MODALITIES OF CONTINUOUS RENAL REPLACEMENT THERAPY—TECHNICAL CONSIDERATIONS
Terminology
Continuous therapies encompass a variety of modalities that vary in the terminology. Recently, an international group of experts has developed the Acute Dialysis Quality Initiative (ADQI) (6) and has proposed adopting previously developed standardized terms for these therapies. The basic goal was to link the nomenclature to the operational characteristics of the different techniques (7). Each letter in the acronym represents a specific characteristic related to the duration, driving force, and operational features. The letter C is used in all the terms to describe the continuous nature. The letters AV or VV in the terminology identify the technique’s driving force, that is, the mean arterial pressure (MAP) for arteriovenous (AV) circuits and external pumps for venovenous (VV) circuits. Solute removal in these techniques is achieved by either convection, diffusion, or a combination of both these methods. Therefore, the letters UF, H, HD, and HDF identify the technique’s operational characteristics:
Convective techniques, including ultrafiltration (UF) and hemofiltration (H), depend on solute removal by solvent drag (8).
Diffusion-based techniques, similar to intermittent hemodialysis (IHD), are based on the principle of a solute gradient between the blood and the dialysate (9).
Hemodiafiltration (HDF) processes use both diffusion and convection in the same technique. In this instance, both dialysate and a replacement solution are used, and both small and middle molecules can be removed easily (10).
The only exception to this nomenclature system is the acronym SCUF (slow continuous ultrafiltration), which designates UF alone without any fluid replacement.
TYPES OF CONTINUOUS RENAL REPLACEMENT THERAPY MODALITIES
As discussed in section “Terminology”, the predominant mechanism of achieving clearance defines the modality of CRRT.
Simple diffusion: continuous venovenous hemodialysis (CVVHD)
Convection: continuous venovenous hemofiltration (CVVH)
Combination of both [continuous venovenous hemodiafiltration (CVVHDF)] (TABLE 10.1 and FIGURE 10.1)
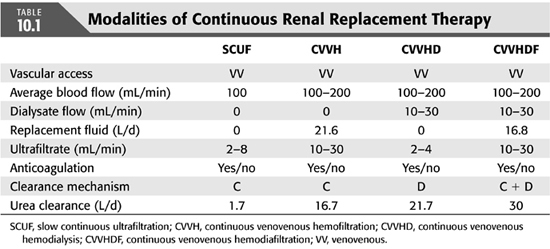
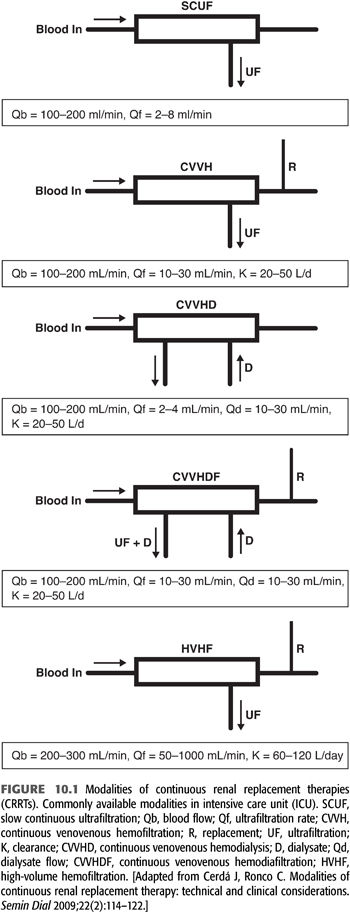
MACHINES AND COMPONENTS OF CONTINUOUS RENAL REPLACEMENT THERAPY
Modern CRRT machines are designed specifically for acute dialysis and are equipped with a friendly user interface to perform SCUF, CVVH, CVVHD, and CVVHDF. These new machines utilize preset disposables, have integrated safety alarms, fluid balancing controls, and connected blood modules that are controlled with sophisticated microprocessors in the machine. The ease of setting wide range of blood, dialysate/replacement flows as well use of highly permeable membranes and pressure sensing systems has improved clearances of large molecules and efficiency of the treatment (11).
VASCULAR ACCESS AND CIRCUIT FOR CONTINUOUS RENAL REPLACEMENT THERAPY
A well-functioning vascular access is a prerequisite for successful CRRT. Several options now exist for access and can be selected based on individual patient requirements, local availability of the various catheters, and unit expertise. AV fistulas and grafts are generally not recommended for CRRT due to the risk for needle displacement and high risk for injury to the access that may make it unusable in the future. If an AV fistula or graft is to be utilized, it should be for a very limited time and preferably cannulated with plastic angiocatheters to minimize risk of complications.
