David C. Miller, MD, MPH, Louis Leon Pisters, MD, Arie S. Belldegrun, MD Historically, the principal treatment options for patients with clinically localized prostate cancer have included radical prostatectomy, radiation therapy, and active surveillance or watchful waiting (Middleton et al, 1995). With the exception of radical prostatectomy and watchful waiting (Bill-Axelson et al, 2005), however, randomized, prospective clinical trials comparing the efficacy of primary prostate cancer therapies are lacking. Radical prostatectomy has been perceived as morbid by some, whereas radiation therapy has been criticized for its uncertain efficacy (Scardino and Wheeler, 1988; Kabalin et al, 1989; Fowler et al, 1993; Crook et al, 2000; Potosky et al, 2000, 2004; Zelefsky et al, 2001; Pollack et al, 2002; Penson et al, 2005). Active surveillance is an early option for some patients; however, these patients often proceed with definitive local therapy during longer-term follow-up (Koppie et al, 2000). These and other concerns regarding conventional treatment have fueled an interest, among both patients and physicians, in alternative treatments that are both efficacious and associated with limited treatment-related morbidity. In fact, cryotherapy—the ablation of tissue by local induction of extremely cold temperatures—has re-emerged as a therapeutic modality that meets these criteria. In 1996 the American Urological Association (AUA) recognized cryotherapy as a therapeutic option for prostate cancer and removed the “investigational” label from this procedure. In late 2008 the AUA released a consensus “Best Practice Statement” on cryosurgical ablation as a treatment option for patients with clinically localized prostate cancer (Babaian et al, 2008). The consensus panel concluded that cryosurgery is an established treatment option for men with newly diagnosed, or radio-recurrent, organ-confined prostate cancer. Moreover, the consensus panel concluded that advances in cryotherapy technology including ultrasonographic ice ball monitoring and use of multipoint thermal sensors have allowed more efficient freezing of the prostate gland while reducing collateral damage to surrounding tissues, notably, the rectum, urethra, and external urinary sphincter (Babaian et al, 2008). Accordingly, contemporary cryotherapy offers patients a minimally invasive treatment option with low morbidity, minimal blood loss, short hospital stay, good recurrence-free survival, and high rates of negative post-treatment biopsies (Shinohara et al, 1996; Koppie et al, 1999; Long et al, 2001; Bahn et al, 2002; Donnelly et al, 2002; Han et al, 2003; Prepelica et al, 2005; Cohen et al, 2008; Jones et al, 2008; Pisters et al, 2008). Human cancers were first managed with cryotherapy in the 19th century when cervical and breast cancers were treated with a salt and ice mixture (Gage, 1998). Although the method was crude, tumor size could be reduced and local tumor control was an attainable goal. In 1961 Cooper and Lee built the first closed cryoprobe system, which circulated liquid nitrogen through a closed metal tube that was placed in contact with the tissue to be treated. This system was used for treating patients with Parkinson disease and other neuromuscular disorders. The first urologic application of prostate cryotherapy was by Gonder and colleagues (1964), who developed probes suitable for transurethral freezing of prostate tissue for benign prostatic hyperplasia and prostate cancer. As of 2008, four distinct generations of cryotherapy for prostate cancer have been described, highlighting both the progressive evolution of relevant technology and improved patient selection (Table 105–1). During the 1960s and 1970s, early cryosurgeons applied both transurethral and open transperineal approaches in their attempts to eradicate prostate cancer (Gonder et al, 1964). In 1972 Flocks and colleagues described a refined transperineal approach. An incision was made anterior to the rectum, allowing separation of the rectum and prostate and exposure to the most posterolateral aspects of the prostate gland. Freezing was then performed on the surface of the gland and monitored by direct vision. Although the technique compared favorably with treatment modalities such as radical prostatectomy and external-beam radiation therapy (EBRT) with respect to survival, morbidity was significant and included urethral sloughing, rectourethral or urethrocutaneous fistulae, bladder neck obstruction, and urinary incontinence (Bonney et al, 1982). In 1974 Megalli and colleagues were the first to report a transperineal percutaneous approach using a single 6.3-mm (18-Fr), sharp-tipped liquid nitrogen cryoprobe (Megalli et al, 1974). This cryoprobe was introduced through perineal skin incisions performed on either side of the midline, and its placement was guided by digital palpation. The digitally monitored freezing process was often augmented by a single thermostat placed in the perirectal region. The cryoprobe was typically repositioned to achieve adequate coverage of the prostate, seminal vesicles, and any identifiable extraglandular extension. In an attempt to improve monitoring, Reuter (1972) described the use of a rigid cystoscope, as an adjunct to the rectal index finger, to directly monitor the bladder and ureteral orifices. Unfortunately, the inability, with first-generation cryotherapy, to