Fig. 6.1
Schematic diagram of the liver regeneration hypothesis using decellularized scaffolds. (a) Partial resection of one hepatic lobule is performed. (b) The defective part is replaced with decellularized liver scaffold. (c) Cells in the native liver cross the suture border and regenerate on the liver scaffold. (From Yu et al. [2])
6.2 Rationale for Decellularization
Xenogeneic and allogeneic cellular antigens are recognized as foreign by the host and therefore induce an inflammatory response or an immune-mediated rejection of the tissue. However, the components of ECM are generally conserved among species and are tolerated well, even by xenogeneic recipients [5, 6]. ECM from a variety of tissues, including heart valves [7, 8], blood vessels [9, 10], skin [11], nerves [12], skeletal muscle [13], tendons [14], ligaments [15], small intestinal submucosa [16], urinary bladder [17], and liver [18], has been reported for use in tissue engineering and regenerative medicine applications. The purpose of decellularization protocols, though minimizing adverse effects on the mechanical integrity of the remaining ECM, is to efficiently remove all cells and nuclear material (Fig. 6.2).


Fig. 6.2
Whole-organ scaffolds decellularized using perfusion. The native extracellular matrixes (ECMs) of cadaveric organs can be isolated by perfusion of the native vascular system with detergent solutions. The resulting scaffolds are acellular, but maintain the structure of the native organ. (a) (i) Rat heart scaffold generated from a cadaveric heart using perfusion decellularization. The ascending aorta was cannulated for perfusion. (ii) Cadaveric human heart before and after perfusion decellularization. (b) (i) Rat lung scaffold generated from cadaveric lung by perfusion decellularization. Perfusion was performed via the pulmonary artery. (ii) Cadaveric sheep lung before and after decellularization. (c) (i) Rat kidney scaffold generated from cadaveric kidney using perfusion decellularization. The abdominal aorta was cannulated for perfusion. (ii) Porcine kidney before and after perfusion decellularization. (d) (i) Rat pancreas scaffold generated from cadaveric pancreas by perfusion decellularization. The abdominal aorta was cannulated for perfusion. (ii) Human pancreas before and after perfusion decellularization. (From Song and Ott [19])
With the purpose of removing the cells, any of the processing steps changes the native three-dimensional structure of ECM. The most common method used for the decellularization of an organ involves a combination of physical and chemical processing. Physical treatment can include agitation or sonication, mechanical massage and pressure, or a freeze–thaw process. Using these methods, the cell contents are removed, the cell membrane is easily destroyed by subsequent rinsing and removal of the cell content from the ECM. However, although these physical processes achieve complete decellularization, they are insufficient, and must be combined with chemical treatment. Enzymatic treatments, such as trypsin, ionic detergents and chemical treatments, can disrupt charge coupling among the cell membrane, cell, and extracellular component. Tissue is composed of both cell materials, and ECM has a variable degree of miniaturization according to the tissue source. ECM is sufficient to allow proper exposure of all the cells to the chaotropic agents. To provide a path for removal from the organ, the cellular material must be destroyed during the decellularization process. The purpose of the main part of the decellularization process is to retain the original mechanical and biological properties and minimize disruption.
6.3 Decellularization Protocols
The most robust and effective physical decellularization protocol uses a combination of chemicals, and includes an enzymatic approach. Decellularization protocols, typically enzyme treatment, surface-active agents, cytoplasmic, solubilization of cellular components of the nucleus, and the final removal of cellular debris from tissue, cellular components from the ECM, involve physical treatment or ionic solution. Following separation, the dissolution of the cell membrane begins. To enhance effectiveness, these steps can be combined with mechanical stirring. Following decellularization, all the residual chemicals must be removed to avoid the response of chemically adverse host tissue. The efficiency of the storage of decellularization and ECM can be assessed using one of several methods.
6.3.1 Physical Methods
6.3.1.1 Temperature
The freeze–thaw process lyses the cells of the tissues and organs effectively; unless removed by subsequent processing, the resulting membranous and intracellular content remains. By using a single freeze–thaw cycle, a harmful immune response, such as leukocyte infiltration into the ECM scaffold, can be reduced [20]. Multiple freeze–thaw cycles may be used during decellularization [21] and do not significantly increase the loss of ECM proteins from the tissue [22]. Freeze–thaw processing does produce minor disruptions of the ECM ultrastructure [23] and should therefore be used only when such effects are acceptable in the final ECM product. The effect of freeze–thaw treatment on the mechanical, load-bearing properties is minimal for a mechanically robust organ [24].