Temporary or tunnelled cuffed hemodialysis (HD) catheters are currently used as vascular access for CRRT. Temporary catheters are generally inserted at bedside ideally under ultrasound guidance (USG) by modified Seldinger technique into internal jugular (IJ), femoral, or subclavian veins using stringent sterile precautions. Although tunneled, cuffed, double-lumen silastic catheters are the preferred access for short- and intermediate-term use in chronic dialysis patients, they are generally not the initial choice for CRRT as they are more difficult to place (12). However, if CRRT continues for longer than a week, ideally a tunnelled catheter should be placed for improving blood flow and reducing the risk of infections.
ADQI recommends use of USG for catheter placement as its use has been reported to reduce the failure and complication rates of central venous catheter insertion in level II and III studies. A single-center study (13) and meta-analysis (14) showed that USG reduces placement failure, complications, and need for multiple attempts.
Optimal site for catheterization in any given patient is determined by the risks of thrombosis and infection, ease of placement, and adequacy of function. The right IJ vein is preferred for temporary catheter, given its more direct route to superior vena cava. The left jugular vein has a more circuitous route to the right atrium, and this can lead to inadequate blood flow in patients with frequent neck movements. Femoral veins should be used as second choice for CRRT, given their ease of accessibility. Subclavian veins cannulation is discouraged, as it may lead to stenosis of the vessel and may interfere with the functioning of an AV fistula or graft that maybe required in the future (15).
Most clinicians do not consider catheter location to be a determinant of risk of infection, as results of various studies are conflicting. A recent meta-analysis has not shown any difference in the rate of catheter-related bloodstream (CRB) infections between the three sites (16). Another prospective study showed that frequency of catheter-related bacteremia was similar in jugular and femoral cannulation. The main risk factors for development of CRB were duration of catheter use and the number of performed dialyses (17). A prospective study by Oliver et al. (18) in 218 patients concluded that IJ catheters may be left in place for up to 3 weeks without a high risk of bacteremia, but femoral catheters in bed-bound patients should be removed after 1 week. Other characteristics, which may influence infection rates, include the catheter material and antimicrobial coating or impregnation. Polyurethane catheters are preferred for CRRT. A prospective randomized study showed that use of polyurethane HD catheters impregnated with minocycline and rifampin decreases the risk of catheter-related infection (19). Another prospective study proved the efficacy of catheter-restricted filling using an antibiotic lock solution in preventing CRB (20). But toxicity, allergic reaction, and bacterial resistance are the concerns associated with antibiotics limiting their widespread use. Recently, trisodium citrate (TSC) has been advocated as a catheter lock solution because of its antimicrobial properties and local anticoagulation. In a multicenter, double-blind, randomized controlled trial (RCT); TSC 30% was compared with unfractionated heparin 5,000 U/mL for prevention of catheter-related infections, thrombosis, and bleeding complications. The study was stopped prematurely because of a difference in catheter-related bacteremia (p <0.01) (21). After this trial, many studies have shown safety and superiority of TSC over heparin (22,23).
Besides infection, catheter malfunction due to thrombosis, kinking of catheter, fibrin sheath around catheter tip is another complication associated with CRRT. Instillation of heparin into both lumens prevents formation of intraluminal thrombus. However, in a completely occluded catheter by thrombus, recombinant tissue plasminogen activator (rtPA)/alteplase can be instilled to reestablish blood flow. In a study by Daeihagh et al. (24), tissue plasminogen activator (tPA) used in a dose of 2 mg with median dwell time of 24 hours appears to be as effective as urokinase for reestablishing adequate blood-flow rates through HD catheters that are thrombosed or have low blood-flow rates. tPA was effective in establishing adequate blood-flow rates (≥200 mL/min) during the next dialysis session in 87.5% cases. Another study also showed that Reteplase installation in dysfunctional HD catheters with dwell until the next HD session was effective in restoring catheter function in 87% of episodes. A dose of 1 U appears to be as effective as 4 and 6 U (25). Fibrin sheaths that form outside the catheter are resistant to thrombolytics and may require mechanical brushing or stripping (26).
ANTICOAGULATION FOR CONTINUOUS RENAL REPLACEMENT THERAPY
As in other extracorporeal circuits, anticoagulation is essential to prevent the activation of clotting mechanisms within the circuit (27,28) and is necessary for effective delivery of CRRT. The choice of anticoagulant should be based on multiple factors, including (a) the access site; (b) the nature and geometry of the membrane; (c) whether enhancements for UF, such as predilution, are used; and (d) the clinical status of the patient and preexisting coagulation abnormalities. The ideal anticoagulant should provide optimal antithrombotic activity with minimal bleeding complications and negligible systemic side effects. It should be inexpensive, have a short half-life, and be easily reversed (29). Adequate anticoagulation ensures efficacy of the filter in fluid and solute removal, overall filter longevity, and optimum patient management. When the anticoagulation is insufficient, filtration performance deteriorates and the filter may eventually clot (30), contributing to blood loss. Excessive anticoagulation, on the other hand, may result in bleeding complications, which have been reported to occur in 5% to 26% of treatments (31).
Several methods of anticoagulation are now available, and the key features of the most common methods are summarized in TABLE 10.2.