precisely place cryoprobes and monitor the extent of freezing in real time resulted in the abandonment of the technology until the late 1980s (Gonder et al, 1964; Flocks et al, 1972). The development and implementation of transrectal ultrasound (TRUS) guidance and urethral warming heralded the second generation of prostate cryotherapy and significantly reduced the number of treatment-related complications. Onik and colleagues (1984) first demonstrated that the frozen area could be visualized ultrasonographically as a well-marginated, hyperechoic rim with acoustic shadowing, and that the leading edge of the growing iceball could be monitored in real time with TRUS imaging (Onik et al, 1988). In the 1990s TRUS technology achieved widespread adoption for the early detection of prostate cancer, and this trend fueled the development of a transperineal, percutaneously introduced, multiple cryoprobe system (Chang et al, 1994). TRUS guidance allowed accurate placement of cryoprobes, real-time monitoring, control of freezing, and visualization of the rectum to protect it from injury. At the same time, the use of urethral warmers with a continuous irrigation system maintains the urethral mucosa above freezing and preserves a thin layer of the mucosa. Urethral warming has been credited with significantly reducing urethral sloughing, stricture formation, and urinary incontinence (Cohen and Miller, 1994; Cohen et al, 1995; Wong et al, 1997; Lee et al, 1999). Another significant advance for modern prostate cryotherapy was the development of cryogenic systems with multiple cryoprobes. Multiple, simultaneously controlled cryoprobes achieve a uniform and effective distribution of lethal temperature throughout the iceball, thereby increasing clinical efficacy and reducing the risk of collateral injury. In addition, the availability of multiple cryoprobes provides surgeons with greater flexibility to conform ice formation to each patient’s unique anatomy. During this same time period, the emergence and widespread adoption of the prostate-specific antigen (PSA) assay allowed for better selection of patients and more precise assessment of postcryotherapy cancer control outcomes (Stamey et al, 1987). The first modern cryoprobe system was a liquid nitrogen system that supported independent throttle control of five cryoprobes (Accuprobe System, Cryomedical Sciences, Inc., Rockville, MD) that delivered high-efficacy freezing. An advancement of the system over earlier liquid nitrogen systems was refrigeration (“supercooling”) of the liquid nitrogen from its usual boiling point at below −195.8° C down to −209° C. This improved efficiency by overcoming problems with nitrogen bubbles forming in the cryoprobe transport tubes or in the cryoprobes, which could unpredictably impede liquid nitrogen cryogen circulation. The first clinical application of such a system in humans was in 63 patients with localized prostate cancer (Onik et al, 1993). Although essential to the successful reintroduction of cryotherapy, liquid systems have intrinsic difficulties in containing, controlling, and circulating liquid nitrogen. Therefore the hallmark of third-generation cryotherapy was the transition from liquid to gas systems. With this technologic advance, surgeons could now use pressurized gas to both freeze (argon gas) and actively warm (helium gas) tissue via the Joule-Thompson effect. According to the Joule-Thompson effect, different gases undergo unique temperature changes when depressurized according to unique gas coefficients (Fig. 105–1). In the case of argon gas, this is a constant enthalpy expansion that results in rapid cooling to its boiling point of argon gas (−185.7° C). To accomplish this, high-pressure (3000 psi) ambient temperature argon gas is circulated to the cryoprobe tip, where it expands rapidly as it decreases to room pressure (15 psi). The expanded gases are circulated back to the cryogenic unit through the larger outer lumen of the cryoprobe and the supply hose. The venting of used gas, usually into the room, occurs at the cryogenic machine. The use of helium gas (67° C), which warms up rather than cools when expanded, provided an active defrosting capability that was not available in the second-generation systems, improving the control of iceball formation. It is noteworthy that both argon and helium are inert gases and therefore safe. However, both will displace oxygen from a room and thus adequate air circulation should be maintained within the cryogenic suite. Importantly, the transition from liquid to gas allowed for the development of ultra-thin, 17-gauge (1.47-mm) cryoprobes, which permitted direct transperineal needle placement through a template without making incisions or using tract dilatation and insertion kits (Fig. 105–2). Another advantage is that gas systems are more compact and easier to use and maneuver within the operating room environment. The introduction of pinpoint thermocouples for systematic temperature monitoring has also substantially contributed to a reduction in cryotherapy-associated morbidity. They are used to ensure both that adequately cold temperatures are reached within the prostate and that sensitive adjacent structures, namely, the rectum and external sphincter, are maintained at temperatures warm enough to ensure maintenance of their structural and functional integrity. In addition, the risk of rectal