6.3.1.2 Force and Pressure
Cells on the surface of a tissue or organ (e.g., urinary bladder, small intestine, skin, amnion) can be effectively removed using mechanical abrasion in combination with enzymes, hypertonic saline, or chelating agents, all of which facilitate the dissociation of cells from their subjacent basement membrane [23]. However, the integrity of the underlying hyperfine structure and the basement membrane can been damaged by direct applications of mechanical force. The formation of barium crystals may disrupt the ultrastructural hydrostatic pressure of the ECM and requires relatively little time compared with enzymes used to remove cells from detergents and blood vessels. It may also be effective in corneal tissue [25]. The temperature rises at the time of the pressure of decellularization, but to prevent the formation of crystals, it is possible to destroy the ECM because of the associated increase in entropy, which can be mitigated by a colloid such as dextran [26].
6.3.2 Chemical Methods
6.3.2.1 Acids and Bases
Acids and bases cause and catalyze the hydrolysis of biological molecules. Peracetic acid, a common disinfectant that by removing residual nucleic acids has a minimal impact on the composition and structure of ECM, also serving as a decellularization agent [27, 28].
Acetic acid damages and removes collagens, thus decreasing ECM strength, but sulfated glycosaminoglycans (sGAG) are not affected. Bases (e.g., calcium hydroxide, sodium sulfide, and sodium hydroxide) are used to remove hair from dermis samples during the early stages of decellularization [29].
6.3.2.2 Hypotonic and Hypertonic Solutions
Hypertonic saline dissociates DNA from proteins [30]. Hypotonic solutions can readily cause cell lysis because of simple osmotic effects, with minimal changes in matrix molecules and architecture [31]. For a maximum osmotic effect for dipping alternately hyper- and hypotonic solution through a few cycles in the organ is common. Hypertonic and hypotonic solution also helps to rinse the cell debris from within the organ after dissolution.
6.3.2.3 Detergents
Ionic, non-ionic, and zwitterionic detergents solubilize cell membranes, isolating DNA from the protein and removing cellular material effectively from the tissue [30]. However, these agents also disrupt and dissociate proteins in the ECM, as demonstrated by their use in the protein extraction procedures of tissue proteomics [22]. The removal of ECM proteins and DNA by detergents increases with exposure time [11] and varies with organ subunit, tissue type, and donor age [22]. Combining multiple detergents increases ECM protein loss [32], but also allows for more complete detergent removal from ECM after decellularization [33]. Triton X-100 can effectively remove cell residues from thicker tissues, such as valve conduits, where enzymatic and osmotic methods are insufficient, with concomitant ECM protein loss accompanied by a decreased adverse immune response in vivo [34]. Sodium dodecyl sulfate (SDS) appears to be more effective than Triton X-100 at removing nuclei from dense tissues and organs such as the kidney and temporomandibular joint, while preserving tissue mechanics [35].
6.3.2.4 Alcohols
Alcohol such as glycerol acid, decellularizes by dehydrating and lysing the cells [29]. Phospholipids in valve leaflets and conduits contribute to prosthesis calcification and failure and can be extracted using alcohols [36]. In fact, isopropanol, ethanol, and methanol are more effective than lipase at the removal of lipids from adipose tissue in a relatively short period of time [37]. Methanol has been used in combination with chloroform during tissue delipidation [29]. Caution should be used when treating tissues with alcohols such as ethanol and methanol because of their use as tissue fixatives in histology, their ability to precipitate proteins [38], and the damage they cause to the ECM ultrastructure [39].
6.3.3 Biologic Agents
6.3.3.1 Enzymes
Enzymes that have been reported in the decellularized protocol include nuclease, trypsin, collagenase, lipase, dispase, thermolysin, and α-galactosidase. Enzymes provide a high specificity for the removal of cell debris or undesirable ECM components. However, it is difficult to effect complete cell removal in a single enzyme treatment; enzyme residues impair recellularization, and can induce a harmful immune response. Nucleases (e.g., DNase and RNase) cleave nucleic acid sequences and thus help in the removal of nucleotides after cell lysis [26].