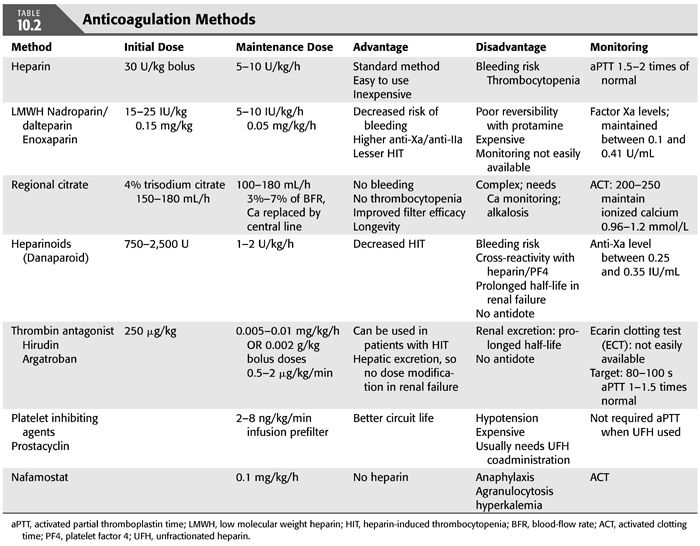
Unfractionated Heparin
Unfractionated heparin (UFH) continues to be the most commonly used anticoagulant. It catalyses the inactivation of thrombin, factor Xa and factor IXa by antithrombin. The main advantage of UFH is the large experience, the short biologic half-life (90 minutes but can increase up to 3 hours in presence of renal insufficiency), the availability of an efficient inhibitor, and the possibility to monitor its effect with routine laboratory tests. Limitations of UFH includes time- and dose-dependent unpredictable pharmacokinetics, resulting in considerable inter- and intrapatient variability (32), resistance to heparin due to reduced level of antithrombin III in renal insufficiency, high incidence of bleeding, and heparin-induced thrombocytopenia (HIT) (33–36). Dosing of heparin and target activated partial thromboplastin time (aPTT) varies in different units with the aim of maintain aPTT 1.5 to 2 times of normal. UFH is usually administered as a bolus of 30 IU/kg (2,000 to 5,000 IU), followed by a continuous infusion of 5 to 10 IU/kg/h into arterial limb of the dialysis circuit. Circuit survival time ranges from 20 to 40 hours. In view of the potential side effects of heparin, alternative methods of anticoagulation have been investigated, including regional heparin/protamine, low molecular weight heparins, heparinoids, thrombin antagonists (hirudin and argatroban), regional citrate, prostanoids, and nafamostat, with regional citrate anticoagulation (RCA) gaining wider acceptance with the development of simplified and safer protocols.
Low Molecular Weight Heparin
Recently, low molecular weight heparins (LMWHs) have been shown to be safe and effective drugs for anticoagulation of CRRT circuit (37–40). These agents have higher anti-Xa/anti-IIa activity than UFH (32), less protein binding, more predictable pharmacokinetics, and lesser incidence of HIT (41). The LMWHs are potent agents with renal excretion, have an increased half-life compared with UFH, poor reversibility with protamine because of stronger anti-Xa effect, more expensive than UFH, and also require specialist laboratory monitoring. Nadroparin, dalteparin in a dose of 15 to 25 IU/kg as bolus followed by 5 to 10 IU/kg/h (39–40), and enoxaparin as 0.15 mg/kg and a maintenance infusion starting at 0.05 mg/kg/h are the agents used in various studies (38).
Regional Citrate
Citrate (C6H7O7) is a small negatively charged molecule with a molecular weight of 191 Da. The use of citrate as anticoagulant in HD was first described by Morita et al. (42) in 1961 and in CRRT circuit by Mehta et al. in 1990 (43). Since then, various studies have shown better filter life and less bleeding with citrate in varied patient population including severe sepsis, liver failure, cardiac surgery, and both adults as well as pediatric patients population (36,44–61). In a prospective randomized multicenter trial by Hetzel et al. (62), citrate was found to have less systemic anticoagulation, a lower risk of bleeding, and a longer hemofilter patency. Some studies have shown evidence of improved biocompatibility by decreasing thrombogenicity and low polymorphonuclear cell degranulation (63–65), while effects of citrate on complement activation are not uniform (66–68). A recent study by Schilder et al. (69) has not shown any difference in mortality and renal outcome between RCA and UFH, but citrate was superior in terms of safety, efficacy, and costs.