freezing can be lessened by injection of saline solution into Denonvilliers fascia, increasing the space between the prostate and the rectum. The development of multipoint thermal sensors marked the emergence of fourth-generation prostate cryosurgical technology. With multipoint sensors, surgeons are now able to monitor temperatures along an entire plane (e.g., between the prostate and rectum), rather than at isolated anatomic sites. Although empiric data are not yet available, it is anticipated that the routine utilization of multipoint thermal sensors will further reduce the incidence of postcryotherapy morbidity including both fistulae and urinary incontinence. Fourth-generation technology also involves the use of newly introduced, variable-length cryosurgery probes. The principal advantage of variable-length probes is that they allow surgeons to conform the iceball to the length of the prostate under treatment, thereby obviating the need for a pullback procedure in a majority of cases. Finally, the newest generation of cryosurgical technology also includes computer software that allows surgeons to construct preoperative temperature maps on the basis of theoretic cryoprobe placement (Babaian et al, 2008). With these computer models, surgeons are able to prospectively plan cryoprobe configuration in an attempt to further optimize targeting of diseased tissue, while minimizing damage to adjacent important structures including the rectum, urethra, and external urinary sphincter (Babaian et al, 2008). The principles of cryotherapy including the mechanisms of cell injury and cell death have been well studied (Gonder et al, 1964; Gage and Baust, 1998, 2007; Hoffman and Bischof, 2002; Baust and Gage, 2005; Baust et al, 2007) (Table 105–2). Unlike radiation (mitotic arrest) or androgen deprivation (apoptosis), the main mechanism of cryotherapy-produced cytotoxicity is the induction of targeted areas of coagulative necrosis in the prostate gland. The key factors involved in freezing injury include direct mechanical shock, osmotic shock, and cellular hypoxia. Mechanisms of action include protein denaturation via dehydration, transfer of water from the intracellular space to the extracellular space, rupture of cell membranes from ice crystal expansion, toxic concentration of cellular constituents, thermal shock from rapid supercooling, slow thawing, vascular stasis, and increased apoptosis (Cooper and Hirose, 1966; Hoffman and Bischof, 2002; Baust and Gage, 2005; Baust et al, 2007; Gage and Baust, 2007). Table 105–2 Cytotoxic and Antineoplastic Effects of Cryotherapy The extracellular fluid begins to crystallize after reaching a tissue temperature below 0° C. The osmotic pressure of the unfrozen portion of the extracellular fluid compartment increases, causing dehydration of cells to occur due to the shift of water from the intracellular to the extracellular space, which also results in an accumulation of toxins within the cell (Hoffman and Bischof, 2002; Baust and Gage, 2005; Baust et al, 2007; Gage and Baust, 2007). Changes in cellular pH lead to the denaturing of cellular proteins. At a temperature of −15° C, most of the extracellular environment is frozen, which causes trapping of tissue and shearing forces to disrupt cellular structure (i.e., mechanical shock) (Hoffman and Bischof, 2002; Baust and Gage, 2005; Baust et al, 2007; Gage and Baust, 2007). Further decreases in temperature lead to crystallization of water in the intracellular space that mechanically breaks the cellular membrane (Hoffman and Bischof, 2002; Baust and Gage, 2005; Baust et al, 2007; Gage and Baust, 2007). The delayed or indirect destructive effects of cryotherapy continue primarily because of injury to the vasculature, resulting in tissue hypoxia and vascular thrombosis (Hoffman and Bischof, 2002; Chao et al, 2004; Baust and Gage, 2005; Baust et al, 2007; Gage and Baust, 2007). Zacarian and colleagues (1970) reported that at temperatures below −20° C, venules were more susceptible to injury than arterioles. Freezing promotes stasis of blood, leading to thrombosis and subsequent coagulative necrosis of tissue. This process includes local edema with activation of the inflammatory cascade. On thawing, extracellular fluid shifts back into the intracellular space, leading to lysis of both the intracellular organelles and the cell membrane itself. The blood vessels around the targeted tissue initially dilate after thawing, and hyperpermeability of the vessel wall occurs. After a few hours of this hyperemic state, microthrombi form on the damaged vessel wall, leading to regional tissue ischemia (Hoffman and Bischof, 2002; Baust and Gage, 2005; Baust et al, 2007; Gage and Baust, 2007). Apoptosis is recognized as a mechanism of cell death that occurs in both normal tissues and various pathologic conditions. Apoptotic cells are characterized by nonrandom DNA cleavage, blebbing of membranes, phospholipid inversion in the membranes, and caspase activation (Kerr et al, 1994). Following cryosurgical ablation, apoptotic cells are found primarily in the peripheral zone of the cryogenic lesion outside the killing zone, where the temperature was not sufficiently cold to kill all the cells (Hollister et al, 1998; Hoffman and Bischof, 2002). In addition, kinetic studies have shown that cells are susceptible to entering the apoptotic state up to 8 hours after rewarming (Yang et al, 2003). The recent finding of apoptosis in cells in the peripheral zone of the cryogenic injury may one day be exploited therapeutically using other proapoptotic stimuli to extend the zone of cell death (Yang et al, 2003; Clarke et al, 2007). Currently, however, the precise role of apoptosis in cryosurgery-induced tissue injury remains incompletely understood (Hoffman and Bischof, 2002; Baust et al, 2004). In-vivo experiments have suggested that cryodestruction of living tissues can elicit an immunologic response by the host that may itself eradicate cancer cells (Ablin, 1972). Experiments with MRMT-1 mammary adenocarcinoma cells and R3327 prostate adenocarcinoma cells in rats have demonstrated the appearance of tumor immunity by 7 to 10 weeks after cryotherapy that was associated with a decrease in growth of metastatic lesions. However, cryotherapy of the Dunning AT-1 cell line, an androgen-insensitive cell line, in Copenhagen rats was associated with a minimal increase in T-cell counts without an effect on the local tumor growth rate (Hoffmann et al, 2001). Although the potential for a cryoimmunologic effect against residual prostate cancer cells is intriguing, cryotherapy must still be considered as a local therapy. The response of human tissue to freezing injury varies from inflammatory to destructive, depending on the severity of freezing (Hoffman and Bischof, 2002; Baust and Gage, 2005; Baust et al, 2007; Gage and Baust, 2007). Minor freezing injury features only inflammatory responses, whereas severe freezing injury destroys cells and tissues, which is the prime requirement for treating tumors. The cryogenic lesion is characterized by a central region of uniform coagulation necrosis. Surrounding the central lesion is a peripheral zone that develops several days after freezing (Fig. 105–3). In this region, tissue temperatures ranged 0° C to −0° C, and only partial cell death has occurred (Hoffman and Bischof, 2002; Baust and Gage, 2005; Baust et al, 2007; Gage and Baust, 2007). The process of wound repair begins in the peripheral zone in the areas in contact with viable tissue; inflammatory cells infiltrate and new blood vessels may grow into the injured tissue. Over the following weeks or months, the dead tissue is slowly replaced by fibroblasts and new collagen formation, yielding a contracted healed area in lieu of the previously ablated tissue. Optimal technical application of cryotherapy requires a thorough understanding of the freeze-thaw cycle and its components (Table 105–3). The variables that correlate with the likelihood of cell destruction include the cooling rate during freezing, the warming rate during thawing, and the lowest temperature achieved in the tissue (Hoffman and Bischof, 2002; Baust and Gage, 2005; Baust et al, 2007; Gage and Baust, 2007; Babaian et al, 2008). Tatsutani and colleagues (1996) described these effects in an in-vivo prostate cancer cell experiment. In this study, complete cell death was unlikely to occur at temperatures higher than −20° C and temperatures lower than −40°C were required to completely destroy cells (Tatsutani et al, 1996). Moreover, the investigators noted that a faster freezing rate and a slower thawing rate resulted in optimal cellular destruction. Table 105–3 Factors Affecting Tissue Destruction during Cryotherapy For optimal results, a tissue temperature of −40° C to −50° C, which is lethal for cells, should be produced within the tumor including a safe distance around the tumor (Bischof et al, 1997; Gage and Baust, 1998; Hoffmann and Bischof, 2002; Baust and Gage, 2005; Baust et al, 2007; Gage and Baust, 2007). In addition to the nadir temperature, the duration of freezing appears to be an important determinant of tissue destruction (Hoffman and Bischof, 2002; Baust and Gage, 2005; Baust et al, 2007; Gage and Baust, 2007). Once the tissue temperature reaches a certain nadir level, more cellular destruction can be achieved by prolonged freezing at this temperature. The target tissue should therefore be held in the frozen state for several minutes to allow time for solute effects, ice crystal formation, and recrystallization effects. When the tissue is held at −15° C to −40° C, biochemical changes and growth of ice crystals are enhanced, increasing the rate of cell death (Hoffman and Bischof, 2002; Baust and Gage, 2005; Baust et al, 2007; Gage and Baust, 2007). Nonetheless, the optimum duration of freezing is not well defined and may prove to be cell/tissue-type specific (Hoffman and Bischof, 2002; Baust and Gage, 2005; Baust et al, 2007; Gage and Baust, 2007). Slow thawing of the frozen tissue is also a crucial determinant of tissue destruction (Hoffman and Bischof, 2002; Baust and Gage, 2005; Baust et al, 2007; Gage and Baust, 2007). In fact, the rate of thawing should be as slow as clinically practical. A longer thaw will yield greater cellular damage due to solute effects, ice crystal restructuring (recrystallization), prolonged oxidative stress, and growth of ice crystals (Hoffman and Bischof, 2002; Baust and Gage, 2005; Baust et al, 2007; Gage and Baust, 2007). The large ice crystals that form during “warming” recrystallization create shearing forces, thereby disrupting the target tissues and promoting cellular death. Repetition of the freeze-thaw cycle is also important (Hoffman and Bischof, 2002; Baust and Gage, 2005; Baust et al, 