate acinar epithelial cells, capillaries, smooth muscle, and nerve fibers but not lymphatics, lymphocytes, or fibroblasts; the laminin structure and its distribution are disrupted in BPH and higher-grade prostatic interepithelial neoplasia and higher-grade prostate neoplasms (Sinha et al, 1989; Brar et al, 2003; Miner and Yurchenco, 2004). In summary, the development and maintenance of the prostate occurs through androgen-dependent and highly regulated tissue morphogenesis in processes involving epithelial cell differentiation, proliferation, and apoptosis (Cunha et al, 2004). Communication through numerous extracellular interactions is directed to the intracellular cytoskeleton and then to the nuclear matrix, which ultimately regulates a variety of transcriptional cell functions that control such critical phenotypic qualities as cell size and shape, cell motility, epithelial cell turnover, proliferation, and differentiation (Getzenberg et al, 1990; Pienta, 1993; Miner and Yurchenco, 2004). The generalized endocrine physiology of the prostate is depicted in Figure 90–4. The hypothalamus releases a small 10-residue polypeptide (decapeptide) referred to as luteinizing hormone–releasing hormone (LHRH), also called gonadotropin-releasing hormone (GnRH). Under the stimulation of LHRH the pituitary releases luteinizing hormone that is transported to the testes and acts directly on the Leydig cells to stimulate de novo steroid synthesis and release of testosterone, the major serum androgen of the body. Most of the estrogen in the male is derived from peripheral conversion of androgens to estrogens through aromatization. Exogenous estrogens, such as diethylstilbestrol, block androgen action not primarily by direct effects on the prostate but indirectly through blocking pituitary function. The estrogen causes a negative feedback on luteinizing hormone release that reduces the serum signal for testicular testosterone production; therefore, estrogen acts as an effective “chemical castration.” Because the testes produce the major serum androgen supporting prostate and sex accessory tissue growth, it is important to briefly review this function. In the normal human male the major circulating serum androgen is testosterone, which is almost exclusively (~95%) of testicular origin. Under normal physiologic conditions the Leydig cells of the testis are the major source of the testicular androgens. The Leydig cells are stimulated by the gonadotropins (primarily the luteinizing hormone) to synthesize testosterone from acetate and cholesterol. The spermatic vein concentration of testosterone is 40 to 50 µg/dL, approximately 75 times more concentrated than the level detected in the peripheral venous serum (Hammond, 1978), which is approximately 600 ng/dL. Other androgens also leave the testes by the spermatic vein, and these include androstanediol, androstenedione (3 µg/dL), dehydroepiandrosterone (7 µg/dL), and DHT (0.4 µg/dL). The concentrations of these androgens are much lower in the spermatic vein than the concentration of testosterone, with all being less than 15% of the concentration of testosterone. The average testosterone concentration in the adult human male plasma is approximately 611 ng/dL ± 186 with a normal range of 300 to 1000 that is equal to 10.4 to 34.7 nmol/L in SI units (Table 90–2). Serum testosterone level is not remarkably related to age between 25 and 70 years, although it does decline gradually to approximately 500 ng/dL after 70 years of age. It is recognized that plasma concentrations of testosterone can vary widely in an individual on any one day and may reflect both episodic and diurnal variations in the production rate. Although testosterone is the primary plasma androgen inducing growth of the prostate gland and other sex accessory tissues, it appears to function as a prohormone in that the most active form of the androgen in the prostate is not testosterone but rather DHT (Farnsworth and Brown, 1963; Anderson and Liao, 1968; Bruchovsky and Wilson, 1968) (Fig. 90–5). The formation of DHT involves the reduction of the double bond in the A ring of testosterone through the enzymatic action of the enzyme 5α-reductase (Fig. 90–6). There are at least two isoforms of this enzyme (type 1 and type 2). Type 2 5α-reductase expression predominates in human accessory sex tissues and is localized to the fibromuscular stromal compartment (Silver et al, 1994). The type 1 isoform predominates in skin, in prostatic epithelia, and to a lesser extent in prostatic fibromuscular stroma. Inhibition of 5α-reductase by finasteride appears to be largely selective for the type 2 isoform (Iehle et al, 1995; Habib et al, 1997); the newer agent dutasteride inhibits both type 1 and type 2 5α-reductase. Both drugs