Citrate is infused into the blood at the beginning of the extracorporeal circuit which chelates ionized calcium and provides anticoagulation in the circuit. Optimal regional anticoagulation occurs when the ionized calcium in the postfilter circuit is kept below 0.35 mmol/L. Calcium-citrate complex which is formed in the circuit is freely filtered and is lost in effluent, so calcium is infused systemically to replace the lost calcium to keep the ionized calcium within normal limits and infusion is titrated accordingly. Citrate which enters the body is metabolized by tricarboxylic acid pathway in liver, kidney, and skeletal muscles, and each citrate molecule yields three bicarbonate molecules, thereby avoiding systemic anticoagulation. Patients with severe liver failure and lactic acidosis may have difficulty in metabolizing citrate and may develop citrate toxicity, which is characterized by increased total to ionized calcium ratio, metabolic acidosis, and high anion gap (55,70–73). Other complications which may occur during RCA are hypernatremia particularly with hypertonic sodium citrate and metabolic alkalosis when too much citrate enters the circulation (43); therefore, frequent monitoring of electrolytes, acid–base status, and ionized calcium is required.
Various protocols for RCA have been described in literature (36,44–60). The ideal CRRT protocol should provide volume control, metabolic (acid–base and electrolyte) control, and adequate solute clearance, without significant complications related to bleeding or clotting. TABLE 10.3 shows CRRT prescription and citrate protocol at University of California, San Diego (UCSD). FIGURE 10.2 shows CRRT circuit using RCA.
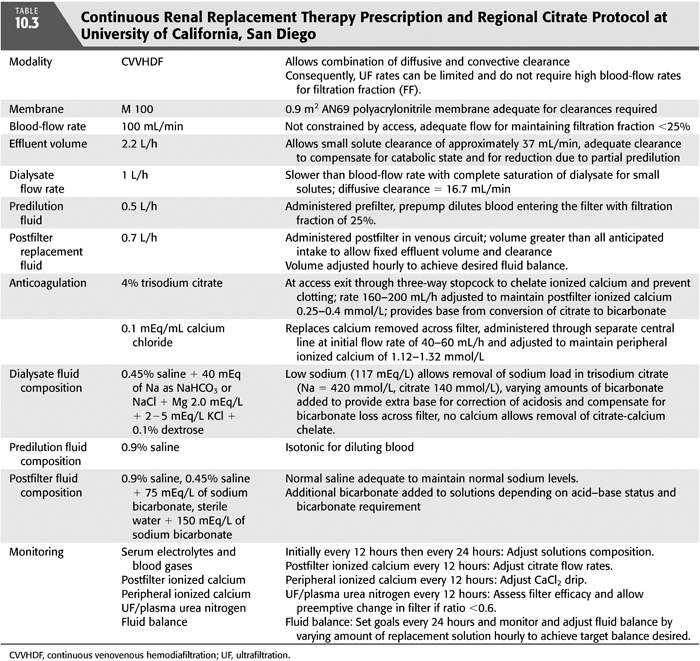
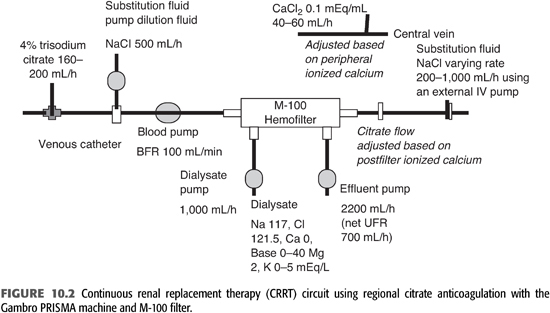
Other methods for anticoagulation in CRRT include regional heparin/protamine (37,74), heparinoids (75), thrombin antagonists [hirudin (76–78) and argatroban (79–83)], and platelet-inhibiting agents (prostacyclin and nafamostat) (84–91). Each of these techniques has unique advantages and disadvantages, and anticoagulation for CRRT should be adapted to the patient’s characteristics and institution’s experience. Doses, advantages, and disadvantages of these agents are summarized in TABLE 10.2.
CONTINUOUS RENAL REPLACEMENT THERAPY SOLUTIONS
All CRRT other than SCUF and CVVHD require the use of replacement fluids to compensate for the ultrafiltrate removed. HD and HDF techniques in addition use a dialysate. The composition of these solutions can be varied extensively to achieve specific metabolic goals (92). Commercially prepared sterile fluids are now available as premixed dialysis and replacement solutions for CRRT. Fluid can also be manufactured at the level of pharmacy/hospital, but manufacturing or customization is labor intensive, more expensive, have short shelf life, and is prone for human error (93,94).
TABLE 10.4 shows the composition of the most commonly used solutions for replacement and dialysate.
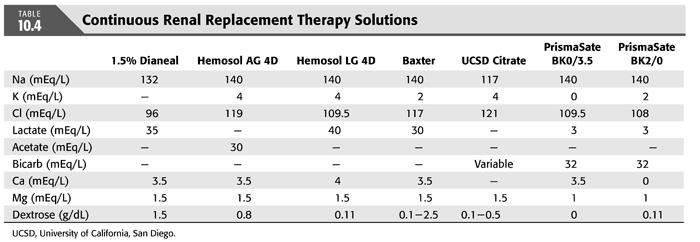
A consequence of all CRRT methods is the ongoing loss of bicarbonate and electrolytes across the filter generally equivalent to the plasma concentration of these solutes times the total effluent (UF and dialysate) flow rates (9). Bicarbonate losses can be replaced by addition of sodium bicarbonate or other base (e.g., lactate, acetate, citrate) to replacement solution administered intravenously, addition of base to the dialysate, or by a combination of these techniques (95). One of the major advantages of CRRT is that the composition of replacement fluid and dialysate can be modified to achieve any specific change in plasma composition.