2007; Gage and Baust, 2007; Babaian et al, 2008). The first freeze-thaw cycle increases the thermal conductivity of the tissue caused by cellular breakdown; the second freeze-thaw subjects the tissue to extended physiochemical changes, while passing through damaging thermal conditions for the second time (Hoffman and Bischof, 2002; Baust and Gage, 2005; Baust et al, 2007; Gage and Baust, 2007). With repetition, the second cycle increases the extent of necrosis up to 80% of the previously frozen volume (Hoffman and Bischof, 2002; Baust and Gage, 2005; Baust et al, 2007; Gage and Baust, 2007). The second cycle also moves the lethal isotherm close to −20° C, permitting a closer approach to the margins of the prostate without endangering the rectum. The interval between freeze-thaw cycles is also an important factor in tissue injury. A longer thaw time supports complete vasoconstriction of larger blood vessels, continued ice crystal growth, and more extensive osmotic damage. A further delay after thawing, but before the second freeze cycle, leaves the tissue in a hypothermic state and allows time for the microcirculation to fail. In combination, these events provide the targeted tissue with conditions consistent with severe oxidative stress that may ultimately give rise to additive post-thaw cell death (Hoffman and Bischof, 2002; Baust and Gage, 2005; Baust et al, 2007; Gage and Baust, 2007). In a clinical setting therefore the number of freezing cycles, the lowest temperature achieved, and the existence of any regional “heat sinks” may be important factors relating to cancer destruction. Double freeze-thaw cycles during cryotherapy for prostate cancer in humans have been associated with lower positive biopsy rates, as well as improved PSA results, when compared with a single freeze-thaw cycle, without an increase in the development of cryotherapy-related morbidities (Shinohara et al, 1996; Pisters et al, 1997; Wong et al, 1997; Mouraviev and Polascik, 2006; Babaian et al, 2008). Temperature mapping studies performed in patients with localized prostate cancer, who agreed to undergo focal cryotherapy of the prostate before undergoing radical prostatectomy, demonstrated that cryotherapy resulted in a central zone of complete cellular necrosis surrounded by a more peripheral zone of cell damage, and that two consecutive 10-minute freeze cycles produced a larger area of coagulative necrosis than a single 20-minute freeze (Larson et al, 2000). Furthermore, a double freeze at temperatures below −40° C appears to yield more complete tissue necrosis (Larson et al, 2000). This fact has important clinical implications because the hyperechoic edge of the iceball visualized is 0° C to −2° C, and temperatures as low as −20° C to −40° C are inside this edge (see Fig. 105–3). Therefore the iceball should be extended beyond the edge of the prostate to ensure adequate tissue ablation. Although the available literature supports the use of a minimum of two freeze-thaw cycles (Shinohara et al, 1996; Pisters et al, 1997; Wong et al, 1997; Mouraviev and Polascik, 2006; Babaian et al, 2008), it remains unknown whether additional therapeutic efficacy may be derived from more than two treatment cycles. Finally, cryosurgeons should be aware that large blood vessels may act as heat sinks and highly vascular areas may not achieve target temperatures even though they are completely enclosed in the treatment area. Recognizing the critical importance of these technical concerns and determinants of tissue destruction, authors of the AUA’s Best Practice Statement for prostate cryosurgery recommended that the following procedural requisites be considered in every case (Babaian et al, 2008): One of the most significant advances in the application of prostate cryosurgery has been the implementation of real-time, ultrasound-guided placement of the cryoprobes and continuous sonographic monitoring of the freezing process. Biplanar transrectal ultrasound systems (TRUSs) allow for transverse and longitudinal views of the prostate, and the views are easily interchangeable during the procedure. Frozen tissue is significantly different from unfrozen tissue in sound impedance, resulting in strong echo reflection at the interface of frozen and normal tissue. Accordingly, the leading edge of frozen tissue is easily visualized as a bright line (Fig. 105–4). The tissue inside this leading edge is concealed within the acoustic shadow created by this boundary so that the anterior boundary of the freezing area cannot be monitored (Mouraviev and Polascik, 2006). The refraction and reflection of the sound wave can also overestimate the lateral boundary of the iceball. The use of color Doppler imaging may be helpful for monitoring tissue viability (vascularity) as freezing approaches the rectum. Although not an objective parameter, color Doppler imaging provides some indication about the vascular state between the ice and the rectal mucosa and may therefore potentially provide some information about the likely speed of freezing in this area. Sonography provides no information about the temperature distribution within the ice, nor does it show the extent of freezing at the lateral or anterior aspects of the prostate. Reports of three-dimensional ultrasound during cryosurgery show potential, especially for preoperative planning of a procedure and cryoprobe placement (Chin et al, 