appear to exert similar effects in terms of reduction in prostatic volume and serum PSA concentration, suggesting that the type 2 isoform is the only clinically significant isoform present in the prostate. DHT concentration in the plasma of normal men is low, 56 ± 20 ng/dL, in comparison to testosterone, which is 11-fold higher at approximately 611 ng/dL (see Table 90–2). In summary, although DHT is a potent androgen (2 to 10 times as potent as testosterone in many bioassay systems), its low plasma concentration and tight binding to plasma proteins diminishes its direct importance as a circulating androgen affecting prostate and seminal vesicle growth. In contrast, DHT is of paramount importance within the prostate, where it is formed from testosterone. DHT is the major form of androgen found within the prostate gland (5 ng/g tissue wet weight) and is fivefold higher than testosterone. In the prostate, DHT binds to ARs and activates the receptors to regulate a variety of cellular processes. In summary, DHT becomes the major androgen regulating the cellular events of growth, differentiation, and function in the prostate. The normal adult male plasma levels of some important steroids are summarized in Table 90–2. These values are derived as averages from numerous studies. Individual values can fluctuate with age, time of day, medications, stress, hospitalization, and environmental changes. There is evidence that overproduction of adrenal steroids can stimulate growth of the prostate gland. For example, in humans, abnormal virilism has been observed in immature males with a hyperfunctioning adrenal cortex. In rodents, overstimulation of the adrenals can also induce limited prostate growth even in the absence of testicular androgens. For example, administration of exogenous adrenocorticotropic hormone to castrated animals does significantly increase the growth of sex accessory glands (Tullner, 1963; Tisell, 1970; Walsh and Gittes, 1970). However, the effect of normal levels of adrenal androgens on the prostate in noncastrated humans and adult male rats does not appear to be significant because adrenalectomy has little effect on prostate size, DNA, or morphologic characteristics of the sex accessory tissue (Mobbs et al, 1973; Oesterling et al, 1986). Furthermore, after castration in animals, with the adrenals intact, the prostate will finally diminish to a very small size (90% reduction in total cell mass). Finally, the small involuted ventral prostate in the castrated rat cannot be significantly reduced further by additional adrenalectomy or hypophysectomy (Kyprianou, 1987). In castrated rats, the DHT level in the prostatic tissue is approximately 20% of that in normal intact animals. Adrenalectomy lowers the DHT to nondetectable levels without further diminution in prostate growth. This indicates that a threshold level of DHT is required in the prostate to stimulate growth and the castrate level is below this threshold. It has also been concluded similarly that the prostate of man does not restore itself after castration, indicating that adrenal androgens are insufficient to compensate for the loss of testicular function. Quantitative morphometry of the human prostate (Oesterling et al, 1986) also confirms that the adrenal gland has little effect on the epithelial cell size of the normal prostate. The adrenal steroids dehydroepiandrosterone (DHEA) and the conjugate dehydroepiandrosterone sulfate (DHEAS) as well as androstenedione are androgens synthesized from acetate and cholesterol (see Fig. 90–6) that are secreted by the normal human adrenal glands. Essentially all of the DHEA in the male plasma is of adrenal cortex origin, and the production rate in man is 10 to 30 mg/day. Less than 1% of the total testosterone in the plasma is derived from DHEA (Horton, 1976; MacDonald, 1976). The prostate and seminal vesicles of the rat and the human prostate can slowly hydrolyze DHEAS to free steroids through a prostate sulfatase enzymatic activity, but the degree of conversion is low; hence, DHEAS is not a potent androgen. A second adrenal androgen is androstenedione, and the plasma concentration in adult men is approximately 150 ± 54 ng/dL (see Table 90–2). The blood production rate of androstenedione in human males is 2 to 6 mg/day, with approximately 20% of the androstenedione being generated by peripheral metabolism of other steroids. Androstenedione cannot be converted directly to DHT. An important role for androstenedione in the male may be its peripheral conversion to estrogens through the aromatase reaction (see Fig. 90–6). The estrogen receptors (ERs) are differentially expressed in the prostate. In the mouse, ER-α is expressed early (1 week) in the stroma of the ventral prostate but by 2 weeks