Fluid Composition
Alkali
The choice of alkali depends on the clinical situation and availability. Both lactate and bicarbonate can correct metabolic acidosis as lactate is metabolized to bicarbonate by the liver.
Lactate-Based Solutions
Available lactate concentrations range from 28 to 49 mM. When base solutions other than bicarbonate are used, replacement of buffer stores depends on the metabolic rate for conversion to bicarbonate. In the absence of lactic acidosis, endogenous lactate clearance does not appear to be impaired. However, the filter clearance of lactate accounts for only 2.4% of overall lactate clearance (96). Lactate levels are generally higher with lactate solutions and might confuse the interpretation of blood lactate levels. Whether this hyperlactatemia is associated with morbidity is not clear. Potential concerns are hemodynamic compromise (96) or increased urea generation (97). Additionally, the ability to convert lactate to bicarbonate might be impaired in the setting of hypotension and multiorgan failure and could contribute to the deleterious effects of lactate accumulation (97). Hyperlactatemia can become pronounced during high-volume CRRT. “Lactate intolerance” is arbitrarily defined as a 5 mmol/L or more rise in serum lactate levels during CRRT.
Bicarbonate-Based Solutions
Bicarbonate concentrations are typically 22 to 35 mM and are preferred in patients with lactic acidosis and/or liver failure (98). One practical issue with the use of bicarbonate-based solutions is that it is difficult to store premixed bicarbonate solutions. A study by Kanagasundaram et al. (99) concluded that sustained bacterial contamination of bicarbonate-based CVVHD is common and could relate to the completeness of dialysate circuit change. Another factor to consider is that premixed solution containing calcium and bicarbonate shows evidence of microprecipitation of calcium carbonate crystals. Although some centers have developed nonsterile bicarbonate solution for CRRT, this practice is not recommended (99,100).
The combination of citrate anticoagulation and bicarbonate-containing solutions has been used effectively to manage complex acid–base disorders (43–59). At our center in UCSD, the hospital pharmacy prepared customized solutions for CRRT. Standard formulation at UCSD uses 1 L of 0.45% saline, to which is added 40 mL of 23% saline (yielding a sodium concentration of 117 mEq/L and a chloride concentration of 121.5 mEq/L), 1.5 mEq/L of magnesium, and 0 to 5 mEq/L of potassium. We use a dialysate dextrose concentration of 0.1% (43). In some circumstances, we add bicarbonate to the dialysate, substituting the 23% NaCl with NaHCO3 so that the final concentration of sodium is 117 mEq/L. More recently, secondary to nationwide drug shortages of bicarbonate, we switched to commercial calcium dialysate and replacement fluids and have not found any difference in filter performance.
ADQI recommends that lactate is an effective buffer in most CRRT patients. Bicarbonate is preferred in patients with lactic acidosis and/or liver failure and in high-volume H. When citrate is used as anticoagulant, no other buffer should be administered, but monitoring of pH is required.
Electrolytes
The replacement fluid and/or dialysate should contain electrolytes in concentrations aiming for physiologic levels and taking into account preexisting deficits or excesses and all inputs and losses. Customized solutions will be required in patients with some electrolyte imbalances, but these fluids should be used with caution, as accidental alteration is infusion rates can lead to significant electrolyte derangements. Therefore, frequent monitoring of serum electrolytes is imperative during CRRT. Most commercially available solutions do not contain phosphate but prolonged use of CRRT is associated with hypophosphatemia. Several methods are used to manage this problem including enteral feed with high phosphorus concentrations, intravenous supplementation with sodium phosphate (101). Recently, the U.S. Food and Drug Administration (FDA) approved Phoxillum Renal Replacement Solutions (BK4/2.5 and B22K4/0) as a replacement solution for CRRT to correct electrolyte and acid–base imbalances. The drug can also be used in the case of drug poisoning when CRRT is used to remove dialyzable substances. This product from Baxter is the only FDA-approved premixed solution including phosphate in a 5-L bag. (See more at http://www.pharmacytimes.com/product-news/fda-approves-new-solution-for-continuous-renal-replacement-therapy)
MODALITIES OF CONTINUOUS RENAL REPLACEMENT THERAPY—CLINICAL CONSIDERATIONS
Principles of Continuous Renal Replacement Therapy
Solute removal in CRRT can be obtained by convection, diffusion, or both. Each component of the therapy can be precisely controlled, and by use of high-flux membrane filters, removal of middle and high molecules can be maximized.