1998). In practice, however, these have been relatively slow and cumbersome to apply, making their clinical implementation difficult. A brachytherapy-like stepper includes a cradle to which the multifrequency biplanar TRUS probe is attached. A brachytherapy-like template drilled with a matrix of holes that will accommodate the cryoprobes is then attached to the holding device (Fig. 105–5). Systems are available with a floor stand or table-mounted device. The brachytherapy-like grid can be adjusted to ensure accuracy with any ultrasound system. Liquid nitrogen (−195.8° C) is the coldest practical cryogenic agent and has the greatest freezing capacity, but it cannot be used in probes of less than 3 mm in diameter. The transition from systems circulating liquid nitrogen to gas-driven probes now permits the use of ultra-thin cryoprobes. In accordance with gas coefficient and nozzle dimensions, different gaseous elements generate different thermal exchange events (Joule-Thompson principle). In clinical practice, argon gas is used for cooling and helium gas is used for heating (thawing). Third-generation cryotherapy using gas-driven delivery systems and 17-gauge cryoprobes inserted via a direct transperineal route into the prostate without using an insertion kit were first reported in 2000 (Zisman et al, 2000). At present in the United States, there are two available systems for cryosurgical ablation of the prostate. The Precise system (Galil Medical, Plymouth Meeting, PA) uses pressurized argon gas as the source of freezing, and up to twenty-five 17-gauge (1.47-mm) cryoprobes (IceSeeds) can be used to create a conformal freezing pattern. The iceball created with the IceSeeds is approximately 18 mm in diameter and 27 mm in length. The Precise system also has available the IceRod needles in which a bigger iceball is created (32 mm × 56 mm), thereby facilitating treatment of men with larger prostate glands. The CRYOcare system (Endocare, Inc., Irvine, CA) also uses pressurized argon gas as a cryogen and can freeze up to eight 2.4-mm, vacuum-insulated cryoprobes simultaneously; the iceball created is approximately 33 mm in diameter and 54 mm in length. An important recent advance is the introduction of variable-length cryotherapy probes that tailor the length of the iceball to the length of the prostate. The authors anticipate that, in the future, cryotherapy probes may be further modified to also allow intraoperative tailoring of the iceball width. Because fairly reliable coagulative necrosis can be achieved when temperatures below −40° C are reached in the target tissues (Gage and Baust, 1998; Hoffman and Bischof, 2002; Baust and Gage, 2005; Baust et al, 2007; Gage and Baust, 2007), many surgeons now place thermocouples at the margins of the targeted treatment zones to ensure that cytotoxic temperatures are achieved during freezing. Several studies reported improved PSA recurrence-free rates and postcryotherapy biopsy results with thermocouple monitoring when compared with patients in whom this had not been used (Lee et al, 1997; Wong et al, 1997). In most cases, thermocouples are also used to ensure maintenance of nonablative temperatures within Denonvilliers fascia, the anterior rectal wall, the external sphincter, and/or the neurovascular bundle(s). The primary role for the urethral warming device during prostate cryotherapy is to protect the urethra and the external urinary sphincter to minimize urethral sloughing and prevent urinary incontinence. The urethral warmer is a closed double-lumen catheter made of a polyethylene membrane through which saline heated to 43° C is continuously circulated by a water pump at a rate of 350 to 500 mL/min. The first urethral warming system was marketed as a nonsignificant risk device until approximately July 1994. Initial experiences with this system were notable for fairly low rates of urethral sloughing, ranging from 4% to 10% (Onik et al, 1993; Coogan and McKiel, 1995; Wieder et al, 1995). Because of concerns submitted to the U.S. Food and Drug Administration (FDA) surrounding the safety of this particular device, it was taken off the market in mid-1994 for approximately 18 months while it underwent regulatory review. During this time period, numerous investigators reported a sharp increase in postcryotherapy transurethral resection of the prostate (TURP) and overall urethral sloughing rates due to the use of alternative urethral warmers or warming systems that did not have many of the features of the original one (Cohen et al, 1996a, 1996b; Lee et al, 1997). As expected, the safety of the original warmers was confirmed and, since 1996, FDA-approved urethral warming devices have been widely available. Studies directed toward improving catheter design and urethral warming efficacy are ongoing, with the hope that slough rates will decline even further as new devices are marketed. Moreover, because urethral warming catheters necessarily preserve a ring of viable tissue around the urethra, further improvements in the efficiency of these devices may allow surgeons to achieve a tighter treatment margin by reducing the diameter of viable periurethral tissue. This may, in turn, yield improved cancer control following cryosurgical ablation as preserved prostate tissue around the urethra represents a sanctuary site for residual and/or recurrent cancer. Computer-guided systems now provide improved