Endonucleases such as benzonase [40] may be more effective than exonucleases because they cleave nucleotides mid-sequence and thereby more effectively fragment DNA to prepare for its removal. Similarly, nonrestriction endonucleases fragment the DNA more effectively than sequence-dependent counterparts. Trypsin is a serine protease that is used as an enzymatic decellularization agent. However, ECM proteins such as collagens have limited resistance to trypsin cleavage [41], and tissue exposure to trypsin should therefore be used with caution. Trypsin is slower at cell removal than detergents. Elastin and collagen are removed from the cell, but although trypsin is more destructive, it does show better preservation of the glycosaminoglycan (GAG) content [42, 43]. Collagenase decellularization may be employed, but only if maintenance of the ultrastructure and of collagen is not critical to the intended clinical applications of the resulting ECM.
6.3.3.2 Nonenzymatic Agents
Ethylenediaminetetraacetic acid (EDTA) and ethylene glycol tetraacetic acid (EGTA) are chelating agents that aid cell dissociation from ECM proteins by sequestering metal ions [44]. Chelating agents are likely to contribute to subtle disruptions in protein–protein interactions using the same mechanism [45]. Chelating agents alone are not sufficient for superficial cell removal, even if agitated [23], and are thus usually used with enzymes such as trypsin [46] or with detergents [20]. The effectiveness of the chelate plus a simple hyper- or hypotonic solution for decellularization is unknown [20]. Toxins such as latrunculin enable the researcher to take advantage of generating a natural cytotoxic agent for decellularization. Gillies et al. [47] demonstrated removal of DNA and intracellular proteins from dense tissue, the tibialis anterior, using only latrunculin B, hyper- and hypotonic solutions, and DNase treatments. This method provided superior removal of DNA and retention of GAG to the enzymes and detergents for decellularization. The properties of passive mechanical testing of the ECM scaffold were similar.
6.4 Changes in the Mechanical Properties of Decellularized ECM During Constructive Remodeling
Facilitating the replacement of the ECM graft with functional host tissue is considered a constructive remodeling response. ECM scaffold changes during such a remodeling response are dependent on factors such as the local tissue microenvironment, the rate of scaffold degradation, forces present within the mechanical environment, and the rate and extent to which the infiltrating cells deposit new ECM [48, 49]. Decellularized scaffolds retain a variety of collagens, growth factors, glycosaminoglycans, and other matrix proteins, and the arrangement of the proteins is minimally disturbed through the process [50–53]. Upon implantation, mononuclear cells migrate into the decellularized scaffold and degrade it, while concomitantly depositing new site-appropriate tissue [54, 55]. The structural changes that occur during in vivo remodeling of ECM scaffolds are associated with marked changes in scaffold strength. In the early phase of remodeling, degradation occurs quite rapidly, before the newly deposited ECM can become fully organized. However, once the infiltrating cells have established residence and begin producing new site-specific ECM, the remodeling process progresses over time toward a tissue construct that has similar mechanical properties very similar to those of native tissue [54, 56].
6.5 Sterilization of Decellularized Scaffolds
Clinical application of a decellularized scaffold requires donor scaffold sterilization. The sterilization process primarily eliminates endotoxins and intact viral and bacterial DNA that may induce unwanted inflammation upon scaffold implantation [57]. Decellularized scaffolds may be sterilized using simple treatments such as incubation in acids [58] or solvents [59], but such methods may not provide sufficient penetration or may damage key ECM constituents [60]. Sterilization methods such as ethylene oxide exposure, gamma irradiation, and electron beam irradiation achieve effective sterilization, but are known to alter ECM microstructure and mechanical properties [61, 62].
6.5.1 Ethylene Oxide Exposure
Ethylene oxide sterilization does not affect cell attachment to ECM or the stimulation of growth factor secretion by fibroblasts in vitro [28]. Ethylene oxide may substantially change ECM mechanical properties [63] or leave them unaltered [24, 62], and ethylene oxide treatment can cause undesirable host immune responses that impair proper function of the biologic scaffold after implantation [64]. Therefore, the use of ethylene oxide for the sterilization of decellularized scaffolds should be considered carefully.