Diffusion
Diffusion is movement of solutes across concentration gradient through semipermeable membrane and depends upon
Concentration gradient (Cs)
Surface area of membrane (A)
Thickness of membrane (Mt)
Diffusion coefficient of the solute (D)
Temperature of solution (T)
The gradient is affected by the dialysate infusion rate (Qd) and blood-flow rate (Qb). The dialysate runs countercurrent to the blood, so faster Qb allows for greater gradient. This is the same technique used in IHD, but Qd are much slower than Qb so there is complete saturation of the dialysate. Therefore, the Qd is the rate-limiting factor for solute removal, but it allows for enhanced clearance. The smaller the size/weight of the solute and the greater the gradient, the more efficiently solute clearance occurs.
Diffusion Flux of a Given Solute Sd = (Cs / Mt) D · T · A
Convection
Convective techniques (UF and H) rely on what is known as “solvent drag,” whereby dissolved molecules are dragged along with ultrafiltrated plasma water across a semipermeable membrane driven by a transmembrane pressure gradient (TMP) in response to a hydrostatic or osmotic force. The process of forcing a liquid against a membrane is called ultrafiltration; the fluid collected after it passes through a membrane is the ultrafiltrate. This can be described by the following equation (EQUATION 10.1):

Kf = coefficient of hydraulic permeability
where Pb is hydrostatic pressure of blood, Puf is the hydrostatic pressure of the ultrafiltrate or dialysate, and π is the oncotic pressure of plasma proteins (EQUATION 10.2).

The convective clearance (Cx) of a solute x will therefore depend on the following (EQUATION 10.3):

Jf = amount of ultrafiltration
Cs = concentration of solute in plasma water
S = sieving characteristics of membrane
Sieving coefficient (S) is the ratio of solute concentration in filtrate to solute concentration in plasma and is regulated by the reflection coefficient of the membrane (S = 1 − σ). A solute with an SC of 1 means that it can pass freely through a filter; if the SC is 0, then a solute cannot pass through the filter at all. Protein-bound solutes or those that exceed the molecular weight cutoff (generally 20,000 Da for polysulfone and polyacrylonitrile membranes) have sieving coefficients less than 1.
Solute Clearances
In all forms of CRRT, the “effluent” from the filter represents the end product of the filtration process and comprises the ultrafiltrate in CVVH, the spent dialysate in CVVHD, and the combination of the ultrafiltrate and spent dialysate in CVVHDF. Consequently, filter clearance (UF × V/P) for most CRRT circuits is equal to Qef (the effluent flow rate = V) × SC (UF/P) for most small and middle molecules and is directly proportional to the amount of effluent volume. Blood-side clearances often do not match the filter clearances, as membrane adsorption modifies the amount of solute in the ultrafiltrate. Consequently, for some solutes [such as tumor necrosis factor-α (TNF-α)] that are adsorbed by membranes, SC can be low, but overall blood clearance can be greater than filter clearance.
CRRT vary in their ability to remove small and middle molecules. For small-sized solutes (e.g., urea nitrogen, creatinine, phosphates), filter clearances were directly proportional to the effluent volume and did not vary significantly with convective or diffusive removal across a spectrum of effluent volumes (0.5 to 4.5 L/h). In contrast, β2-microglobulin removal was influenced by the membrane type and the amount of convective clearance (102).
Clearance of molecules in CRRT circuits also depends on the site of replacement solution administration either pre- or postfilter.
In postdilution CVVH (purely convective therapy), primary determinants of solute clearances are ultrafiltration rate (Qef), sieving coefficient of membrane (S). Therefore, clearance in milliliters per minute (K) equals to product of ultrafiltration rate and sieving coefficient (EQUATION 10.4).

For small molecules, as S approaches unity, clearance equals the UF rate in postdilution.
Removal of ultrafiltrate across the filter concentrates the cellular elements and proteins in the blood emerging from the filter and is directly proportional to the ratio of ultrafiltrate to plasma flow rate (filtration fraction = FF). Plasma flow rate in turn depends upon blood flow (Qb) and patient hematocrit (Hct). Therefore, in postdilution CVVH (EQUATION 10.5):

Previous studies have demonstrated that FF >20% contributes to reduced filter performance and filter clotting. Consequently, if UF rates are increased, the blood-flow rate should be increased to maintain FF less than 20%. As higher blood flows are usually difficult to reach with the temporary dialysis catheters and poor hemodynamics among these patients, achieving higher doses are difficult to do in postdilution mode.
Predilution fluid replacement reduces the FF and reduces the solute concentration in the blood entering the filter. Thus, it is useful in preventing clotting of the extracorporeal circuit and to extend filter life. The effective small-solute clearance (K) for predilutional H is equal to Qef × (Qb/[Qb + Qr]), where Qb and Qr represent blood and replacement fluid rates. Clearance in predilution H is less than in postdilutional H for the same Qef. However, because of the dilution of blood entering the filter, much higher filtration fractions (larger Qef and Qr) are feasible in predilutional H (103).