is preferentially expressed in the epithelia, and ER-α is absent altogether in the ventral prostate by 4 weeks. In contrast, ER-β exists in the epithelial compartment as the dominant ER by the fourth week. Interestingly, however, knockout mice for ER (both isoforms) are able to form grossly normal prostates, although fertility may be limited in the ER-α (Couse et al, 2001). Only small amounts of estrogen are produced directly by the testes. In the plasma of young healthy human males 75% to 90% of the estrogens are derived from the peripheral conversion of androstenedione and testosterone to estrone and estradiol through the aromatase reaction (see Fig. 90–6) (Horton, 1976; MacDonald, 1976). The androgenic C19 steroids (testosterone and androstenedione) are converted to the estrogenic C18 steroids first by removal of the 19-methyl group and followed by the formation of an aromatic or phenolic steroid A ring (aromatase reaction), present in both estradiol and estrone. Estradiol is formed from testosterone and estrone from androstenedione; these two estrogens are interconvertible. The daily production of estradiol in the human male is 40 to 50 µg, and only 5 to 10 µg (10% to 25%) can be accounted for by direct testicular secretion (see Table 90–2). Less than 2% of the total testosterone in human plasma is free or unbound; the remaining 98% is bound to several different types of plasma proteins (see Fig. 90–5). The plasma proteins that bind steroids include human serum albumin, sex hormone–binding globulin (SHBG or testosterone-estrogen–binding globulin [TeBG]), corticosteroid-binding globulin (also named transcortin), progesterone-binding globulin, and, to a lesser extent, α1-acid glycoprotein. Under normal conditions, the total amount of testosterone bound to progesterone-binding globulin and α1-acid glycoprotein is nominal and is usually ignored. The regulation of the amount of androgen that is free is an important physiologic variable and varies in different species. The total amount of steroid bound depends on two factors: (1) the affinity of the steroid to bind to a specific protein and (2) the capacity, which is the maximal potential binding when all of a binding protein is saturated with bound steroid; the capacity is governed by the amount of binding protein in the plasma. Serum albumin has a relatively low affinity for testosterone, but, given its abundance, it has a high capacity. In contrast, SHBG has a high affinity for binding steroids but the protein is present in relatively low concentrations; however, the plasma molarity of each binding protein exceeds the plasma molarity for total testosterone concentration. The majority of testosterone bound to plasma protein is associated with SHBG. For example, Vermeulen (1973) has calculated that in the normal human male 57% of testosterone in the plasma is bound to SHBG and 40% is bound to human serum albumin. Less than 1% is bound to corticosteroid-binding globulin, and only 2% of the total testosterone is free (see Fig. 90–5). The normal plasma free testosterone level is therefore 12.1 ± 3.7 ng/dL or 0.42 nM; this non–protein-bound “free testosterone” is bioavailable to diffuse into the sex accessory tissue and into liver cells for metabolism. In addition, a large percentage of the SHBG is saturated whereas only a small fraction of the total capacity of corticosteroid-binding globulin and albumin is used under normal conditions. As testosterone levels increase in the plasma, the order of increasing saturation of the plasma proteins proceeds from SHBG to corticosteroid-binding globulin to albumin. Therefore, the binding of androgen is a dynamic equilibrium between various serum proteins. The total plasma levels of SHBG can be altered by hormone therapy. Administration of testosterone decreases SHBG levels in the plasma, whereas estrogen therapy stimulates SHBG levels (Forest et al, 1968; Vermeulen, 1969; Burton and Westphal, 1972). Estrogen also competes with testosterone for binding to SHBG, but estrogen has only one third the binding affinity of testosterone. Therefore, administration of small amounts of estrogen increases the total concentration of SHBG, and this effectively increases the binding of testosterone and thus lowers the free testosterone plasma concentration. Because only free testosterone is bioavailable, the binding of testosterone to plasma proteins inhibits net testosterone uptake into the prostate (Lasnitzki and Franklin, 1972). It is apparent that androgenic activity is regulated in part by the extent of binding of an androgen to the steroid-binding proteins in the plasma. Key Points: Endocrine Control of Prostate Growth There are multiple levels of prostate growth regulation that include steroid hormone action, growth factors, and direct cell-cell communication and interactions with the extracellular matrix. These interactive types of growth control are accomplished by several generalized systems, as depicted in the schematic in Figure 90–7. They include the following: Testosterone in the serum arrives at the prostate bound to albumin and to the steroid-binding globulins. Free testosterone enters the prostate cell by diffusion, where it is then subjected to a variety of steroid metabolic steps that appear to regulate the activity of the steroid hormone and its downstream effectors. A simplified schematic of the temporal sequence of intracellular events is depicted in Figure 90–8 and includes the following:
Developmental and Cell Biology
Seminal Vesicles
Embryonic Development of the Seminal Vesicles
The Prostate
Regional Differentiation of the Lower Urinary Tract
Prostate Budding
Cytodifferentiation
Molecular Features of Prostate Development
Induction of Prostate Budding
Epithelial Budding
Genes Required for Prostate Epithelial Budding
Andromedins
Molecular Control of Branching Morphogenesis
Fibroblast Growth Factors
The Hedgehog (Hh) Signaling Pathway
The Transforming Growth Factor β (TGF-β) Superfamily
Prostate Zonal and Lobar Anatomy
Prostate Cell Types
COMPONENTS
PROPERTIES
Development
Seminal vesicles
From wolffian ducts through testosterone stimulation
Prostate
From urogenital sinus through dihydrotestosterone stimulation
Prostate Zones
Anterior fibromuscular
30% of prostate mass, no glandular elements, smooth muscle
Peripheral
Largest zone, 75% of prostate glandular elements, site of carcinomas
Central
25% of prostate glandular elements; surrounds ejaculatory ducts
Transitional
Smallest zone, surrounds upper urethra complex, sphincter
5% of prostate glandular elements, site of benign prostatic hyperplasia
15%-30% of prostate volume
Epithelial Cells
Basal
Small and flattened undifferentiated, nonsecretory cells with a low proliferative index (<1%) that express keratins 5, 14, and 18
Intermediate
Proliferating cell type that has characteristics intermediate between basal and secretory cells, including production of basal and secretory cell keratins
Columnar secretory
Terminally differentiated, nondividing, rich in acid phosphatase and prostate-specific antigen; 20-µm tall, most abundant cell, keratins 5, and 18
Epithelial Cells cont’d
Neuroendocrine
Terminally differentiated, nonproliferating cells that express serotonin, chromogranin-A, neuron-specific enolase, and synaptophysin proteins
Stromal Cells
Smooth muscle
Rich in α-actin, myosin, and desmin
Fibroblast
Vimentin rich and associated with fibronectin
Endothelial
Associated with fibronectin; alkaline phosphatase positive
Tissue Matrix
Extracellular matrix
Basement membrane
Type IV and V collagen meshwork that is laminin rich and supports basal cells, stem cells, transit-amplifying cells, and secretory epithelium
Connective tissue
Type I and type III fibrillar collagen; elastin
Glycosaminoglycans
Sulfates of dermatan, chondroitin, and heparin; hyaluronic acid
Cytomatrix
Tubulin, α-actin, and intermediate filaments of keratin
Nuclear matrix
Dynamic structure of the nucleus that directs the functional organization of DNA into loop domains; contains ribonuclear proteins
Luminal Epithelial Cells
Neuroendocrine Cells
Basal Cells
Prostate Epithelial Stem Cells
Intermediate Cells
The Stroma and Tissue Matrix
Endocrine Control of Prostate Growth
Androgen Production by the Testes
Adrenal Androgens
Estrogens in the Male
Androgen-Binding Proteins in the Plasma
Regulation of Prostate Growth by Steroids and Protein Growth Factors
Androgen Action at the Cellular Level
Stay updated, free articles. Join our Telegram channel
Full access? Get Clinical Tree
Development, Molecular Biology, and Physiology of the Prostate
• The prostatic epithelium in the human is composed of two major cell compartments: epithelial cells and stromal cells.
• Free testosterone in the plasma is converted in the prostate by 5α-reductase type 2 into dihydrotestosterone, which is 2 to 10 times more active than testosterone.
• Endocrine factors or long-range signals arriving at the prostate by serum transport of hormone originating from the secretions of distant organs; this includes serum steroid hormones such as testosterone and estrogens and serum peptide hormones such as prolactin and gonadotropins.
• Extracellular matrix factors, which are insoluble tissue matrix systems and make direct and coupled contact by being attached through integrins and adhesion molecules of the basal membrane and couple cytoskeleton organization with the extracellular matrix components, which include the glycosaminoglycans, such as heparan sulfate (Getzenberg et al, 1990)
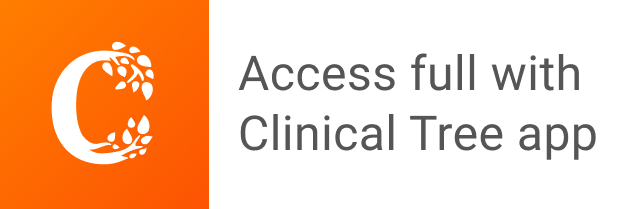