intraoperative treatment planning with a potential for more precise and complete tissue destruction. The thermal distribution is predictable around a cryoprobe and can be modeled using computer simulation (Rewcastle et al, 1998, 2001; Babaian et al, 2008). Similar to the treatment planning strategies used for radiation therapy, it is possible to determine optimal placement of cryoprobes in the prostate to maximize ablation of the prostate while minimizing collateral damage (Sandison, 2002; Babaian et al, 2008). Research and development are focusing on the incorporation of functional and multimodality imaging data, as well as on the development of accurate mathematic models for in-vivo tissue injury response to thermal history (including cryotherapy-induced ischemia) to optimize treatment planning. Before proceeding with cryosurgery, patients with a high risk of metastatic disease should undergo imaging, radionuclide bone scan, or cross-sectional imaging of the abdomen and pelvis on the basis of the stage and grade, as well as serum PSA concentrations. Patients at high risk for lymph node metastases may undergo regional lymph node dissection if identification of disease would alter treatment choice. Patients with gross extracapsular extension or seminal vesicle invasion are treated with neoadjuvant hormone therapy to reduce the tumor volume and allow for easier inclusion within the iceball. If the prostate size exceeds 50 cm3, complete freezing of the prostate may be difficult and neoadjuvant hormone therapy is indicated to reduce the target volume and allow uniformly cold temperatures throughout the gland (Bales et al, 1995; Miller et al, 1996; Shinohara et al, 1996; Baust et al, 1997; Cespedes et al, 1997; Pisters et al, 1997; Babaian et al, 2008). There are no data, however, that neoadjuvant or concurrent androgen deprivation therapy influences postcryosurgery cancer control outcomes (Babaian et al, 2008). Nearly one third of men with newly diagnosed prostate cancer are treated with EBRT and/or brachytherapy as primary therapy each year (Mettlin et al, 1998, 1999). Despite advances in radiation therapy techniques including image-guided and intensity-modulated radiation therapy (IMRT), three-dimensional conformal radiation therapy (3DCRT), and image-guided implantation of electromagnetic sensors to improve treatment planning (Pisansky, 2006), urologists will continue to see men with recurrent prostate cancer following primary radiation therapy (Allen et al, 2007; Nguyen et al, 2007). In fact, depending on failure definitions and initial cancer severity, it estimated that between 20% and 60% of men who undergo initial definitive radiation therapy for prostate cancer may develop a postradiotherapy PSA recurrence (Allen et al, 2007; Nguyen et al, 2007); among this group, local-only failure rates range from 25% to 32% (Touma et al, 2005). Radioresistant or radio-recurrent prostate cancer is typically an aggressive form of localized prostate cancer that represents a serious health risk for patients treated with primary radiation therapy for clinically localized prostate cancer. Many patients with radio-recurrent disease harbor large-volume and/or poorly differentiated tumors at the time of diagnosis (Allen et al, 2007; Nguyen et al, 2007), making it a challenge for salvage therapies to fully eradicate the cancer. Early detection with serum PSA monitoring and prostate needle biopsy after primary radiation therapy may identify residual adenocarcinoma at an earlier stage and increase the likelihood of successful salvage therapy (Allen et al, 2007; Nguyen et al, 2007). The goal of salvage therapy is to provide a treatment option with curative intent that improves local control and possibly affect long-term survival (Babaian et al, 2008). Radical prostatectomy, cryotherapy, brachytherapy, and radiation therapy are the options available for salvage treatment of radiorecurrent prostate cancer (Touma et al, 2005; Allen et al, 2007; Nguyen et al, 2007). Salvage prostatectomy is associated with significant morbidity and therefore used relatively infrequently, particularly among older men with radio-recurrent prostate cancer (Krupski et al, 2006). Salvage brachytherapy and/or radiation therapy confer the potential for increased rectal and urinary toxicity in a patient who has previously received prostate irradiation (Allen et al, 2007; Nguyen et al, 2007). As a result, patients are often left with the option of either watchful waiting or temporary palliation with hormone deprivation therapy and its attendant toxicity. Notably, recent modifications in the technique of salvage cryotherapy have led to the ability to eradicate radiorecurrent prostate cancer safely and with decreased morbidity (Spiess et al, 2006; Ismail et al, 2007; Ng et al, 2007). However, complications associated with salvage cryotherapy are higher than those associated with primary treatment (Bales et al, 1995; Pisters et al, 1997; Long et al, 1998; Perrotte et al, 1999; Han et al, 2003; Spiess et al 2006; Ismail et al, 2007; Ng et al, 2007). Only patients with a rising PSA value after radiation, a positive postradiation biopsy, and a negative metastatic survey are candidates for salvage cryotherapy (Mouraviev et al, 2006; Babaian et al, 2008). The authors’ practice has been to wait at least 18 to 24 months after radiation therapy and to perform a biopsy of the