In convective removal, the only way to increase solute clearances is to increase the amount of ultrafiltrate generated and consequently increase the volume of replacement fluid given. A study by Brunet et al. (104) showed that convection is effective than diffusion in removing middle molecular weight solutes during CRRT and that high convective fluxes should be applied if the goal is to remove middle molecules more efficiently.
CONTINUOUS RENAL REPLACEMENT THERAPY—APPLICATIONS
Fluid Management in Continuous Renal Replacement Therapy
Volume management is an integral component of the care of critically ill patients to maintain hemodynamic stability and optimize organ function. The dynamic nature of critical illness often necessitates volume resuscitation and contributes to fluid overload particularly in the presence of altered renal function. Diuretics are commonly used as an initial therapy to increase urine output; however, they have limited effectiveness due to underlying AKI and other factors contributing to diuretic resistance. In this setting, successful volume management with CRRT depends on an accurate assessment of fluid status, an adequate comprehension of the principles of fluid management with UF, and clear treatment goals. In the Beginning and Ending Supportive Therapy (BEST) for the Kidney study (105), fluid overload was present in 36.7%, while oliguria and anuria was present in 70.2% of patients. Fluid overload increases complications associated with acute lung injury, acute respiratory distress syndrome (106–108), tissue healing, gut, and cerebral edema (109). Emerging data overwhelmingly suggest that fluid overload is associated with adverse outcomes (110–112).
CRRT techniques have three inherent characteristics that make them highly effective and versatile methods for fluid control (113) (a) the use of highly permeable membranes, (b) the infusion of various replacement solutions, and (c) the continuous nature of the techniques. Adjustments in the UF and replacement fluid rates allow