6.5.2 Gamma Irradiation Exposure
Degradation of ECM during gamma irradiation is at least partially attributed to spontaneous denaturation of key structural proteins such as collagen that occurs even at relatively low doses at body temperature [65]. As the irradiation dose increases, denaturation of collagen continues to increase, resulting in dose-dependent alterations in mechanical properties [66]. Even at a dose as low as 2 kGy, gamma irradiation increases tissue stiffness and tensile strength, but at doss higher than 15 kGy, mechanical properties decrease in a dose-dependent manner [67]. Gamma irradiation causes residual lipids to become cytotoxic and accelerates enzymatic degradation [67, 68].
6.5.3 Supercritical Carbon Dioxide
Supercritical carbon dioxide has become a mainstay of the sterilization of food and pharmaceuticals. It has recently been investigated as an alternative method of sterilizing decellularized scaffolds. Supercritical carbon dioxide is versatile for sterilization owing to its nonreactive nature, its ability to penetrate cells and tissues, its reduced energy usage, and its improved quality of retention of heat-sensitive substrates [69]. Supercritical carbon dioxide sterilization with peracetic acid sterilant causes only minor changes in the susceptibility of porcine acellular matrix to collagenase digestion, in tensile or tear strength, indicating limited alteration of the tissue structure following supercritical carbon dioxide sterilization [70]. However, supercritical carbon dioxide sterilization is relatively new and requires further investigation.
6.6 Effects and Evaluation of Decellularized Scaffolds
The major concerns of all decellularization protocols remain ECM disruption, immunogenicity, and thrombogenicity. The effectivity of decellularization and the alterations to the ECM vary according to the source, composition, and density of the tissue, and to other factors. Decellularization agents that are very efficient at removing cellular components can also cause damage to the collagen structure and remove growth factors and other important ECM components. However, because of insufficient decellularization, residual cellular components within ECM may contribute to in vitro cytotoxicity and adverse host responses in vivo upon reintroduction of cells [71–73]. There are conflicting results on the effect of decellularization techniques. Grauss et al. [74] found that chemically induced decellularization using Triton X-100 or trypsin resulted in changes in the ECM constitution, which could lead to problems in valve functionality and cell growth and migration, whereas Schenke-Layland et al. [75] used a decellularization method, in which pulmonary valves were dissected from porcine hearts, placed in a solution of trypsin-EDTA, incubated at 37°C for 24 h, followed by a 24-h wash in phosphate-buffered solution (PBS), and showed acceptable removal of cell components from a porcine pulmonary valve. Cartmell and Dunn [14] compared the methods of decellularization for rat tail tendon using three extraction chemicals (t-octylphenoxypolyethoxyethanol [Triton X-100], tri(n-butyl)phosphate [TnBP], and sodium dodecyl sulfate [SDS]). They showed that treatment of tendons with 1% Triton X-100 for 24 h disrupted the collagen fiber structure, but did not remove cells. Treatment with 1% SDS for 24 h or 1% TnBP for 48 h resulted in an acellular tendon matrix with retention of near-normal structure and mechanical properties. Woods and Gratzer [15] also compared the effect of decellularization using one of the three protocols incorporating the surfactant lauryl sulfate (SDS), Triton X-100, and/or an organic solvent, tri(n-butyl)phosphate (TnBP). They showed that all three treatments were effective at removing cells and preserving the mechanical properties of the graft, with subtle, but notable, differences. Most detergents, when used as agents for decellularization, cause some removal of GAG from the scaffold, which has varying degrees of a negative effect on the tensile viscoelasticity of the scaffold [76]. Another factor that can influence the mechanical properties of the scaffold is the duration of exposure to decellularization agents [77]. There is practically no consensus regarding the effects of any of the decellularization methods. Therefore, it is very important to optimize the decellularization method for obtaining acceptable cell removal according to the source, composition and density of the tissue, and other factors.