prostate if the PSA value rises above the nadir level and there are three consecutive rises. Patients with a postradiation PSA greater than 10 ng/mL and/or a PSA doubling time of 16 months or less have been shown to be more likely to have unsuccessful salvage cryotherapy (Spiess et al, 2006; Ng et al, 2007; Babaian et al, 2008). If a biopsy is undertaken, multiple cores should be obtained and the pathologist needs to be informed that the patient has had previous radiation. Severe radiation effects with both nuclear and cytoplasmic alterations are seen in many prostatic biopsies and may confound the diagnosis of residual cancer (Cheng et al, 1999). Given the high prevalence of seminal vesicle involvement in series of patients undergoing salvage radical prostatectomy, consideration should also be given to performing concurrent seminal vesicle biopsies (Babaian et al, 2008). The incidences of positive biopsy results after primary radiation therapy vary widely in the literature but seem to be higher after EBRT than after brachytherapy (Stone et al, 2000). Once it has been determined that primary therapy has failed, local recurrences must be distinguished from systemic recurrences. Local failure has been defined as histologically proven active adenocarcinoma on repeat prostate biopsy in the absence of radiographic evidence of metastatic disease. Unfortunately, multiple studies have demonstrated the relative lack of sensitivity and specificity of most radiographic tests including computed tomography (CT), magnetic resonance imaging (MRI), bone scan, and, more recently, monoclonal antibody-labeled nuclear scans for the diagnosis of systemic disease in biochemically recurrent prostate cancer (Albertsen et al, 2000; Sodee et al, 2000). High-resolution MRI with a lymphotropic superparamagnetic nanoparticle imaging agent may offer greater sensitivity for the detection of lymph node metastases; however, this technology is not yet available in clinical practice (Harisinghani et al, 2003; Nguyen et al, 2007). Despite the shortcomings of currently available imaging techniques, these modalities should be applied if recurrence is suspected because the presence of overt metastatic disease may obviate the patient being exposed to unnecessary local therapies. In this setting, pathologic confirmation via prostate biopsy of locally recurrent disease is warranted before consideration of invasive salvage therapies. In rare cases, there may be a role in performing an open or laparoscopic biopsy of the pelvic lymph nodes because between 20% and 40% of patients will have lymph node metastases (Rogers et al, 1995; Babaian et al, 2008). Caution is advised for novice laparoscopic surgeons because the dissection of these nodes can be technically challenging owing to potential adherence to the pelvic side wall and external iliac vessels. Relative contraindications to cryotherapy are similar to those for brachytherapy and include prior TURP with a large tissue defect, significant symptoms of urinary obstruction before treatment, large prostate size, and a history of abdominoperineal resection for rectal cancer, rectal stenosis, or other major rectal pathology (Babaian et al, 2008). Prior TURP is associated with an increased risk for sloughing and urinary retention (Babaian et al, 2008). Absolute contraindications include fistulae for any reason (including but not limited to inflammatory bowel disease) and previous surgery or trauma to the pelvis (e.g., urethroplasty, open book fracture) that may distort the anatomy of the prostate.
History of Cryotherapy
Cryobiology
Mechanisms of Cell Injury and Death by Cryotherapy
Mechanical
Biochemical
Ischemic
Apoptotic
Immunologic
Tissue Response to Freezing
Factors Affecting Tissue Destruction during Cryotherapy
Technical Improvements in Cryotherapy Equipment
Transrectal Ultrasound Systems
Template and Stands
Cryogenic Systems
Thermocouples
Urethral Warming Device
Computerized Planning
Patient Selection
Primary Cryotherapy
Salvage Cryotherapy
Contraindications
Stay updated, free articles. Join our Telegram channel
Full access? Get Clinical Tree
Cryotherapy for Prostate Cancer
1. Tissue freeze rate: The authors concluded that rapid freezing is more efficacious than slow freezing with respect to cell death (Babaian et al, 2008).
2. Temperature monitoring: The consensus panel strongly recommended the use of thermal couples during cryosurgical ablation, despite the absence of clear scientific evidence to support their use. It is believed that real-time temperature monitoring allows the cryosurgeon to better control the freezing process while at the same time protecting key vital structures (e.g., rectum, urethra) from unintended injury (Babaian et al, 2008).
4. Thaw rate: The authors concluded that prostate cancer ablation is more effective with slow (passive) versus rapid (active) tissue thawing. They note, however, that active thawing may not affect thaw rates at the distal edges of the gland (Babaian et al, 2008).
5. Freeze cycles: Members of the panel endorsed the use of a double freeze-thaw cycle during cryosurgical ablation of the prostate. They based this recommendation on experimental and clinical evidence that cancer cells not killed by the first freeze cycle may be stressed to such an extent that a second cycle achieves complete cellular death (Babaian et al, 2008).
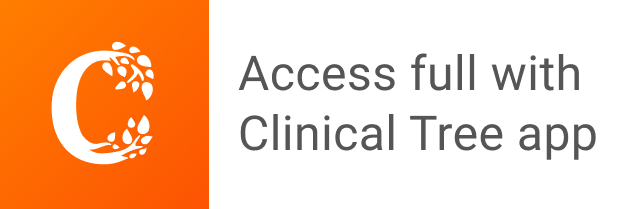