CRRT techniques to serve as fluid regulatory systems that can maintain fluid balance without compromising the system’s ability to maintain metabolic balance. A major distinction for these methods is the ability to dissociate solute removal (e.g., sodium) from fluid balance. As an example, by varying the composition of the replacement fluid or dialysate, solute balance can be altered while overall fluid balance can be kept even, negative, or positive (113). Fluid removal in CRRT is achieved by formation of an ultrafiltrate. The UF rate used depends on two factors: the type of technique and the fluid balance requirements of the patient. In convective techniques (CVVH), the UF rate can vary from 0.5 to 12.0 L/h, although most centers use a range of 1.0 to 3.0 L/h. When dialysate is used (CVVHDF), almost all the current machines limit UF rate to a maximum of 2 L/h.
Bouchard and Mehta (114) describe three techniques for achieving fluid balance with CRRT (TABLE 10.5). The level 1 technique is to vary the net ultrafiltration rate (Quf) to meet the anticipated fluid balance needs over 8 to 24 hours; the net ultrafiltrate is the difference between the total ultrafiltrate (the plasma water removed) and the total replacement (the fluid given to the patient). As an example, a patient may have a total anticipated fluid intake of 3 L with a desired 1-L net loss over 24 hours; the Quf would be set at −170 mL/h (3 L + 1 L/24 h). This may not be the best technique to use, as there may be unanticipated changes in clinical status and fluid needs, leading to a different net ultrafiltrate that is different from the desired fluid balance. Also, effluent volume and treatment dose will vary, as net Quf are not steady with this method.
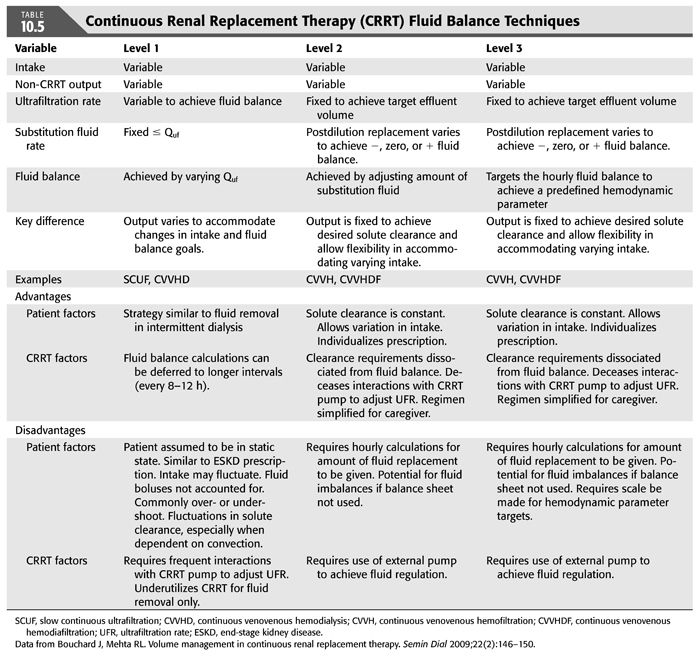
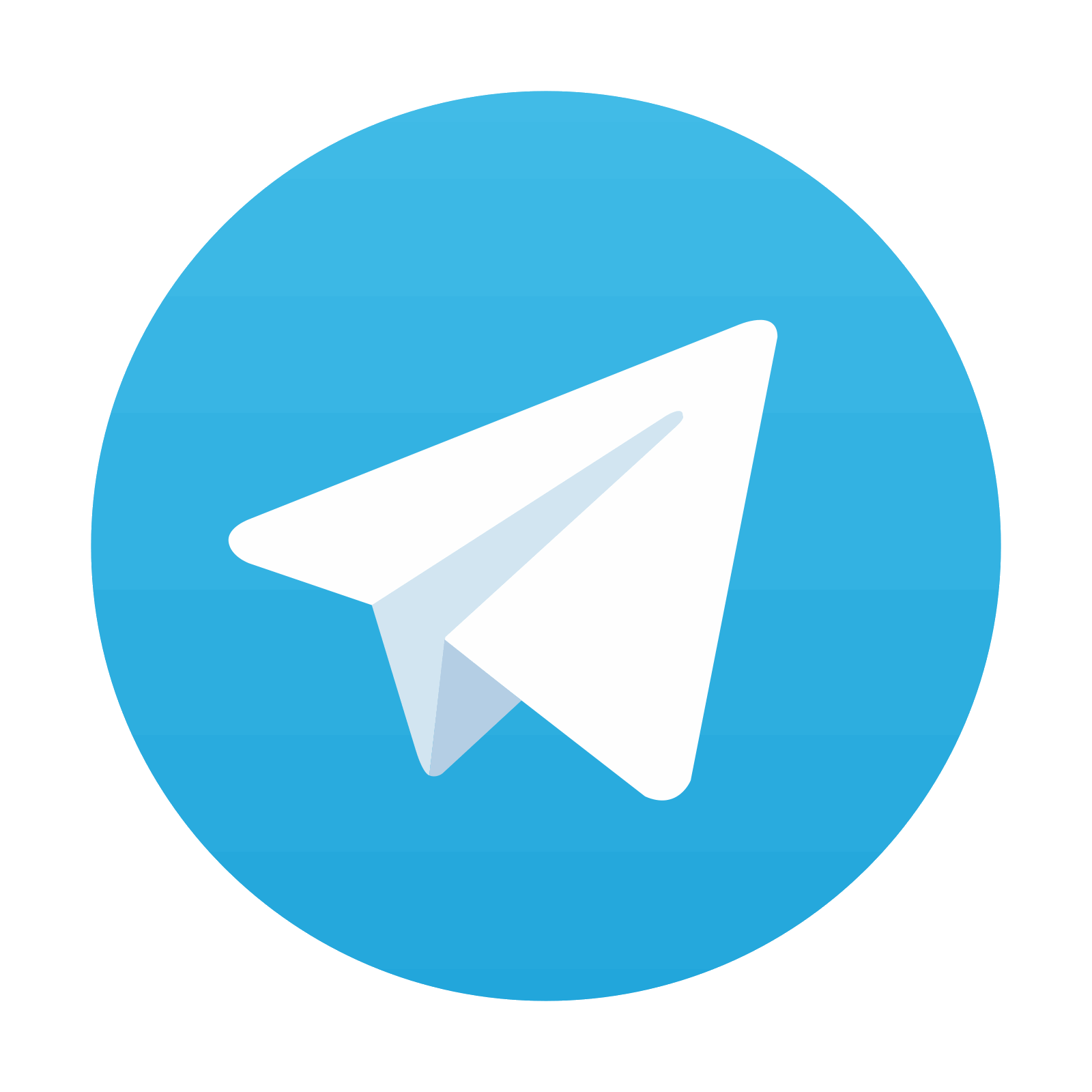
Stay updated, free articles. Join our Telegram channel
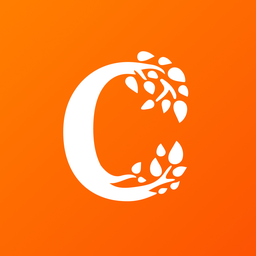
Full access? Get Clinical Tree
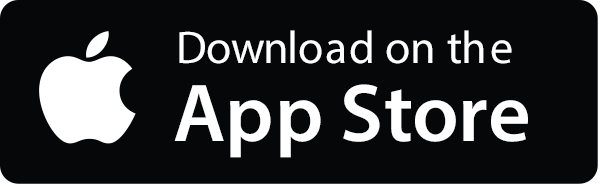
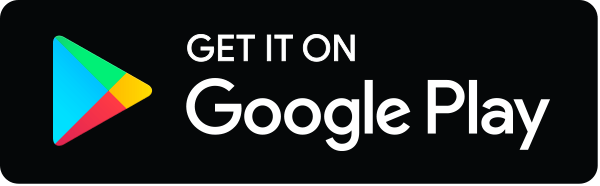