Evaluation of the residual materials and cellular components within the decellularized ECM is a pivotal step in translating them into a clinically practicable, transplantable substance. There are a number of methods available to determine the effectivity of the elimination of cellular material from tissues. Although the decellularization process cannot remove 100% of cell material, it is crucial in quantifying cellular components such as double-stranded DNA (dsDNA), mitochondria, or membrane-associated molecules such as phospholipids in a decellularized scaffold. Crapo et al. [57] suggested the following minimal criteria sufficient to satisfy the intent of decellularization: (1) no visible nuclei upon histological evaluation, (2) the remaining dsDNA content should not exceed 200 base pairs in length, and (3) the amount of dsDNA should not exceed 50 ng/mg of dry weight of the material. The first criterion is easily evaluated using standard histological staining with hematoxylin and eosin or immunofluorescent methods such as DAPI or Hoechst. The second and third criteria are easily evaluated using commercially available dsDNA intercalators, such as PicoGreen®, propidium iodide, or bisbenzimide, and by gel electrophoresis respectively. Jackson and Simon [78] demonstrated the fate of DNA from allografts after transplantation using a DNA probe technique that clearly distinguished donor cells from host cells and could also be utilized to evaluate whether any DNA is present in the decellularized scaffold in the Spanish goat model. Electron microscopic methods or polymerase chain reaction (PCR) are usable, but not typically used to evaluate the presence of residual nuclear material or cytoplasmic debris because of the technical complexity and expense of these procedures for routine work [79]. Schmitt et al. [80] studied the cell viability, cell migration parameters, and biomechanical properties of human adipose-derived stem cells following reseeding on human tendon scaffolds in vivo using bioluminescent imaging and immunohistochemistry. They showed that reseeded cells remained viable on the implanted constructs at up to 4 weeks using bioluminescent imaging, and histological evaluation showed host cell invasion and proliferation of the repair sites. Biomechanical testing revealed no significant difference in ultimate load to failure and a 2-mm gap force. This movement of host cells and human adipose-derived stem cells into the scaffold suggests the biointegration of the allograft. Lin et al. [81] evaluated the biocompatibility of the decellularized scaffolds using enzyme–detergent methods for the cell removal of mouse skeletal muscle tissue by histological staining (sections stained with H&E, Van Gieson’s, and DAPI) and DNA quantification. They showed that mouse skeletal muscles combined with an enzyme–detergent mixture (trypsin and Triton X-100) can yield an intact matrix devoid of cells, depleting more than 93% of the nuclear component and exhibiting comparable biomechanical properties to those of native tissue, and that infiltration of inflammatory cells increased into the scaffold initially and then decreased gradually until day 30. Although the above methods provide important information regarding the effectiveness of decellularization methods, the biologic consequences of small amounts of nuclear material or cytoplasmic debris within the decellularized scaffold are unclear. Crapo et al. [57] suggested that a standard for tissue decellularization might provide numerous benefits, including (1) allowing investigators and ECM product manufacturers to evaluate the effectiveness of a protocol when reporting new decellularization techniques or describing products composed of ECM derived from decellularized tissue; (2) enabling congruous comparison of different ECM products; (3) eliminating variations in cell and host responses to ECM products caused by variations in residual DNA, thereby facilitating interpretation and comparison of in vitro and in vivo results; and (4) promoting the rapid and effective development of additional clinical applications for ECM products within the field of regenerative medicine and tissue engineering.
6.7 Removal of Residual Chemicals
The decellularization methods involve a wide variety of chemicals used because of their capability to damage cells. The strong chemicals can thoroughly remove the cell components, but the internal fiber scaffold structure may also be seriously damaged, whereas mild chemicals can preserve the internal fiber scaffold structure. However, the removal effect of cell components is far from satisfying, which is the messy problem of chemical extraction. The presence of some residual decellularization agents within ECM biomaterials is toxic to host cells and may be responsible for discouraging cellular ingrowth [43, 82]. There is a need for the development of assays to quantify the presence of residual chemicals in the decellularized scaffold material and the removal methods of residual chemicals.
6.8 Eight Decellularized Scaffolds as a Platform for Bioengineered Organs
6.8.1 Liver
Liver tissue engineering provides an insight into liver regeneration; it has seen remarkable progress in recent years [83, 84]. In 2010, liver scaffolding of transferred and intact acellular liver has been developed by perfusion of various chemical detergents into the portal vein of the rat. These scaffolds maintain the function of the microvasculature and the three-dimensional structure of ECM components (Fig. 6.3) [84]. Scaffolding of decellularized liver has demonstrated the ability to efficiently support in vitro decellularization with subsequent perfusion of primary liver cells [85, 86]. In in vivo microinjection of scaffolds from decellularized liver with microscopic vascular anastomosis, the scaffold showed seeding cells. However, thrombosis formation was noticed shortly post-transplantation [87]. To address thrombus formation, heparin was perfused in the multilayer on the inside surface of the scaffold [88, 89]. Despite the short-term effectiveness of this intervention, long-term effectiveness requires further experiments.


Fig. 6.3
Fabrication, vascular cast, light microstructure, and implantation of the decellularized liver scaffolds. (a) Decellularization of a single lobe of rat liver progressing under continuous detergent perfusion. Scale bar 10 mm. (b) Decellularized whole-liver scaffold with the hepatic artery intact. Scale bar 20 mm. (c) Vessel corrosion casting of the microstructure of the hepatic portal vein (blue), the hepatic artery (red), and the hepatic duct (transparent). Scale bar 2 mm. (d) H&E staining of liver matrix shows the existence of blue-stained nuclei in intact liver, but not in (e) decellularized liver scaffold. (f) H&E staining results show the border between the liver parenchyma and the implanted decellularized scaffold. Scale bar 100 μm. (From Yu et al. [2])
6.8.2 Heart
The first decellularized cardiac scaffolds were produced from rats in 2008 [14]. These scaffolds were perfused with heart muscle cells and vascular endothelial cells in vitro to mimic the cell composition of the heart. This construct successfully ran the pump function after transplantation [90]. Induced pluripotent stem cells (iPSCs) of human origin were seeded onto decellularized mouse heart in vitro. The seeded iPSCs migrated, proliferated, and differentiated into functional cardiomyocytes after implanting, enabling the constructed cardiac tissues to demonstrate contractility [91].
Recently, these studies focused on the repair of myocardial infarction after myocardial ischemia. Bone marrow mesenchymal stem cells (MSCs) were promoted to cardiac repair after myocardial ischemia or infarction [92]. Transplantation of stem cells improves the tissue condition and overall cardiac function of infarcted tissue [93].
6.8.3 Lung
The study of lung tissue regeneration has passed through two stages. The basic concept of the regeneration of the lung stem cell is possible to play the lung tissue, to join the synthetic material and lung stem cells to build functional pulmonary units (alveoli). Based on such a proposal, lung stem cells were seeded in synthetic material in vivo and in vitro. The construct could not be formed, owing to the poor integration and tissue compatibility and the possibility of complete failure of the respiratory function caused by surgical infection [94, 95]. Recently, lung tissue engineering has been facilitated by decellularized scaffold in vivo and in vitro. Although the cellular components are removed during decellularization, the relevant cytokines and ECM are retained [96].
6.8.4 Kidney
Porcine kidneys have been successfully decellularized, proposing the possibility of using these transplantable scaffolds to construct tissue-engineered kidney that is clinically applicable (Fig. 6.4) [97]. Whole porcine kidneys were decellularized and then orthotopically transplanted in vivo, and then anticoagulant was administered as prophylaxis. Inflammatory cells in the pericapsular region and thrombosis occurred owing to the lack of endothelial cells [98]. In recent findings, successfully engineered renal tissue absorbed by the proximal tubule was shown to have the ability of metabolic and endocrine functions [99]. Yu et al. [4] successfully reported that renal decellularized scaffolds induce the regeneration of injured kidney (Fig. 6.5). There is a possibility that various cytokines in the scaffold play an important role in the recovery of postoperative renal function after partial nephrectomy.
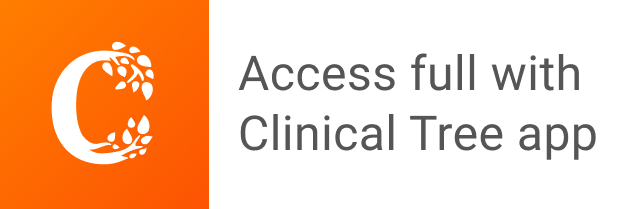